- 1Laboratorio de Paleomagnetismo del Instituto de Geofísica, Universidad Nacional Autónoma de México, Mexico City, Mexico
- 2Laboratorio Interinstitucional de Magnetismo Natural, Instituto de Geofísica, Unidad Michoacán, Morelia, Mexico
- 3Área de Prehistoria, Departamento de Ciencias Históricas y Geografía, Universidad de Burgos, España, Burgos, Spain
- 4Departamento de Edafología del Instituto de Geología, Universidad Nacional Autónoma de México, Mexico City, Mexico
- 5Centro de Investigaciones en Geografía Ambiental, Universidad Nacional Autónoma de México, Morelia, Mexico
Geomagnetic reversals are global phenomena that require several stratigraphic correlation and dating methods for their firm identifications. For about 50 years the paleomagnetists attempted to acquire as many detailed records as possible using the magnetic memory of sediments and lava flows. Yet, transitional field behavior remains poorly characterized largely because of sporadic aspect of volcanic eruptions. In some specific cases, paleosols such as those developed from alluvial or aeolian sediments, may also record the variations of the Earth's Magnetic Field across the polarity changes. Here, we report a detailed paleomagnetic and rock-magnetic investigation on some radiometrically dated chromic Luvisols located in Central Mexico carrying detrital or chemical remanent magnetization. The research was developed in order (i) to demonstrate the primary origin of the recorded magnetic remanence and (ii) to show that paleosols are good candidates to provide a high resolution record of the behavior of Earth magnetic field during geomagnetic reversals. The lower part of the paleosol sequence shows a clearly defined reverse polarity magnetization followed by geomagnetically unstable transitional field and ended by normal polarity remanence. Considering the K-Ar datings available at the bottom of the sequence, observed polarity changes most probably correspond to the Matuyama-Brunhes transition. Our AMS and rock magnetic data suggest that the magnetization is acquired during the initial stage of soil formation in context of active volcanic activity since the magnetic fabric is essentially sedimentary and reverse and normal polarity paleodirections are almost antipodal. Moreover, titanomagnetites are identified as main magnetic carriers of rock-magnetic measurements including thermomagnetics and hysteresis cycles. We propose that the transition recorded in this study correspond to the Brunhes-Matuyama boundary andabstractthat the chromic Luvisols are potentially good recorders of the paleosecular variation. The identification of the Brunhes-Matuyama boundary within the studied sequence has fundamental significance for improving the chronological scale of Tlaxcala paleosol-sedimentary sequence and its correlation with the global proxies.
Introduction
Several geomagnetic reversals have been documented from geological records. However, we still have limited evidence from paleomagnetic measurements about the patterns of change in the magnetic field during a polarity transition. A still debated question is whether reversals and secular variation are different features, or whether the polarity changes may be considered as an extreme expression of the secular variation. Because a polarity reversal is a global event, the geomagnetic reversals provide an effective mean of stratigraphic correlation and dating. Recognition of polarity changes and excursions as a part of the Earth's paleomagnetic field behavior has developed during the last five decades together with absolute age (astrochronological) calibration of the polarity timescale (Singer et al., 2002).
Brunhes (1907) and later Mercanton (1926) demonstrated first that volcanic rocks may record reverse polarity remanent magnetization. Later, Opdyke et al. (1966) found a reversal record in marine sediments. During a polarity transition, the virtual geomagnetic poles (VGP) follow different paths for different reversals (Prévot and Camps, 1993). Moreover, Earth's magnetic field has often departed for brief periods from its usual axial configuration without establishing a reversed direction. This fundamental property, which has been reported in lava flows and sediments worldwide, is known as geomagnetic excursion. The relatively short periods (103−8 × 103 after Gubbins, 1999) during which the geomagnetic field changes polarity are of considerable interest in our understanding of the physical processes in the Earth's liquid core that generate the geomagnetic field (see also Petronille et al., 2005).
Numerous geomagnetic events have been discovered in the previously believed stable Brunhes and Matuyama chrons (Laj and Channell, 2007) and the Matuyama-Brunhes transition was revisited by several authors because of its particularity to show a kind of precursor prior to the polarity change (Tauxe et al., 1996; Quidelleur et al., 2002; Singer et al., 2005 among others).
The last geomagnetic polarity reversal took place about 790 ka ago (Singer et al., 2002; Coe et al., 2004), marking the beginning of the present period of normal polarity—the Brunhes chron. Channell et al. (2010) recently reported that the mean age for the midpoint of M-B reversal is about 773.1 ka (SD = 0.4 ka), ~7 ka younger than the presently accepted astrochronological age for this polarity reversal (780–781 ka). Valet et al. (2012) analyzed seven most detailed geomagnetic transitions worldwide since 180 Ma including two B-M reversals recorded at Hawaii and Tahiti. They propose that reversing field is characterized by a precursor event, a full polarity change and rebound. The polarity “switch” seems to occur during in less than 1000 years which is too brief to be accurately recorded by the sedimentary rocks.
There is now a general agreement that volcanic rocks are the best recorders of geomagnetic transitions and reversals. However, sub-aerial volcanism is mainly characterized by sporadic eruptions with some periods of intense eruptions alternating with quiet intervals. This is well illustrated investigating several parallel sections in Hawaii (Herrero-Bervera and Valet, 1999; Herrero-Berrvera and Valet, 2005). They all recorded the same reversal but do not show the same successions of transitional directions. Incremental 40Ar/39Ar heating techniques reports ages for Hawaiian lavas from 783 ± 11 ka (Baksi and Farrar, 1990; Baksi et al., 1992) to 780. A mean unweighted value of 787 ± 1.9 ka was reported by Singer and Pringle (1996) from lavas of Maui, Chile, La Palma and Tahiti. A younger value of 783 ± 11 ka was obtained by Spell and McDougall (1992) from Valles lava by 40Ar/39Ar. Thus, due their sporadic character volcanic rocks are unable to deliver the complete picture of geomagnetic transition. Sediments can provide continuous records of magnetic field variation, while data from lavas, due to the sporadic nature of volcanic activity, yield rather discontinuous records of geomagnetic field variation.
