- 1Department of Geological Sciences, University of Oregon, Eugene, OR, USA
- 2Baker City Field Office, Oregon Department of Geology and Mineral Industries, Baker City, OR, USA
- 3Department of Earth and Atmospheric Sciences, University of Alberta, Edmonton, AB, Canada
- 4School of the Environment, Washington State University, Pullman, WA, USA
We present new isotopic and trace element data for four eruptive centers in Oregon: Wildcat Mountain (40 Ma), Crooked River (32–28 Ma), Tower Mountain (32 Ma), and Mohawk River (32 Ma). The first three calderas are located too far east to be sourced through renewed subduction of the Farallon slab following accretion of the Yellowstone-produced Siletzia terrane at ~50 Ma. Basalts of the three eastern eruptive centers yield high Nb/Yb and Th/Yb ratios, indicating an enriched sublithospheric mantle source, while Mohawk River yields trace element and isotopic (δ18O and εHf) values that correlate with its location above a subduction zone. The voluminous rhyolitic tuffs and lavas of Crooked River (41 × 27 km) have δ18Ozircon values that include seven low δ18Ozircon units (1.8–4.5‰), one high δ18Ozircon unit (7.4–8.8‰), and two units with heterogeneous zircons (2.0–9.0‰), similar to younger Yellowstone-Snake River Plain rhyolites. In order to produce these low δ18O values, a large heat source, widespread hydrothermal circulation, and repeated remelting are all required. In contrast, Wildcat Mountain and Tower Mountain rocks yield high δ18Ozircon values (6.4–7.9‰) and normal to low εHfi values (5.2–12.6), indicating crustal melting of high-δ18O supracrustal rocks. We propose that these calderas were produced by the first appearance of the Yellowstone plume east of the Cascadia subduction zone, which is supported by plate reconstructions that put the Yellowstone plume under Crooked River at 32–28 Ma. Given the eastern location of these calderas along the suture of the accreted Siletzia terrane and North America, we suggest that the Yellowstone hotspot is directly responsible for magmatism at Crooked River, and for plume-assisted delamination of portions of the edge of the Blue Mountains that produced the Tower Mountain magmas, while the older Wildcat Mountain magmas are related to suture zone instabilities that were created following accretion of the Siletzia terrane.
Introduction
Trace element and isotopic data of magmatic rocks have long been used to relate magma petrogenesis to geotectonic settings (e.g., Auer et al., 2008; Jicha et al., 2009; Seligman et al., 2014). We use these methods to investigate three large 30–40 Ma calderas in eastern Oregon that were recently identified and have an unknown geotectonic origin (McClaughry et al., 2009b) (Figure 1). Despite nearly 40 m.y. of erosion, these calderas preserve volcano-tectonic depressions with respective rings of hydrothermally altered post-caldera rhyolite intrusions, thick intracaldera tuffs, and central resurgent and ring-fracture rhyolite domes. The rocks that form these three paleontologically important calderas were originally mapped as part of the John Day and Clarno formations, signifying a correlation and likely source. These three eastern Oregon calderas are all located near the Klamath-Blue Mountain gravity-anomaly lineament (Figure 1), which marks the boundary between the Blue Mountains Province and the accreted Siletzia terrane, and were all erupted through the Paleozoic Blue Mountains Province (Figure 1). Limestone is locally present in the accreted terranes underlying the calderas and is present as xenoliths in multiple tuffs. Other calderas and caldera-forming tuffs that we studied for comparison belong to the volcanic front of the ancestral Cascades.
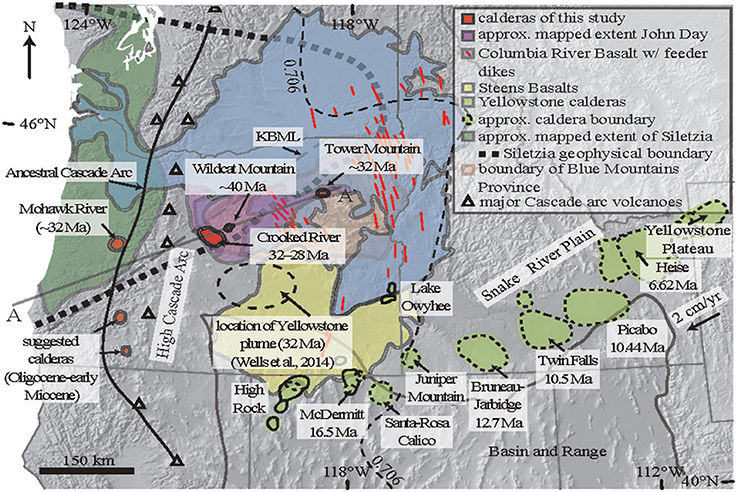
Figure 1. Map showing the location of the newly identified Crooked River, Wildcat Mountain, Tower Mountain, and Mohawk River calderas (McClaughry et al., 2009a,b, 2010; age for Tower Mountain caldera is from Martin Streck, personal communication) in relation to other key features of the Pacific Northwest. Location of the 32 Ma Yellowstone plume is derived from G-Plates (Seton et al., 2012; Wells et al., 2014; see the Supplementary Material for Movie and sources for the map features and ages). Accounting for rotation of Oregon (Wells et al., 1998) causes the locations of the Crooked River caldera and the reconstructed Yellowstone plume to coincide, as shown in the Supplementary Material. A–A' transect is shown in cross section in Figure 7.
Around 50 Ma, subduction of the nearly horizontal Farallon slab was halted by accretion of the Large Igneous Province known as Siletzia (56–49 Ma) from western Oregon to southwestern British Columbia (Atwater and Stock, 1998; Wells et al., 2014). Accretion caused (1) dismemberment of the subducting Farallon slab, (2) subduction to migrate westward and reinitiate along the western margin of Siletzia, and (3) stagnation of the nearly horizontal limb of the Farallon slab beneath Oregon, where geophysical data suggest the slab remnant is presently lodged (Gao et al., 2011; Darold and Humphreys, 2013). Previous work by Duncan (1982) and Wells et al. (1984) suggested that the long-lived Yellowstone plume powered magmatism responsible for development of the Siletzia Large Igneous Province oceanward from the Pacific Northwest coast. However, the proposed scenario results in an unusually large ~30 m.y. gap in recognized Yellowstone plume-related eruptions between 56 and 49 Ma Siletzia magmatism (Wells et al., 2014) and eruption of the Columbia River basalts at ~17 Ma in eastern Oregon and coeval calderas in northern Nevada (Coble and Mahood, 2012; Ferns and McClaughry, 2013).