Some rapidly deposited sedimentary rocks offered relatively continuous records of reversal transitions available. Certain lake sediments have also permitted to reveal important information to study the secular variation of the Earth's magnetic field over geological time. It is also true that the natural remanent magnetization in sediments is frequently post-DRM (depositional remanent magnetization) and secondary overprints are common (Barton et al., 1980). As underlined by Channell and Lehman (1997) the remanence is locked in over a depth that varies significantly from one to another sediment, depending on how the sediments are compacted, how benthic organisms mix them, differences in remanence acquisition or real differences in the timing record (Channell and Kleiven, 2000).
Soil magnetism emerged during the last two decades, it initially was used to detect the presence of pedogenic ferrimagnetic minerals (Thompson and Oldfield, 1986), particularly in loess-palaeosol sequences where measurements are used as proxy indicators of climatic change. The magnetic properties of soils are currently used for identifying soil-forming processes and to identify their possible links with paleoclimate (Maher and Thompson, 1995; Han et al., 1996). Recently, rock-magnetism was also applied to Quaternary and older paleosols, in order to identify their soil-forming processes and environments. The magnetic properties of paleosols of Permian age appear to record glacial-interglacial fluctuations in low-latitude Pangea (e.g., Tramp et al., 2004).
This study is aimed to investigate whether a multilayer quaternary paleosol formed essentially in volcanic context, and thus providing high magnetic signal, may be used in terms of recording the paleosecular variation and polarity transition. “La Barranca de Tlalpan” sequence was first investigated for paleoenvironmental purpose (Ortega-Guerrero et al., 2004; Sedov et al., 2009; Solis-Castillo et al., 2012), but some few oriented hand samples at the lower part yielded stable, reverse polarity magnetization. This fact was the main motivation to revisit and sample the whole sequence in greater details. We determine the characteristic magnetizations by AF demagnetization and employ AMS and rock magnetic studies to determine if the origin of these remanences is primary.
Geological Settings and Sampling
The Trans-Mexican Volcanic Belt (TMVB) is a major continental volcanic arc which spans about 1000 km across central Mexico from the Pacific to the Atlantic coast (Figure 1). The TMVB began to form in middle Miocene as a result of subduction of the Cocos and Rivera plates and covers the Eocene to early Miocene Sierra Madre Occidental volcanic province (Ferrari et al., 1999). The initial stage of the TMVB is marked by a widespread basaltic volcanism, occurring from the Pacific coast to Mexico City, to the north of the modern volcanic arc. This volcanism is characterized by plateau-like structures which have an aggregate volume estimated between 3200 and 6800 km3 (Ferrari et al., 2000). Geologic and stratigraphic studies have shown that these basaltic lavas were emplaced in a well defined period between 11 and 8 Ma (Ferrari et al., 1994, 2000; Moore et al., 1994; Righter et al., 1995). However, the volcanic activities continued during the Plio-Quaternary.
The “Barranca de Tlalpan” is a paleosol sequence near the city of Tlaxcala in the central part of the Trans Mexican Volcanic Belt (Figure 1). The studied sequence consists of seven pedostratigraphic units separated by ash layers. It is subdivided in four main layers based on morphologic characteristics: a modern soil, the Gray, the Brown and the Red Units, from top to bottom with a total depth of 1528 cm (Figure 2) (for more details see Rivas et al., 2006; Sedov et al., 2009; Solis-Castillo et al., 2012).
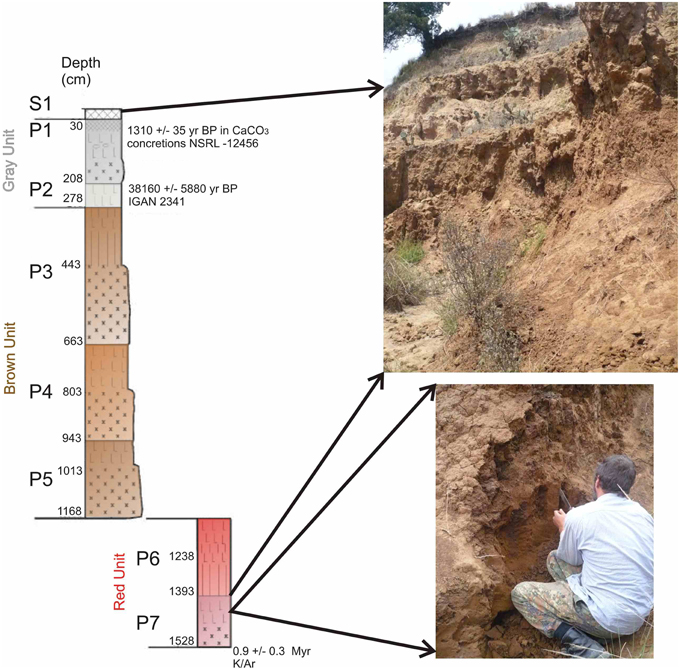
Figure 2. Paleosol sequence with the different units: Gray (30–278 cm), Brown (278–1168 cm) and Red (1168–1528 cm). Please see text for more details.
The 30 cm thick modern soil horizon shows evidence of numerous prehispanic artifacts like obsidian and ceramic fragments including colonial ones. A well marked erosion horizon defines a clear boundary with the underlying unit.
The Gray unit from 30 to 303 cm consists of two gray paleosols (P1 and P2), separated by a tepetate (Central American term for a kind of brittle volcanic rock) layer. The P1 (of 178 cm thickness) shows Ah, Bt and Cx horizons, the P2 (60 cm thickness) is partly truncated and only exhibits the Bt horizon (Sedov et al., 2009).
The Brown unit from 303 to 1168 cm has three brown paleosols (P3, P4, and P5), Fragic Luvisol, with well developed Bt horizons, underlying by tepetates consolidated subsoil tephtous horizons, (Sedov et al., 2009) now identified as Fragipan (IUSS Working Group WRB, 2006).
The Red unit from 1168 to 1528 cm includes two paleosols (P6 and P7) with reddish-brown Bt horizons. These paleosols are classified as Chromic Luvisols (Sedov et al., 2009). The P1 paleosol was dated by 14C on CaCO3 concretions with 1310 ± 35 year BP and calibrated age of 656–772 AD, a very young age produced by pedogenic carbonates (Sedov et al., 2009). The 14C dates of P2 paleosol on humus gave 38,160 ± 5880 and >33,595 year BP (Sedov et al., 2009). Finally, a 0.9 Ma date by K/Ar was obtained from the lowest layer, underlying P7 (Sedov et al., 2009). This date corresponds to very upper part of Matuyama reversed polarity chron.
Our sampling campaign was motivated to obtain oriented samples from P7 trough P5 profiles hoping to catch the last Matuyama-Brunhes reversal. The sampled section consists of two segments, one that corresponds to 1153–1439 cm (P5, P6, and part of P7, Figure 2 upper right side) and one from 1417 to 1528 cm (P7, Figure 2 lower right side).
The pedostratigraphic units surfaces were cleaned and later cutted with non-magnetic tools following pedologycal techniques to get rectangular blocks. These blocks were oriented with a Brunton compass (Figure 3). At laboratory the blocks were consolidated with a solution of vinil poliacetic resine and water. The consolidated blocks were cut with a non-magnetic tool to get standard cubes of 8 cm3 and introduced in plastic cubic sample boxes (Figure 3). A total of 125 samples were prepared and kept about 1 month in free magnetic field in order to diminish the effect of viscous remanent magnetization.
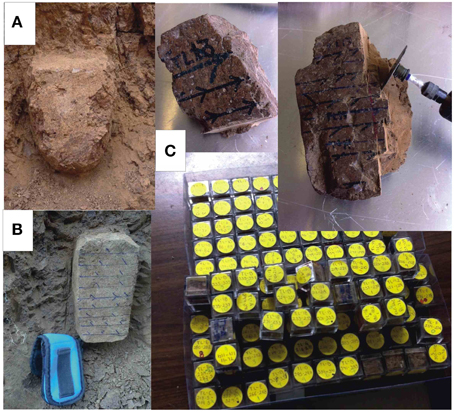
Figure 3. (A) In situ block (B) In situ block oriented (C) Sample preparation and cutting using a non-magnetic moto tool.
Methods
Representative samples from the studied palaeosols were analyzed in order to identify the main magnetic carriers, their domain state and thermomagnetic stability. With the aid of a Variable field Translation Balance (MM_MFTV) we measured progressive isothermal remanent (IRM) acquisition curves, hysteresis loops (±1 T), backfield coercivity curves and thermomagnetic curves up to 700°C in air. All these analyses were performed on bulk sample (~400 mg) extracted from 16 representative specimens at the laboratory of Palaeomagnetism of Burgos University (Spain). Curie temperatures were determined using the two-tangent method of Grommé et al. (1969).
The remanent magnetization of the samples were measured in a JR6 in a field free environment and demagnetized by alternating fields using 7–12 steps up to a maximum fields of 100 mT with Molspin demagnetizer. A detailed AMS analysis was performed on 117 samples from P5, P6 and P7 paleosols employing a KLY2 instrument and anisoft software for obtaining principal AMS directions and AMS parameters of shape T, anisotropy degree Pj and main susceptibility Km in SI units.
Results
IRM acquisition curves are almost saturated between 150 and 300 mT, indicating that the remanent magnetization is dominated by low coercivity minerals (i.e.,: magnetite/maghaemite). A small fraction of high coercivity minerals seems to be also present because the curves are not fully saturated at 1 T. However, its contribution to the total remanent magnetization do not exceeds 5%. Thus, it cannot be considered really significant (Figure 4).
Some representative thermomagnetic curves (saturation magnetization vs. temperature) are shown in Figure 5. In the heating cycles of the studied samples several slope changes at ~150–200°C, ~510°C and ~580°C were observed. The ferromagnetic phase with a Curie point of ~150—200°C is characteristic of Ti-rich Titanomagnetite and is particularly evident in samples TLG 212–214 5b (1396 cm depth) and TL-F 256.5–258.5 (1438 cm depth, Figures 5A,B). The second slope change observed around 510°C in the heating curves most probably corresponds to a slightly substituted magnetite and the phase with a Tc around 580°C is magnetite. Occasionally, this latter phase displays higher Curie temperatures up to 600–610°C (e.g.,: Figure 5C) which is interpreted as magnetite partially maghaemitized due to oxidation (TL 13 148.5–150.5, 1334 cm depth). Finally, three samples exhibit also a subtle inflection on the heating curve around 320°C, which is absent in their respective cooling cycles (e.g.,: Figure 5D, TL 18 243–245, 1425 cm depth). We do not have evidences of ferromagnetic sulphurs (e.g.,: pyrrothite or greigite) in these sediments, so this inflection might be attributable to maghaemite which partially inverts to (titano) haematite. The magnetite Tc appears in all cooling curves and in most cases also reproduces the inflection around 150–200°C. Within their variability, the cooling cycles exhibit a relatively high thermal stability and all samples except one (Figure 5D) display cooling cycles of lower intensity than heating ones. On the basis of these results, remanent magnetization prove to reside in Titanomagnetites (with variable Ti-content), as one may expect considering the volcanoclastic origin of the deposit.
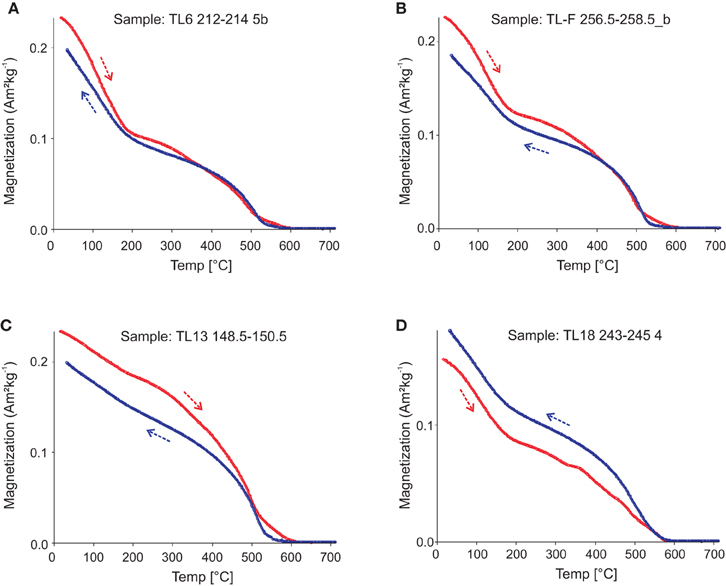
Figure 5. (A–D) Representative thermomagnetic curves from the Tlalpan Paleosols. The sample code and magnetization values are indicated for each specimen. Heating (red) and cooling (blue) cycles are shown with their respective arrows.