Renewed Cascadia subduction and related arc magmatism built a north-south Cascade volcanic front, initiating in southern Washington and northern Oregon, with the first ancestral Cascade volcanoes and calderas appearing around 42 Ma (du Bray and John, 2011). East of the ancestral Eocene-Oligocene Cascade arc, voluminous 30–40 Ma ash-flow tuffs associated with large caldera forming eruptions were deposited as part of the Clarno and John Day formations. However, the causative tectonic and magmatic origins of these calderas and their correspondent ash-flow tuffs remain enigmatic (McClaughry et al., 2009b).
The present study aims at determining how the voluminous silicic magmas in these newly identified calderas were formed, assuming that each site of abundant silicic magmatism requires large quantities of basaltic heat and mass fluxes from the mantle. Herein, we define processes that may foster genesis of large-scale, within-plate volcanism. In particular, we explore whether the Yellowstone plume could have been somehow responsible for the genesis of these large-volume centers of volcanism, and if so, how it can be reconciled with the ongoing subduction of the Farallon slab under North America. We use major and trace element geochemistry, new U-Pb geochronologic data, and in situ O and Hf isotopic investigations of zircons for three recently identified calderas, whose rocks are part of the John Day and Clarno formations (Figure 1) (McClaughry et al., 2009b): Crooked River, Tower Mountain, and Wildcat Mountain. We then compare their isotopic and trace element characteristics with those of contemporaneous calderas known to be part of the Cascade arc: the large 25-km diameter Mohawk River caldera (McClaughry et al., 2010) and several other regionally abundant 40–25 Ma tuff layers (Figure 1), known to be part of the ancestral Cascade arc. Our trace element and isotopic data thus place constraints on crustal and mantle processes that previously have been investigated from a geodynamic and geophysical perspective.
Materials and Methods
In this study we apply single crystal and in situ methods for determining the primary magmatic values for these rocks that have been heavily altered. Intense hydrothermal alteration has caused many of the minerals, such as feldspar, to break down to clays, and for much of the quartz to be secondarily reprecipitated. Any quartz or feldspar analyzed from the Crooked River caldera was pretreated in HF to remove any outer rind of alteration and checked for melt inclusions to be sure these are primary minerals. Furthermore, when reducing the data, we trust the lowest feldspar and quartz δ18O values, since higher values are typically indicative of secondary effects due to higher Δ18Omin-H2O values. In addition, we primarily focus on analyses of alteration-resistant zircon when studying rocks from the Crooked River caldera. δ18O compositions of 1–2 mg of quartz, plagioclase, pyroxene, olivine, amphibole, and bulk zircon phenocrysts were determined by laser-fluorination in the stable isotope laboratory at the University of Oregon (e.g., Bindeman, 2008). δ18O compositions of mounted and imaged zircon crystals were further refined in their δ18O values by targeting cores and rims in situ using the Cameca 1280 ion microprobe at the University of Alberta (±0.16‰2σ). The Hf isotopic composition of zircon was then determined for some of these same spots at Washington State University's Radiogenic Isotope and Geochronology Lab (±0.8–2.0 εHf) (Fisher et al., 2014). Individual zircon cores and rims were then analyzed for 238U-206Pb ages using the CAMECA ims 1270 ion microprobe at UCLA. Analytical techniques are described in detail in the Supplementary Material, and details of these analyses can be found in Tables S1–S6. Selected XRF data were obtained at Pomona College and others at Washington State University (published), and basalt and basaltic andesite samples were analyzed for trace elements by ICP-MS at the Solid Earth Geochemistry Lab at Harvard to determine the geochemical signature of the source magmas.
Results
Petrography and Geochemistry of Rocks Associated with the Large Oregon Calderas
The Mohawk River caldera currently has two main units associated with it (the Tuff of Mohawk River and the basalt of Mt. Tom). The location of the Mohawk River caldera, within the ancestral Cascade volcanic arc domain, implies a subduction-related petrogenesis (Figure 1). Furthermore, the presence of abundant (10–20%) phenocrysts in the tuff of Mohawk River and its calc-alkaline geochemistry (low Nb and Zr), which are also characteristics of other major coeval tuffs of the ancestral Cascade arc that we studied (du Bray and John, 2011), suggest a subduction-type source and derivation by fractionation of a cool and wet basaltic magma (Figures 1, 2B). Other studied tuffs (Dexter, Fox Hollow, and Bond Creek) have similar mineralogical characteristics.
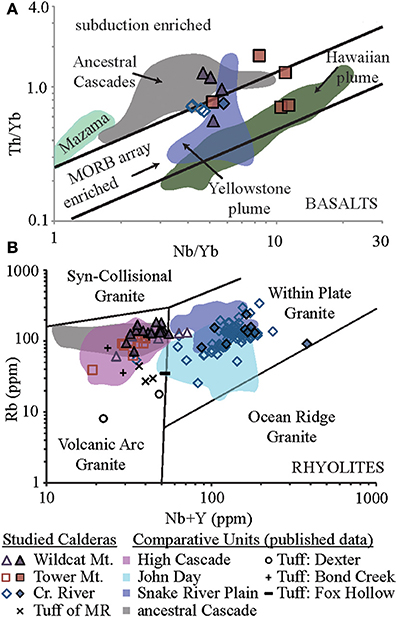
Figure 2. Trace element data for rocks from the studied calderas and other nearby Cenozoic volcanic rocks (see the Supplementary Material). (A) Discrimination diagram showing trace element ratios for pre- and post- caldera basalts associated with the three eastern Oregon calderas; data field boundaries from Pearce and Peate (1995). All of the basalts have elevated Nb/Yb and Th/Yb relative to N-MORB. For caldera data, closed symbols are from this study and open symbols represent compiled published data from multiple sources (see Supplementary Material for References). (B) Trace element discrimination diagram of Pearce et al. (1984) showing the relative abundances of Rb vs. Nb+Y in rocks from the four studied calderas vs. those of other nearby Cenozoic volcanic rocks, including specific tuffs of the ancestral Cascades (Dexter, Bond Creek, and Fox Hollow). The distinct overlap in the compositions of the Snake River Plain rocks of the Yellowstone hotspot track and those of the Crooked River rhyolites suggests similar petrogenetic histories.