The Figure 6A show hysteresis cycles of some selected samples belonging to P5, P6, and P7. Considering that these samples are dominated by titano-magnetites, the hysteresis parameters (saturation magnetisation, Ms; remanence saturation magnetisation, Mrs; and coercive field, Hc) together with remanence coercivity (Bcr -determined separately from the back field curves-) were plotted in the so-called Day plot (Day et al., 1977) to estimate the domain state of the sample set (Figure 6B). Bc and Bcr ranges between 5.02–8.83 mT and 22.89–27.65 mT, respectively, in general accordance with IRM acquisition curves. The hysteresis ratios range from 0.09 < Mrs/Ms < 0.14 and 2.90 < Bcr/Bc < 4.68, indicative of (Ti) magnetite in pseudo- single domain state (Day et al., 1977; Dunlop, 2002). It is striking how the hysteresis parameters of these samples are rather uniform, suggesting small granulometric variations among paleosols. Interestingly, the presence of ultrafine (~10–30 nm) magnetite particles is significant when compared with the mixing theoretical lines of Dunlop (2002) and this agrees well with the results already observed by Ortega-Guerrero et al. (2004) specifically in these paleosols (P5, P6, and P7).
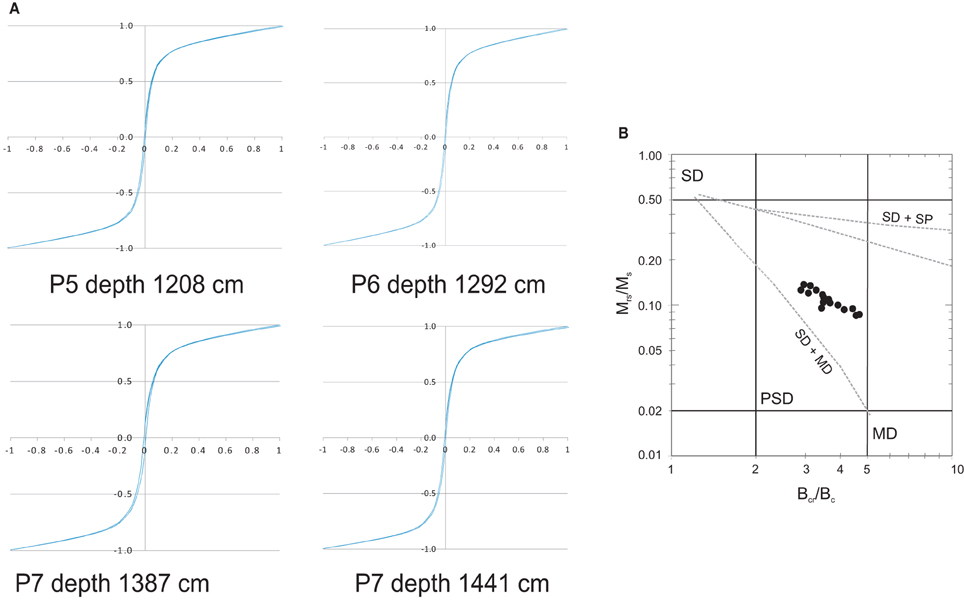
Figure 6. (A) Representative hysteresis cycles of selected paleosols (B) Mrs/Ms vs. Bcr/Bc logarithmic plot (Day et al., 1977) for representative samples of the Tlalpan Paleosols. The dashed lines represent mixing curves taken from Dunlop (2002) for mixtures of single-domain (SD) with multidomain (MD) or superparamagnetic (SP) magnetite particles.
The characteristic magnetization directions were obtained by principal component analysis on stepwise demagnetization data with remasoft 3.0 software (Chadima and Hrouda, 2006). Some representative orthogonal vector plots (so-called Zijdervel diagrams) are shown on Figure 7. A characteristic magnetization was determined by the least squares method (Kirschvink, 1980), with 4–9 points used for this determination. A secondary component, probably of viscous origin was present in an important number of samples, but was removed after applying an alternative peak field of 10 mT (Figure 7). Moreover, some few samples show slight deviation from the origin mainly at high alternating peak fields. However in any case, the maximum angular deviation to calculate the characteristic directions was less than 3°C. The median destructive fields (MDF) range mostly from 20 to 40 mT, suggesting pseudo-single domain grains as remanent magnetization carriers (Dunlop and Özdemir, 1996).
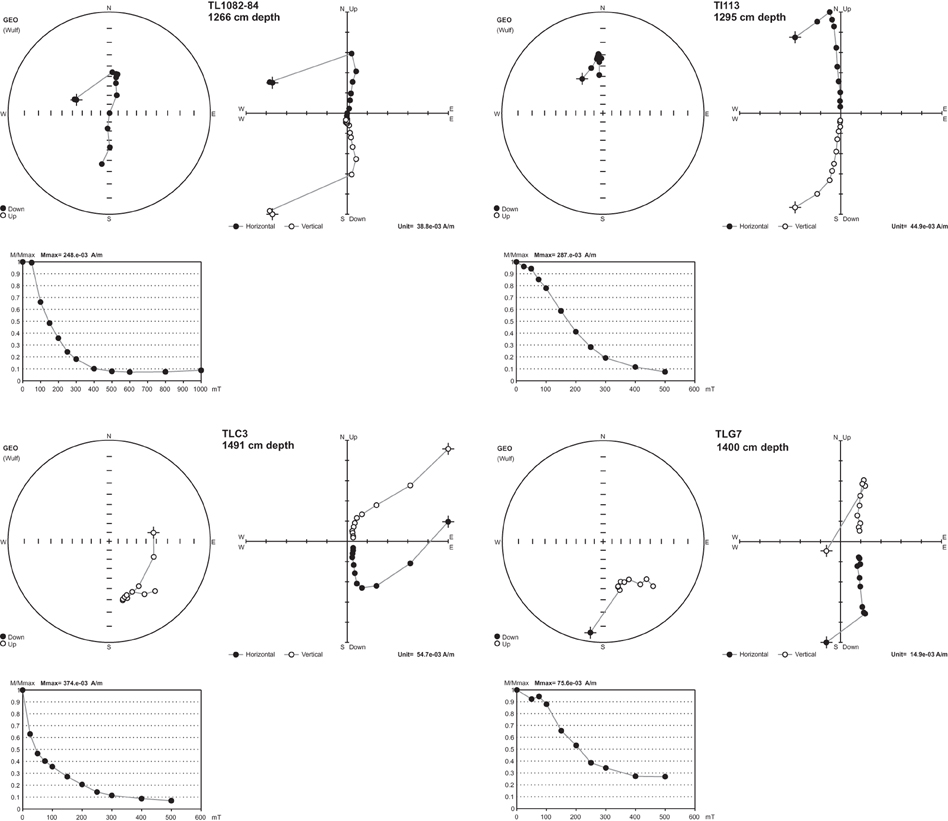
Figure 7. Equal area projection, Orthogonal diagram and Demagnetization spectra for some representative samples: TL10-82-84, TL11-3, TlC3, andTLG7.
The susceptibility of magnetic anisotropy (AMS) analysis is not common practice for paleosols. However, the objective of employing it was to estimate whether magnetic fabric is sedimentary. A detailed AMS analysis was performed with 117 samples from P5, P6, and P7 paleosols employing a KLY2 instrument and anisoft software. Since the Brunhes-Matuyama reversal was observed along the P6 paleosol profile, special attention was focused along this profile in order to detect possible AMS changes that could give some insight about soil formation processes that could support or reject the reliability of observed reversal (Figure 8).
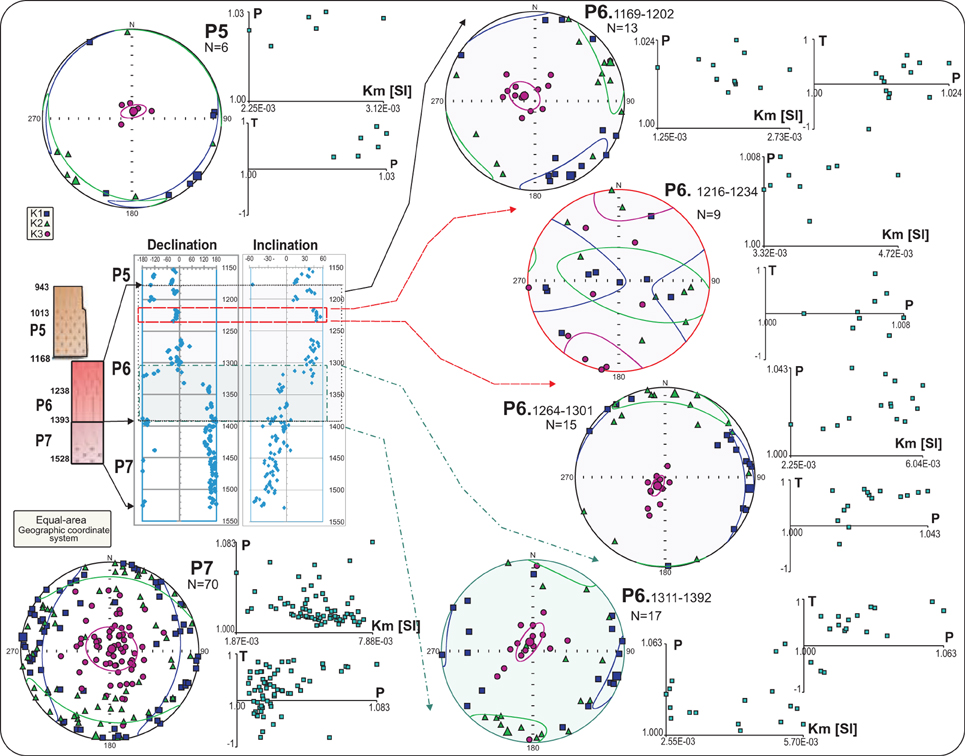
Figure 8. AMS directional results in equal-area equatorial diagrams and corresponding graphs of AMS parameters, referred to characteristic magnetization results of soil profile. P5 and P7 are shown in one set of graphs each. P6 results are shown in 4 sections, depth of each is labeled in centimeters next to the P6 label; N is the number of samples. The lower P6 (1311–1392) section corresponds to the section of polarity switching which shows a typical primary fabric. P6 transect in red shows a disordered AMS results corresponding apparently to illuvial process in a B horizon.
AMS results suggest sedimentary magnetic fabric all along P5, P7, and most of P6, in which the k3 mean directions are around vertical positions with narrow error ellipsoids, meanwhile the k1 and k2 directions are around sub-horizontal and scattered positions. Only along a short interval in P6 profile (1235–1215), the AMS shows a most disorganized fabric on which it seems to have a tendency of subvertical k1 and subhorizontal k3 directions.
AMS results above and below the turn point of the Brunhes Matuyama reversal in P6, as well as AMS results from most of the studied profiles, suggest that paleosols are from an alluvial origin, as would be expected in Luvisols (P6 and P7); and that pedogenic process did not change the original magnetic fabric in most part of paleosol profiles. Only along the 1235–1215 interval, which correspond to a middle part of the P6 paleosol, apparently to a Bt paleo-horizon, the illuvial processes seem to have been strong enough to alter the original sedimentary fabric and produce the magnetic fabric observed.