Rocks analyzed from the Tower Mountain and Wildcat Mountain calderas include their major caldera forming tuffs (the tuff of Steins Pillar from the Wildcat Mountain caldera and the tuff of Dale from the Tower Mountain caldera), as well as pre- and post-caldera domes and lavas that range continuously from basalt to rhyolite. Rocks associated with these calderas include hydrous minerals (amphibole ± biotite). The presence of hydrous minerals and the continuous range in magma compositions is similar to many rocks found in subduction-type settings. However, their locations far behind the already well-defined ancestral Cascade arc (du Bray and John, 2011) indicate an intraplate origin. The rhyolites of the Tower Mountain and Wildcat Mountain calderas have Nb+Y abundances that overlap with those of the high and ancestral Cascade rhyolites (Figure 2B). In contrast, the correlated basalts have high field strength element (HFSE) abundances and Nb/Yb and Th/Yb ratios that are elevated relative to N-MORB (Figure 2A and Supplementary Material) and the modern (e.g., Mazama) and ancestral Cascades (Bacon, 1989; Bacon et al., 1997; du Bray and John, 2011). These relations are consistent with a deep, undepleted, sublithospheric mantle origin (Pearce and Peate, 1995).
In contrast, rocks associated with the Crooked River caldera are nearly aphyric, containing sparse quartz and feldspar, compositionally bimodal (basalt and rhyolite), and do not contain hydrous minerals. These characteristics are consistent with a dry, high temperature, crystal poor magma. Rocks analyzed from the Crooked River caldera include multiple caldera-forming tuffs, ring-fracture rhyolites, and basaltic lavas. These types of rocks are similar to many rhyolites of the Yellowstone-Snake River Plain (Nash et al., 2006; Christiansen and McCurry, 2008; McCurry and Rodgers, 2009; Watts et al., 2011). The Crooked River and correlative John Day Formation rhyolites also have distinctly elevated Nb+Y concentrations (Figure 2B). Similar to the basalts of the Tower Mountain and Wildcat Mountain calderas, the basalts associated with the Crooked River eruptive center also have elevated HFSE and Nb/Yb and Th/Yb ratios relative to N-MORB (Figure 2A and Supplementary Material). These data are again consistent with a deep, undepleted, sublithospheric mantle origin.
U-Pb Dating of Zircons and Eruptive Histories of the Oregon Calderas
In an attempt to better constrain eruptive order, we determined U-Pb ages of zircons from three units of the Crooked River caldera. Although the ages of these three units correlate with known stratigraphic positions, their errors are unusually large, and we therefore report two possible ages for each unit (Tables S4, S5 in the Supplementary Material). For all other units, we rely on previously determined 40Ar/39Ar, K/Ar, and U-Pb ages, as well as stratigraphic constraints, which are all listed in Table S1 in the Supplementary Material.
δ18O and εHfi Compositions: A Case for Crustal Remelting
δ18O and εHfi compositions of zircon in the rhyolites of the four calderas were used as proxies for magmatic values and thus help distinguish magmatic processes that contributed to the petrogenesis of each of the four studied calderas. εHfi in the individual zircons (+5.2 to +12.6) is lower than that of depleted mantle, as expected of young magma sourced from non-depleted mantle. There is an overall similarity of relatively high εHfi values across the four calderas located west of the 87Sr/86Sr = 0.706 line. The lowest εHfi values (+5.2, +5.3) are from a single zircon in the tuff of Dale from the easternmost Tower Mountain caldera, which suggests the influence of an older (lower εHfi) crustal source such as pre-Mesozoic sedimentary deposits of North America (Figure 6).
Measured δ18Ozircon values are both lower and higher than normal mantle δ18Ozircon values (+5.0–5.6‰; Valley et al., 2005). Magmas that crystallize δ18Ozircon >5.6‰ generally necessitate assimilation of high δ18O rocks (e.g., older supracrustal rocks), which is seen in rocks erupted from the Crooked River, Tower Mountain, and Wildcat Mountain calderas, whereas δ18Ozircon <5.0‰ typically requires assimilation of material that was previously hydrothermally altered by low δ18O meteoric water (Crooked River caldera—see below for further details) (e.g., Watts et al., 2011). δ18O of zircons and quartz from the Mohawk River caldera in the ancestral Cascades arc of western Oregon (Figure 1) have εHfi that ranges from +8.6 to +12.4, and normal to moderately low δ18Ozircon (~+5.0‰), which are in equilibrium with quartz (δ18O = +6.9–7.7‰). These normal δ18O values are similar to other major coeval tuffs studied here from the ancestral Cascades (Figures 3–5), and also from arc rocks worldwide (Johnson et al., 2009; Bindeman et al., 2010; Martin et al., 2011). Conversely, rocks of the Wildcat Mountain and Tower Mountain calderas have elevated (+6.2–7.9‰) zircon δ18O values that are in equilibrium with other analyzed phenocrysts (plagioclase, quartz, and a few amphiboles) and subsequently show true magmatic isotopic fractionations (Figures 3, 4). High δ18O values require melting of high δ18O rocks, such as supracrustal sediments and limestone, present in the surrounding Paleozoic Blue Mountains Province and xenoliths in most tuffs, which we determined to have a carbonate δ18O value of +24.7‰. Alternatively, high δ18O values could be coming from the underlying Siletzia terrane pillow lavas and high δ18O sediments on top of Siletzia.
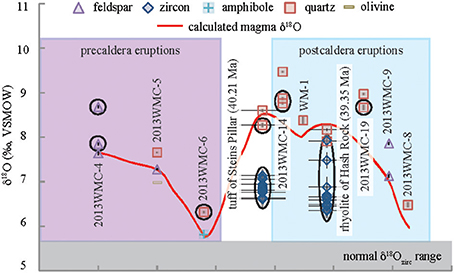
Figure 3. δ18O vs. age for zircon, feldspar, quartz, olivine, and amphibole for rocks associated with the Wildcat Mountain caldera (References for ages are listed in the Supplementary Material). The vertical and horizontal bars through the symbols are 2σ error for the age (if applicable) and δ18O analysis, respectively. A circle around the analysis indicates single grain analysis. The units analyzed are split into pre- and post-caldera subsets due to the lack of known relative ages for all units except two. The calculated magma δ18O curve is based on the fractionation between average zircon (1.8‰), quartz (−1‰), or feldspar (~0‰) and the magma. The normal mantle δ18Ozircon range (5.0–5.6‰) is from Valley et al. (2005).
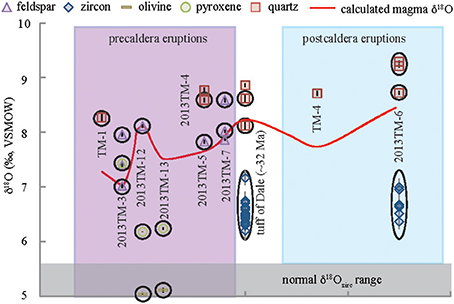
Figure 4. δ18O vs. age for zircon, feldspar, quartz, olivine, and pyroxene for rocks associated with the Tower Mountain caldera. The age for the tuff of Dale is based on personal communication by Martin Streck. See Figure 3 for other symbols and explanations.
Zircons (cores and rims) in nine rhyolite units associated with the Crooked River caldera have a mixture of homogenous low and high δ18O values, and heterogeneous δ18O values within each sample (Figure 5). Only zircon and scarce quartz and feldspar phenocrysts preserve magmatic δ18O values, because nearly all phenocrysts in the Crooked River rocks are altered. These low δ18O units (+1.8–4.5‰) include the major caldera-forming tuff of Smith Rock (δ18Ozircon = +2.6‰; ~29 Ma), the tuff of Eagle Rock (δ18Ozircon = +4.4‰; 29.7 Ma), and four ring fracture rhyolites (δ18Ozircon = +2.3, +2.4, +2.4, +4.5). In addition, using laser fluorination we obtained a bulk zircon value of +4.2‰ for the 28.65 Ma Picture Gorge Ignimbrite of the John Day Formation (Figure 5). The low δ18Ozircon value suggests it was likely sourced from the Crooked River caldera. These low δ18Ozircon values of successive caldera-forming ignimbrites and post-caldera lavas indicate that Crooked River is a voluminous low δ18O province. The earlier erupted tuffs, however, have high δ18O values: Antelope Creek (δ18Ozircon +7.4–8.8‰; ~29.6 Ma) and the Tuff of Rodman Spring (δ18Oquartz +9.1–10.7‰; 32.5 Ma), while post-Picture Gorge ignimbrite eruptions exhibit heterogeneous δ18Ozircon populations: tuff of Barnes Butte (+2.3–8.7‰; 28.3 Ma), and the ring-fracture rhyolite of Ochoco Reservoir (+2.0–9.0‰; 27.54 Ma). Large-scale remelting of previously erupted, initially high δ18O tuffs and lavas, which were hydrothermally altered is required to produce so many low δ18O units. These processes are similar to those that are considered responsible for low δ18O magmatism associated with the vast majority of the Yellowstone-Snake River Plain calderas (e.g., Bindeman and Simakin, 2014) (Figure 1).
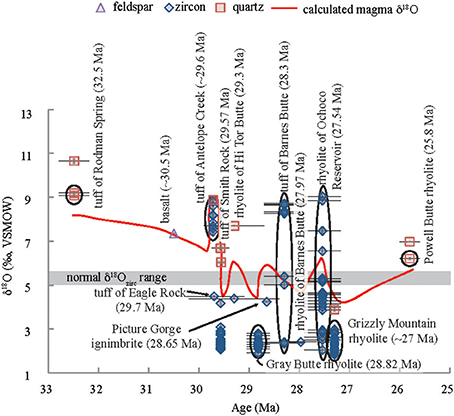
Figure 5. δ18O vs. age for zircon, feldspar, and quartz associated with the Crooked River caldera. Ages are from this work and previous studies (listed in the Supplementary Material). The low δ18Ozircon values of the Crooked River caldera are contrasted by the high δ18Ozircon values of the Tower Mountain (Figure 4) and Wildcat Mountain (Figure 3) calderas. See Figure 3 for other symbols and explanations.
The δ18O values in all three central and eastern Oregon calderas signify large degrees of crustal melting of both high δ18O basement and low δ18O hydrothermally altered rocks. Since the zircons were extracted from rhyolites that were formed through crustal melting, the lower than depleted mantle εHfi values also indicate the influence of basement rocks that originated from sublithospheric mantle. The similarity of the εHfi values across the three calderas therefore signifies a similar source, such as the surrounding Paleozoic Blue Mountains Province (Figure 6). Therefore, the difference in δ18O values between the Wildcat and Tower Mountain calderas and the Crooked River caldera is not due to the difference in what is being melted, but is due to the degree of hydrothermal alteration. In other words, the elevated δ18O values of the Wildcat Mountain and Tower Mountain calderas define regional high δ18O levels, from which the low δ18O Crooked River magmas were derived after hydrothermal alteration.
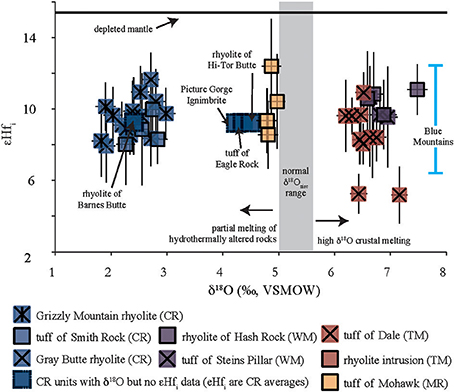
Figure 6. δ18O vs. εHfi data for zircons of the studied calderas. εHfi values for the Picture Gorge Ignimbrite, the rhyolite of Hi-Tor Butte, the Tuff of Barnes Buttes, and the Tuff of Eagle Rock are average values for the Crooked River caldera, since they were analyzed for δ18O and not εHf, and are symbolized by a blue square with a dashed border. εHfi depleted mantle value is from Nowell et al. (1998). The range in εHfi values of zircons from the Blue Mountains Province is from Schwartz et al. (2011).
Discussion
Low δ18O Rhyolites Associated with the Yellowstone Hotspot
Eruptive centers associated with the Yellowstone plume have produced some of the world's most voluminous low δ18O magmas. Low δ18O magmas are associated with nearly all currently identified Yellowstone-plume related calderas, which have an aggregated low δ18O eruption volume >10,000 km3 (Boroughs et al., 2005; Cathey et al., 2011; Watts et al., 2011; Drew et al., 2013). Although plume magmas do not initially have lighter oxygen isotopic ratios, their larger heat source makes it possible, and more likely, to foster widespread hydrothermal circulation, alteration, and subsequent remelting that yields low δ18O magmas (e.g., Bindeman and Simakin, 2014), which could be possible at eruptive centers such as Crooked River, as is further argued below. We therefore use low δ18O values in voluminous tuffs as an indicator of a need for an exceptionally large heat source to achieve repeated shallow crustal remelting.