The Figure 9 shows the evolution of magnetic declination (blue diamonds), inclination (red diamonds) and intensity (green diamonds) vs. depth. Lowest layer studied (between 1505 and 1434 cm) show mean inclination of −44.4° (orange square, Figure 9) and declination fluctuating around 175° yielding the evidence of clear reversed polarity paleodirections as may be expected for the Matuyama chron. Then, inclinations become shallower with the mean value of −34°. Between 1319 and 1436 cm (red square, Figure 9) the inclination swings from positive to negative defining a transitional zone of clear intermediate polarity. This phenomenon seems to be a common event prior to the Matuyama-Brunhes reversal. The upper layers (green zone on Figure 9 and beyond) yielded a mean paleoinclination of 44.3° and paleodeclination of 344°. A single sample belonging to P5 shows the negative inclination which we attribute to some measurement artifact rather than true feature of the Earth's Magnetic Field just after the reversal.
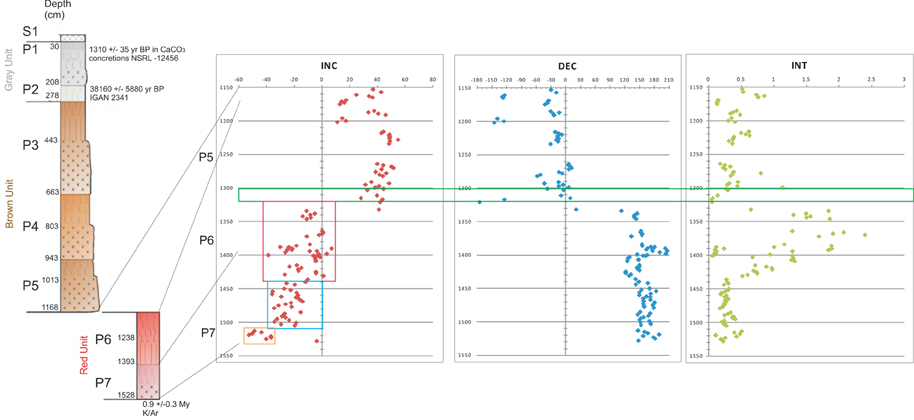
Figure 9. Paleosols sequence with depths in cm. Inclination, Declination of characteristic magnetization and NRM Intensity. Four sections have been recognized: the green rectangle (1300–1319 cm) when the inversion starts, the red rectangle (1318–1438 cm) where the inclination swings from positive to negative and vice versa, the blue rectangle (1438–1505 cm) with a mean negative inclination of 34.4° and finally the orange rectangle where the inversion ends with a mean inclination of 44.4°. The full data appears at the Supplementary Table 1.
The intensity (green diamonds, Figure 9) shows a clear decrease in the section of the green zone followed by large fluctuations in the red zone and finally recovering the same pre-transitional mean value.
The mean magnetic direction of the normal component (N = 53) has an Inclination: 54.7°, Declination: 324.8°, the reverse component (N = 73) has an inclination: 21.4°, Declination: 336.0°. Using 60° of paleolatitude as cut of angle to separate the transitional field and stable geomagnetic (normal or reversed) directions because it is widely used in literature, we have 29 normal and 41 reverse polarity (full and open circles respectively, Figure 10) with critical angle γ = 5.76° which corresponds to positive type B reversal test following McFadden and McElhinny's (1990) classification. Interestingly, the cut of angle given by Vandamme's iterative method Vandamme (1994) is 61°, very similar to our preferred cut of angle pointing to γ = 5.76° It should be also noted that the cut of angle of Vandamme guaranty the Fisherian distribution of data. We also estimated the “antipodality” through reversal test using 45° obtaining the critical angle above 10° (11.65°) pointing still positive, but Type B reversal test. The Figure 10 showing exclusively data selected using a 60° paleolatitude cut of angle, also shown mean directions and α95 values for normal (Dec:350.9°, Inc:43.0°, α95:3.8°) and reverse components (Dec:347.2°, Inc:26.7°, α95:4.1°), the fact that these α95's do not overlap may be interpreted as the asymmetry between the pre and post-transitional record and the difficulties to accurate remanence acquisition, as already shown in previous works analyzing sediments and soils (Kong et al., 2014 among others).
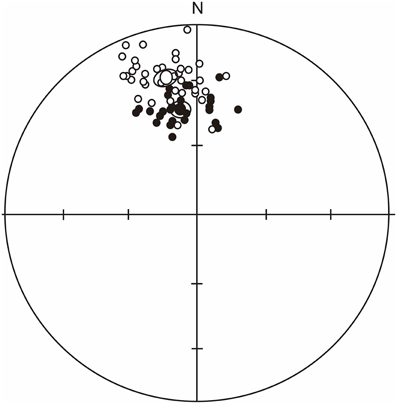
Figure 10. Mean magnetic directions of normal and reverse components with a95 of a data selected using a 60° paleolatitude cut of angle. The mean Normal direction of 29 samples has 43.0° of Inclination, 350.9° of Declination and an α95 = 3.8°. The mean Reverse direction of 41 samples has 26.8° of Inclination, 347.2° of Declination and an α95 = 4.1°. Normal (full circles) and Reverse (empty circles) magnetic directions show a positive reversal test type B.
Discussion and Concluding Remarks
The type of remanent magnetization carried by these paleosols may be a matter of debate. It is well known that the NRM in sedimentary rocks is often a DRM or post-DRM and secondary overprints are not uncommon (e.g., Dunlop and Özdemir, 1996). Nevertheless, a few marine and terrestrial sedimentary cores (particularly for rapidly deposited sediments) have produced some of the best continuous records of reversal transitions available. Liu and Zhang (2013) showed that the chemical remanent magnetization (CRM) is the main mode of remanence due to pedogenesis. Thus, an extreme caution is required to interpret the paleomagnetic signal in paleosol as true geomagnetic characteristics. This is probably the main reason to explain why loess paleosol sequence yield distinct magnetic record during the M–B transition than records from marine sediments (Kong et al., 2014). In case of Tlalpan paleosols, our preferred interpretation is detrital or post-detrital remanence since the magnetic fabric is essentially sedimentary and reverse and normal polarity paleodirections are almost antipodal. As underlined by Suganuma et al. (2011), the lock-in of post-detrital remanent magnetization may be delayed but with relatively little distortion of the geomagnetic signal. We obviously admit the possibility of chemical remanence but their direction should be recorded parallel to the original DRM.