Calderas of Oregon as Geodynamic Indicators
The location of the central-eastern Oregon calderas to the east of the ancestral Cascade volcanic arc, which was already developed prior to formation of these calderas (du Bray and John, 2011) (Figure 1), suggests these magmas are related to a within-plate tectonic process. Another significant trend involving their location is represented by their location along the suture between the Siletzia terrane and the terranes underlying the Blue Mountains Province (Figure 1). If these calderas were associated with flat slab subduction, a wider swath of ancestral Cascade arc volcanoes across Oregon should be (but is not) present. Instead, the ancestral Cascades reside to the west of the high Cascades (du Bray and John, 2011), which are still located to the west of the three eastern Oregon volcanoes of this study (Figure 1). If these magmas were formed through back-arc spreading, then an arc-parallel or rift-parallel arrangement of volcanic vents is expected, which is seen in back arc volcanism such as Kamchatka (Münker et al., 2004) or in rifting environments such as eastern Africa (Chorowicz, 2005). If these calderas were a northwestern extension of the “ignimbrite flare-up” of the Great Basin of the southwest United States (Coney, 1978) one would expect to see a time transgressive series of eruptions due to the proposed “peeling off” of the underlying Farallon slab from the base of the North American crust (Humphreys, 1995), which is also not observed (Figure 1). This hypothesis is also contrasted by seismic imaging by Gao et al. (2011) and Darold and Humphreys (2013) who imaged the Farallon slab beneath this region of Oregon, signifying that there has been no wholesale peeling off of the Farallon slab in this area. Therefore, a different tectonic process is needed, which incorporates the location of these calderas to the east of the ancestral Cascades arc and along the suture between the Siletzia terrane and the terranes underlying the Blue Mountains, which is likely a region of geodynamic instability (Gorczyk et al., 2012).
A Case for Yellowstone Plume Assisted Delamination and the Earliest Appearance of Caldera-Forming Volcanism of the Yellowstone Plume
Recent work by Wells et al. (2014) shows that the Yellowstone plume was under central Oregon by ~35 Ma, and more specifically under the Crooked River caldera 32–28 Ma (see Movie in Supplementary Material). Therefore, based on the location of the Yellowstone plume, and the location and geochemistry of the two ~30 Ma eastern Oregon calderas, we propose that their magmas are formed through interactions between the Yellowstone plume and delamination of the overlying crust near a region of geodynamic instability. The older 40 Ma Wildcat Mountain caldera likely has a different instability-based origin, which will be discussed below.
Current regional geodynamic models document complex interactions between the subducting Farallon slab and the Yellowstone plume (e.g., Johnston and Thorkelson, 2000; Murphy et al., 2003; Obrebski et al., 2010; Liu and Stegman, 2012). Models suggest that the plume could have five different options for how it interacted with the crust: (1) migrating through a gap in the Farallon slab; (2) migrating around the subducting slab, perhaps in bifurcating fashion; (3) melting through the slab; (4) ponding under the slab but allowing decompression basaltic partial melts to penetrate through it; or finally (5) ponding under the slab and causing the slab to buoyantly rise and restrict volcanism on top. The Yellowstone plume could have used any of the first four of these scenarios to migrate east of the Farallon slab following the formation of the Siletzia terrane.
In terms of plume-assisted delamination, numerical modeling by Burov et al. (2007) suggests that Rayleigh–Taylor instabilities of the lower crust occur within a few million years of the arrival of a plume at the base of the lithosphere. Camp and Hanan (2008) utilize plume-assisted delamination to explain the formation of the Columbia River Basalts. Plume-assisted delamination proceeds with: (1) creating cracks in the overlying crust through dikes and sills and (2) lowering the density and viscosity (1020 Pas; Steinberger and O'Connell, 1998) of the underlying mantle, which may in turn accelerate Stoke's sinking velocity, giving it sufficient time for devolatilization-melting of the surrounding mantle. Furthermore, non-plume-assisted delamination can still occur within ~5 m.y. based on numerical modeling, depending on the density of the lower crust and the viscosity of the mantle (e.g., Elkins-Tanton and Hager, 2000; Elkins-Tanton, 2005). Non-plume-assisted delamination may explain the magma formation of the 40 Ma Wildcat Mountain caldera.
More specifically, we propose that the magmas of the Tower Mountain and Wildcat Mountain calderas were produced through delamination and devolatilization of portions of the underlying terranes of the Blue Mountains (Figure 7). Delamination was likely caused through one of two processes. The first possibility is that the docking of the Siletzia terrane between 51 and 49 Ma could have resulted in instabilities at its boundary with the Blue Mountains Province. Localized instabilites could have caused the first episode of delamination, producing the magmas of the Wildcat Mountain caldera, which formed ~40 Ma. The delamination event that formed the magmas of the Tower Mountain caldera, which erupted ~8 m.y. later, was likely caused by plume-assisted delamination, based on the longer period of time between the docking of the Siletzia terrane and the eastern migration of the Yellowstone plume. These hypotheses are supported by our new geochemical data. The mantle lithosphere beneath eastern Oregon was previously hydrated and modified by tens of millions of years of flat subduction prior to accretion of the Siletzia terrane (Atwater and Stock, 1998). If a portion of the terranes underlying the Blue Mountains were delaminated underneath the Wildcat Mountain and Tower Mountain calderas, previous hydration and modification would allow for subsequent devolatilization following delamination. Delamination would further allow magma to be produced from a deeper, non-depleted region of the mantle, as is supported by the trace elemental signature of basalts studied in this region (Figure 2A). Delamination also allows the magmas to be produced from a cooler, wetter mantle, subsequently producing phenocrystic rocks that are rich in hydrous minerals, which is characteristic of the rocks erupted from the Wildcat and Tower Mountain calderas.
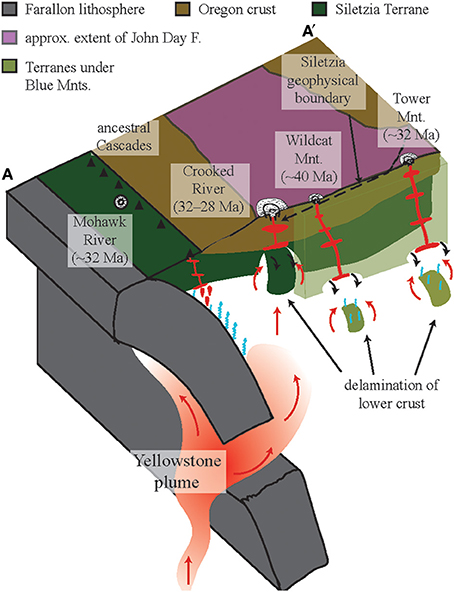
Figure 7. Schematic diagram illustrating the magmatic/tectonic setting that prevailed during formation of the four newly identified calderas. Small-scale delamination, devolatilization, and hydrous mantle melting of delaminated terranes underlying the Blue Mountains along the suture with the Siletzia terrane results in the magmas erupted from the Tower Mountain and Wildcat Mountain calderas. The largest episode of delamination (of the Siletzia terrane) produced the magmas of the Crooked River (super) caldera, aided by encroachment of Yellowstone plume. The location and compositional characteristics of the Mohawk River caldera (Figures 1, 2) are consistent with subduction-related arc magmatism. The location of the A–A′ transect is shown in map view in Figure 1.
In contrast, we propose that the Crooked River magmas are sourced directly from the Yellowstone plume. The formation of these magmas may also involve delamination, but this time of the Siletzia terrane, due to the close proximity between Crooked River and the suture between the Siletzia terrane and the Blue Mountains Province (Figure 1). Evidence for the Yellowstone plume producing magmas of the Crooked River caldera include: (1) geodynamic reconstructions using G-Plates by Wells et al. (2014) placing the Yellowstone plume under the Crooked River caldera from 32 to 28 Ma, which is the period of major and repeated silicic ignimbrite eruptions there (Figure 1; Movie in Supplementary Material); (2) The nearly aphyric, “hot and dry” nature of rocks and mineral assemblages associated with the Crooked River caldera, similar to other eruptions of the Yellowstone plume (Nash et al., 2006; Christiansen and McCurry, 2008; McCurry and Rodgers, 2009; Watts et al., 2011), signifies the need for a large heat source; (3) The enriched-MORB geochemical signature of the basalts, signifying the need for a non-depleted mantle source, similar to the Yellowstone plume (Figure 2A); (4) The bimodal basalt-rhyolite character; and finally (5) The low δ18O oxygen isotopic signature of multiple major Crooked River ignimbrites and post-caldera lavas, and zircon δ18O diversity in some later units, similar to nearly all such magmas in the Yellowstone hotspot track. This amplifies the need for remelting and recycling of previously erupted and hydrothermally-altered ignimbrites being incorporated in the eruptive material and thus requires a large heat source under the eruptive center (e.g., Bindeman and Simakin, 2014). Hence, we propose that the Crooked River caldera represents the oldest low δ18O province of the Yellowstone plume. Although not directly related to geotectonics, it is the recycled, low δ18O nature of Crooked River ignimbrites and post-caldera lavas that provides the most compelling evidence for the earliest caldera-forming eruptions of the Yellowstone plume at ~32 Ma.
Conflict of Interest Statement
The authors declare that the research was conducted in the absence of any commercial or financial relationships that could be construed as a potential conflict of interest.
Acknowledgments
We thank Charles Langmuir for inductively coupled plasma-mass spectrometry, and Jade Star Lackey for some X-ray fluorescence analyses. We would also like to thank Gene Humphreys and Paul Wallace for their valuable discussions. We would also like to thank Martin Streck for his discussions that greatly improved this manuscript. We also thank Charles Knaack for his assistance in the Radiogenic Isotope and Geochronology Lab at Washington State University. Angela N. Seligman is permanently indebted to Dana Drew for her tireless assistance in the field. We would also like to thank Dylan Colón and David Zakharov for assistance with sample collection. We recognize both Mark Ferns and Carrie Gordon for pioneering mapping efforts and for invaluable discussions about Oregon calderas. We also recognize Karyn Patridge for her early research on the Crooked River caldera. Finally, we thank Jörn-Frederik Wotzlaw and Timothy Druitt for their thoughtful reviews that improved this manuscript, and Adelina Geyer for editorial handling. The ion microprobe facility at the University of California, Los Angeles utilized during this study is partially supported by a grant from the Instrumentation and Facilities Program, Division of Earth Sciences, National Science Foundation. This work was supported by the National Science Foundation [EAR/CAREER-844772], and a grant from the Evolving Earth Foundation.
Supplementary Material
The Supplementary Material for this article can be found online at: http://www.frontiersin.org/journal/10.3389/feart.2014.00034/abstract
References
Atwater, T., and Stock, J. (1998). Pacific-North America plate tectonics of the Neogene Southwestern Unites States: an update. Int. Geol. Rev. 40, 375–402.
Auer, S., Bindeman, I., Wallace, P., Ponomareva, V., and Portnyagin, M. (2008). The origin of hydrous, high-d18O voluminous volcanism: diverse oxygen isotope values and high magmatic water contents within the volcanic record of Klyuchevskoy volcano, Kamchatka, Russia. Contrib. Mineral. Petrol. 157, 209–230. doi: 10.1007/s00410-008-0330-0
Bacon, C. R. (1989). Calc-alkaline, shoshonitic, and primitive tholeiitic lavas from monogenetic volcanoes near Crater Lake, Oregon. J. Petrol. 31, 135–166.
Bacon, C. R., Bruggman, P. E., Christiansen, R. L., Clynne, M. A., Donnelly-Nolan, J. M., and Hildreth, W. (1997). Primitive magmas at five Cascade volcanic fields: melts from hot, heterogeneous sub-arc mantle. Can. Mineral. 35, 397–423.
Bindeman, I. N. (2008). Oxygen isotopes in mantle and crustal magmas as revealed by single crystal analysis. Rev. Mineral. Geochem. 69, 445–478. doi: 10.2138/rmg.2008.69.12
Bindeman, I. N., Lenov, V. L., Izbekov, P. E., Ponomareva, V. V., Watts, K. E., Shipley, N. K., et al. (2010). Large-volume silicic volcanism in Kamchatka: Ar-Ar and U-Pb ages, isotopic, and geochemical characteristic of major pre-Hoocene caldera-forming eruptions. J. Volcanol. Geotherm. Res. 189, 57–80. doi: 10.1016/j.jvolgeores.2009.10.009
Bindeman, I. N., and Simakin, A. G. (2014). Rhyolite-Hard to produce, but easy to recycle and sequester: integrating microgeochemical observations and numerical models. Geosphere 10, 930–957. doi: 10.1130/GES00969.1
Boroughs, S., Wolff, J., Bonnichsen, B., Godchaux, M., and Larson, P. (2005). Large-volume, low-δ18O rhyolites of the central Snake River Plain, Idaho, USA. Geology 33, 821–824. doi: 10.1130/G21723.1
Burov, E., Guillou-Frottier, L., D'Acremont, E., Le Pourhiet, L., and Cloetingh, S. (2007). Plume head-lithosphere interactions near intra-continental plate boundaries. Tectonophysics 434, 15–38. doi: 10.1016/j.tecto.2007.01.002
Camp, V. E., and Hanan, B. B. (2008). A plume-triggered delamination origin for the Columbia river basalt group. Geosphere 4, 480–495. doi: 10.1130/GES00175.1
Cathey, H., Nash, B., Seligman, A., Valley, J., Kita, N., Allen, C. M., et al. (2011). “Low δ18O from the Bruneau-Jarbidge eruptive center: a key to crustal anatexis along the track of the Yellowstone hotspot [abs],” in Eos - Transactions, American Geophysical Union 92 (San Francisco, CA).