The identification of the BM boundary within the Red Unit has fundamental significance for improving the chronological scale of Tlaxcala paleosol-sedimentary sequence and its correlation with the global proxies. Before of this finding, the only instrumental time marker available for the lower part of the exposure was the K-Ar date obtained from the basal tepetate of the Red Unit. Establishing precise and confinable position of BM boundary provides an independent chronological marker, consistent with previous datings. Furthermore this finding facilitates correlation of the Tlaxcalan sequence with the Marine Oxygen Isotope curve and loess-paleosol sequences where BM boundary is well established (Hambach, 2012). The BM magnetic reversal is now accepted as the limit between low and middle Pleistocene. Its presence in Tlaxcala sequence allows considering it as the unique record of the Mid-Pleistocene Climate Transition period (MPT) (Mudelsee and Stattegger, 1997), known for the territory of Mexico.
The occurrence of intermediate directions just before transition is also evidenced by Quidelleur et al. (2002) (see also Quidelleur and Valet, 1996) who reported first volcanic evidence of a geomagnetic excursion occurring some 40 ka prior to the Matuyama-Brunhes transition. An age of 821 ± 13 ka was obtained using the Cassignol K-Ar technique for a transitionally magnetized flow. In other hand, Singer et al. (2002) obtained the mean age of 822.2 ± 8.7 ka on three intermediate polarity lava flows (again from Canaries) using 40Ar-39Ar systematics. Their absolute paleointensity gave relatively low values as commonly accepted for transitions and large departures from the geomagnetic axial dipole (Goguitchaichvili et al., 1999). Petronille et al. (2005) reported same evidence studying Ceboruco lava flows at the Western Trans-Mexican Volcanic Belt. More evidences for a geomagnetic event just prior to M-B reversal comes from the sedimentary records (Kent and Schneider, 1995; Hartl and Tauxe, 1996; Carcaillet et al., 2004). These authors detected shallow inclinations and relatively low paleointensity prior to M-B transition.
Sagnotti et al. (2014) demonstrated that unusually rapid directional change may be occurred in some lake sediments at an extremely fast rate, estimated to be less than 2° per year, with no intermediate paleodirections documented during the polarity change. This is supported by the fact that a mean sediment accumulation rate is of about 0.2 mm year−1 during the transition. We should however note that in our particular case it is extremely difficult to estimate the deposition rate in soils because of obvious difference with the lacustrine sediments. An abrupt directional change occurs at about 1330 cm depth (characteristic mean inclination jumps from ca. −20° to ca. 40° while declination from ca. 120° to ca. 0°).
In summary, we propose that Tlalpan paleosol records Matuyama-Brunhes reversal at the lower part of sequence. This opens new opportunities to study the fine characteristics of the Earth's Magnetic Field on chromic Luvisols worldwide.
Conflict of Interest Statement
The authors declare that the research was conducted in the absence of any commercial or financial relationships that could be construed as a potential conflict of interest.
Acknowledgments
AG acknowledges the financial support provided by UNAM - PAPIIT n° IN105214 and CONACYT n° 129653 and 169915. We also thanks the financial support of the project CGL2012-32149, MINECO.
Supplementary Material
The Supplementary Material for this article can be found online at: http://www.frontiersin.org/journal/10.3389/feart.2015.00011/abstract
Supplementary Table 1. Characteristic magnetisation declination (CHRMdec), inclination (CHRMinc) and Maximum Angular Deviation (MAD), demagnetization steps (demag steps), number of steps (# steps) and NRM intensity of Paleosol Tlalpan obtained per sample.
References
Baksi, A. K., and Farrar, E. (1990). Evidence for errors in the geomagnetic polarity time-scale at 17–15 Ma: 40Ar/39Ar dating of basalts from the Pacific Northwest, USA. Geophys. Res. Lett. 17, 1117–1120. doi: 10.1029/GL017i008p01117
Baksi, A. K., Hsu, V., McWilliamsm, M. O., and Farrar, E. (1992). 40Ar/39Ar dating of the Brunhes-Matuyama geomagnetic reversal. Science 256, 356–357.
Barton, C., McElhinny, M., and Edwards, D. (1980). Laboratory studies of depositional DRM. Geophys. J. R. Astron. Soc. 61, 355–377.
Brunhes, D. (1907). Recherches sur les directions d'aimantation des roches volcaniques, J. Phys. 5, 705–724.
Carcaillet, J. T., Bourles, D., and Thouveny, N. (2004). Geomagnetic dipole moment and 10Be production rate intercalibration from authigenic 10Be/9Be for the last 1.3 Ma. Geochem. Geophys. Geosys. 5, 1–13. doi: 10.1029/2003GC000641
Chadima, M., and Hrouda, F. (2006). Remasoft 3.0 a user-friendly paleomagnetic data browser and analyzer. Trav. Géophys. XXVII, 20–21.
Channell, J. E. T., Hodell, D. A., Singer, B. S., and Xuan, C. (2010). Reconciling astrochronological and 40Ar/39Ar ages for the Matuyama-Brunhes boundary and late Matuyama Chron. Geochem. Geophys. Geosys. 11, Q0AA12. doi: 10.1029/2010GCG003203.
Channell, J. E. T., and Kleiven, H. F. (2000). Geomagnetic Paleointensities and astrochronological ages for the Matuyama-Brunhes boundary and the boundaries of the Jaramillo Subchron: palaeomagnetic and oxygen isotope records from ODP Site 983, Phylos. Trans. R. Soc. Lond. Ser. A 358, 1027–1047. doi: 10.1098/rsta.2000.0572
Channell, J. E. T., and Lehman, B. (1997). The last two geomagnetic polarity reversals recorded in high-deposition-rate sediment drifts. Nature 389, 712–715.