Chorowicz, J. (2005). The East African rift system. J. Afr. Earth Sci. 43, 379–410. doi: 10.1016/j.jafrearsci.2005.07.019
Christiansen, E., and McCurry, M. (2008). Contrasting origins of Cenozoic silicic volcanic rocks from the western Cordillera of the United States. Bull. Volcanol. 70, 251–267. doi: 10.1007/s00445-007-0138-1
Coble, M. A., and Mahood, G. A. (2012). Initial impingement of the yellowstone plume located by widespread silicic volcanism contemporaneous with Columbia River flood basalts. Geology 40, 655–658. doi: 10.1130/G32692.1
Coney, P. J. (1978). “Mesozoic-Cenozoic Cordilleran plate tectonics,” in Cenozoic Tectonics and Regional Geophysics of the Western Cordillera, Vol. 15, eds R. B. Smith and G. P. Eaton (Boulder, CO: Geological Society of America Memoir), 33–50.
Darold, A., and Humphreys, E. (2013). Upper mantle seismic structure beneath the Pacific Northwest: a plume-triggered delamination origin of the Columbia River flood basalt eruptions. Earth Planet. Sci. Lett. 365, 232–242. doi: 10.1016/j.epsl.2013.01.024
Drew, D., Bindeman, I., Watts, K., Schmitt, A., Fu, B., and McCurry, M. (2013). Crustal-scale recycling in caldera complexes and rift zones along the Yellowstone hotspot track: O and Hf isotopic evidence in diverse zircons from voluminous rhyolites of the Picabo volcanic field, Idaho. Earth Planet. Sci. Lett. 381, 63–77. doi: 10.1016/j.epsl.2013.08.007
du Bray, E. A., and John, D. A. (2011). Petrologic, tectonic, and metallogenic evolution of the ancestral Cascades magmatic arc, Washington, Oregon, and northern California. Geosphere 7, 1102–1133. doi: 10.1130/GES00669.1
Duncan, R. A. (1982). A captured island chain in the coast range of Oregon and Washington. J. Geophys. Res. 87, 827–10837.
Elkins-Tanton, L. T. (2005). “Continental magmatism caused by lithospheric delamination,” in Geological Society of America Special Paper, Plates, plumes, and paradigms, Vol. 388, eds G. R. Foulger, J. H. Natland, D. C. Presnall, and D. L. Anderson, 449–461.
Elkins-Tanton, L. T., and Hager, B. H. (2000). Melt intrusion as a trigger for lithospheric foundering and the eruption of the Siberian flood basalts. Geophys. Res. Lett. 27, 2937–3940. doi: 10.1029/2000GL011751
Ferns, M. L., and McClaughry, J. D. (2013). “Stratigraphy and volcanic evolution of the middle Miocene to Pliocene La Grande-Owyhee eruptive axis in eastern Oregon,” in The Columbia River Flood Basalt Province. Geological Society of America Special Paper, Vol. 497, eds S. P. Reidel, V. E. Camp, M. E. Ross, J. A. Wolff, B. S. Martin, T. L. Tolan, et al., 401–427.
Fisher, C. M., Vervoort, J. D., and DuFrane, S. A. (2014). Accurate Hf isotope determinations of complex zircons using the “laser ablation split stream” method. Geochem. Geophys. Geosyst. 15, 1–19. doi: 10.1002/2013GC004962
Gao, H., Humphreys, E., Yao, H., and van der Hilst, R. (2011). Crust and lithosphere structure of the northwestern U.S. with ambient noise tomography: Terrane accretion and Cascade arc development. Earth Planet. Sci. Lett. 304, 202–211. doi: 10.1016/j.epsl.2011.01.033
Gorczyk, W., Hobbs, B., and Gerya, T. (2012). Initiation of Rayleigh-Taylor instabilities in intra-cratonic settings. Tectonophysics 514, 146–155. doi: 10.1016/j.tecto.2011.10.016
Humphreys, E. D. (1995). Post-Laramide removal of the Farallon slab, western United States. Geology 23, 987–990.
Jicha, B. R., Hart, G. L., Johnson, C. M., Hildreth, W., Beard, B. L., Shirey, S. B., et al. (2009). Isotopic and trace element constraints on the petrogenesis of lavas from the Mount Adams volcanic field, Washington. Contrib. Mineral. Petrol. 157, 189–207. doi: 10.1007/s00410-008-0329-6
Johnson, E. R., Wallace, P. J., Granados, H. D., Manea, V. C., Kent, A. J. R., Bindeman, I. N., et al. (2009). Subduction-related volatile recycling and magma generation beneath Central Mexico: insights from melt inclusions, oxygen isotopes and geodynamic modeling. J. Petrol. 50, 1729–1764. doi: 10.1093/petrology/egp051
Johnston, S. T., and Thorkelson, D. J. (2000). Continental flood basalts: episodic magmatism above long-lived hotspots. Earth Planet. Sci. Lett. 175, 247–256. doi: 10.1016/S0012-821X(99)00293-9
Liu, L., and Stegman, D. R. (2012). Origin of Columbia River flood basalt controlled by propagating rupture of the Farallon slab. Nature 482, 386–390. doi: 10.1038/nature10749
Pubmed Abstract | Pubmed Full Text | CrossRef Full Text | Google Scholar
Martin, E., Bindeman, I. N., and Grove, T. L. (2011). The origin of high-Mg magmas in Mt Shasta and Medicine Lake volcanoes, Cascade Arc (California): higher and lower than mantle oxygen isotope signatures attributed to current and past subduction. Contrib. Mineral. Petrol. 162, 945–960. doi: 10.1007/s00410-011-0633-4
McClaughry, J., Ferns, M. L., Gordon, C. L., and Patridge, K. A. (2009a). Field trip guide to the Oligocene Crooked River caldera: Central Oregon's supervolcano, Crook, Deschutes, and Jefferson Counties, Oregon. Oregon Geol. 69, 25–44.
McClaughry, J. D., Ferns, M. L., Streck, M. J., Patridge, K. A., and Gordon, C. L. (2009b). “Paleogene calderas of central and eastern Oregon: eruptive sources of widespread tuffs in the John Day and Clarno Formations,” in Volcanoes to Vineyards: Geologic Field Trips Through the Dynamic Landscape of the Pacific Northwest. Geological Society of America Field Guide, Vol. 15, eds J. E. O'Connor, R. J. Dorsey, and I. P. Madin, 407–434.
McClaughry, J. D., Wiley, T. J., Ferns, M. L., and Madin, I. P. (2010). Digital Geologic Map of the Southern Willamette Valley, Benton, Lane, Linn, Marion, and Polk Counties, Oregon. Oregon Department of Geology and Mineral Industries Open File Report 0-10-03.
McCurry, M., and Rodgers, D. W. (2009). Mass transfer along the Yellowstone hotspot track I: petrologic constraints on the volume of mantle-derived magma. J. Volcanol. Geotherm. Res. 188, 86–98. doi: 10.1016/j.jvolgeores.2009.04.001
Münker, C., Wörner, G., Yogodzinski, G., and Churikova, T. (2004). Behaviour of high field strength elements in subduction zones: constraints from Kamchatka-Aleutian arc lavas. Earth Planet. Sci. Lett. 224, 275–293. doi: 10.1016/j.epsl.2004.05.030
Murphy, J. B., Hynes, A., Johnston, S. R., and Keppie, J. D. (2003). Reconstructing the ancestral Yellowstone plume from accreted seamounts and its relationship to flat-slab subduction. Tectonophysics 365, 185–194. doi: 10.1016/S0040-1951(03)00022-2
Nash, B., Perkins, M., Christensen, J., Lee, D., and Halliday, A. (2006). The Yellowstone hotspot in space and time: Nd and Hf isotopes in silicic magmas. Earth Planet. Sci. Lett. 247, 143–156. doi: 10.1016/j.epsl.2006.04.030
Nowell, G. M., Kempton, P. D., Noble, S. R., Fitton, J. G., Saunders, A. D., Mahoney, J. J., et al. (1998). High precision Hf isotope measurements of MORB and OIB by thermal ionasation mass spectrometry: insights into the depleted mantle. Chem. Geol. 149, 211–233. doi: 10.1016/S0009-2541(98)00036-9
Obrebski, M., Allen, R. M., Xue, M., and Hung, S. (2010). Slab-plume interaction beneath the Pacific Northwest. Geophys. Res. Lett. 37, 1–6. doi: 10.1029/2010GL043489
Pubmed Abstract | Pubmed Full Text | CrossRef Full Text | Google Scholar
Pearce, J. A., Harris, N. B., and Tindle, A. G. (1984). Trace element discrimination diagrams for the tectonic interpretation of granitic rocks. J. Petrol. 25, 956–983.
Pearce, J. A., and Peate, D. W. (1995). Tectonic implications of the composition of volcanic arc magmas. Ann. Rev. Earth Planet. Sci. 23, 251–285.
Schwartz, J. J., Johnson, K., Miranda, E. A., and Wooden, J. L. (2011). The generation of high Sr/Y plutons following Late Jurassic arc-arc collision, Blue Mountains province, NE Oregon. Lithos 126, 22–41. doi: 10.1016/j.lithos.2011.05.005
Seligman, A., Bindeman, I., Jicha, B., Ellis, B., Ponomareva, V., and Leonov, V. (2014). Multi-cyclic and isotopically diverse silicic magma generation in an arc volcano: gorely eruptive center, Kamchatka, Russia. J. Petrol. 55, 1561–1594. doi: 10.1093/petrology/egu034
Seton, M., Muller, R. D., Zahirovic, S., Gaina, C., Torsvik, T., Shephard, G., et al. (2012). Global continental and ocean basin reconstructions since 200 Ma. Earth Sci. Rev. 113, 212–270. doi: 10.1016/j.earscirev.2012.03.002
Steinberger, B., and O'Connell, R. J. (1998). Advection of plumes in mantle flow: implications for hotspot motion, mantle viscosity and plume distribution. Geophys. J. Int. 132, 412–434.
Valley, J. W., Lackey, J. S., Cavosie, A. J., Clechenko, C. C., Spicuzza, M. A., Basei, M. A. S., et al. (2005). 4.4 billion years of crustal maturation: oxygen isotope ratios of magmatic zircon. Contrib. Mineral. Petrol. 150, 561–580. doi: 10.1007/s00410-005-0025-8
Watts, K., Bindeman, I., and Schmitt, A. (2011). Large-volume rhyolite genesis in caldera complexes of the Snake River Plain: insights from the Kilgore Tuff of the Heise Volcanic Field, Idaho, with comparison to Yellowstone and Bruneau-Jarbidge rhyolites. J. Petrol. 52, 1–34. doi: 10.1093/petrology/egr005
Wells, R. E., Bukry, D., Friedman, R., Pyle, D., Duncan, R., Haeussler, P., et al. (2014). Geologic history of Siletzia, a large igneous province in the Oregon and Washington Coast Range—correlation to the geomagnetic polarity timescale and implications for a long-lived Yellowstone Hot Spot. Geosphere 10, 692–719. doi: 10.1130/GES01018.1
Wells, R. E., Engebretson, D. C., Snavely, P. D., and Coe, R. S. (1984). Cenozoic plate motions and the volcano-tectonic evolution of western Oregon and Washington. Tectonics 3, 275–294.
Keywords: Yellowstone plume, oxygen isotopes, hafnium isotopes, Oregon, calderas, delamination
Citation: Seligman AN, Bindeman IN, McClaughry J, Stern RA and Fisher C (2014) The earliest low and high δ18O caldera-forming eruptions of the Yellowstone plume: implications for the 30–40 Ma Oregon calderas and speculations on plume-triggered delaminations. Front. Earth Sci. 2:34. doi: 10.3389/feart.2014.00034
Received: 26 September 2014; Accepted: 10 November 2014;
Published online: 28 November 2014.
Edited by:
Adelina Geyer, Institute of Earth Sciences Jaume Almera (ICTJA-CSIC), SpainReviewed by:
Timothy Druitt, Blaise Pascal University, FranceJörn-Frederik Wotzlaw, University of Geneva, Switzerland
Copyright © 2014 Seligman, Bindeman, McClaughry, Stern and Fisher. This is an open-access article distributed under the terms of the Creative Commons Attribution License (CC BY). The use, distribution or reproduction in other forums is permitted, provided the original author(s) or licensor are credited and that the original publication in this journal is cited, in accordance with accepted academic practice. No use, distribution or reproduction is permitted which does not comply with these terms.
*Correspondence: Angela N. Seligman, Department of Geological Sciences, University of Oregon, 100 Cascade, 1275 E 13th Ave., Eugene, OR 97403-1272, USA e-mail:c2VsaWdtYW5AdW9yZWdvbi5lZHU=