Coe, R. S., Singer, B. S., Pringle, M., and Zhao, X. (2004). Matuyama-Brunhes reversal and Kamikatsura event on Maui: paleomagnetic directions, 40Ar/39Ar ages and implications. Earth Planet. Sci. Lett. 222, 667–684. doi: 10.1016/j.epsl.2004.03.003
Day, R., Fuller, M. D., and Schmidt, V. A. (1977). Hysteresis properties of titanomagnetites grain size and composition dependence. Phys. Earth Planet. Inter. 13, 260–266.
Dunlop, D. J. (2002). Theory and application of the Day plot (Mrs/Ms versus Hcr/Hc) 2. Application to data for rocks, sediments, and soils. J. Geophys. Res. 107, 1–15. doi: 10.1029/2001JB000487
Dunlop, D. J., and Özdemir, O. (1996). Rock Magnetism: Fundamentals and Frontiers. Cambridge; New York, NY: Cambridge University Press.
Ferrari, L., Conticelli, S., Vaggelli, C., Petrone, C., and Manetti, P. (2000). Late miocene mafic volcanism and intra-arc tectonics during the early development of the Trans-Mexican Volcanic Belt. Tectonophysics 318, 161–185. doi: 10.1016/S0040-1951(99)00310-8
Ferrari, L., Garduño, V. H., Innocenti, F., Manetti, P., Pasquarè, G., and Vaggelli, G. (1994). A widespread mafic volcanic unit at the base of the Mexican Volcanic Belt between Guadalajara and Queretaro. Geofísica Int. 33, 107–124.
Ferrari, L., Lopez-Martinez, M., Aguirre-Diaz, G., and Carrasco-Nuñez, G. (1999). Space-time patterns of Cenozoic arc volcanism in central Mexico: from the Sierra Madre Occidental to the Mexican Volcanic Belt. Geology 27, 303–306.
Goguitchaichvili, A., Prévot, M., and Camps, P. (1999). No evidence for strong fields during the R3-N3 Icelandic geomagnetic reversals. Earth Planet. Sci. Lett. 167, 15–34.
Gromm,é, C. S., Wright, T. L., and Peck, D. L. (1969). Magnetic properties and oxidation of iron-titanium oxide minerals in Alae and Makaopuhi lava lakes, Hawaii. J. Geophys. Res. 74, 5277–5294.
Gubbins, D. (1999). The distinction between geomagnetic excursions and reversals, Geophys. J. Int. 137, F1–F3.
Hambach, U. (2012). The Matuyama-Brunhes Boundary in the Stari Slankamen loess section (Vojvodina, Serbia): its detailed record and its stratigraphic position. Q. Int. 279–280, 186. doi: 10.1016/j.quaint.2012.08.302
Han, J., Lu, H., Wu, N., and Guo, Z. (1996). Magnetic susceptibility of modern soils in China and climate conditions. Stud. Geophys. Geodaetica 40, 262–275.
Hartl, P., and Tauxe, L. (1996). A precursor to the Matuyama/Brunhes transition–field instability as recorded in pelagic sediments. Earth Planet. Sci. Lett. 138, 121–135.
Herrero-Berrvera, E., and Valet, J. P. (2005). Absolute paleointensity from the Waianae volcanics (Oahu, Hawaii) between the Gilbert-Gauss and the upper Mammoth reversals. Earth Planet. Sci. Lett. 234, 279–296. doi: 10.1016/j.epsl.2005.02.032
Herrero-Bervera, E., and Valet, J. P. (1999). Paleosecular variation during sequential geomagnetic reversals from Hawaii. Earth Planet. Sci. Lett. 171, 139–148.
IUSS Working Group WRB. (2006). World reference base for soil resources 2006: Rome, Food and Agriculture Organization of the United Nations (FAO), World Soil Resources Reports No.103, 2nd Edn. (Rome), 128.
Kent, D. V., and Schneider, D. A. (1995). Correlation of paleointensity variation records in the Brunhes/Matuyama polarity transition interval, Earth Planet. Sci. Lett. 129, 135–142.
Kirschvink, J. L. (1980). The least-squares line and plane and the analysis of palaeomagnetic data. Geophys. J. Int. 62, 699–718.
Kong, X., Zhou, W., Beck, J. W., Xian, F., and Wu, Z. (2014). Asynchronous records of Brunhes/Matuyama reversal in marine sediments and Chinese loess: review and discussion. Q. Int. 319, 137–142. doi: 10.1016/j.quaint.2013.08.001
Laj, C., and Channell, J. E. T. (2007). “Geomagnetic excursions. C,” in Treatise in Geophysics: Vol. 5 Geomagnetism, ed M. Kono (Amsterdam: Elservier), 373–416.
Liu, W., and Zhang, L. (2013). Chemical magnetization in Chinese loess. Phys. Earth Planet. Inter. 218, 14–18. doi: 10.1016/j.pepi.2013.02.005
Maher, B. A., and Thompson, R. (1995). Quaternary Climates, Environments and Magnetism. Cambridge: Cambridge University Press.
McFadden, P. L., and McElhinny, M. W. (1990). Classification of reversal test in palaeomagnetism. Geophys. J. Int. 103, 725–729.
Mercanton, P. L. (1926). Inversion de l'inclinaison magnétique terrestres aux ages géologiques. Terr. Magn. Atmosph. Elec. 31, 187–190.
Moore, G., Marone, C., Carmichael, I. S. E., and Renne, P. (1994). Basaltic volcanism and extension near the intersection of the Sierra Madre volcanic province and the Mexican Volcanic Belt. Geol. Soc. Am. Bull. 106, 383–394.
Mudelsee, M., and Stattegger, K. (1997). Exploring the structure of the mid-Pleistocene revolution with the advanced methods of time-series analysis. Geol. Rundsch. 86, 499–511.
Opdyke, N. D., Glass, B., Hays, J. D., and Foster, J. H. (1966). Palaeomagnetic study of Antarctic deep sea cores Science 154, 349.
Ortega-Guerrero, B., Sedov, S., Solleiro-Rebolledo, E., and Soler, A. (2004), Magnetic mineralogy in Barranca Tlalpan exposure paleosols, Tlaxcala, Mexico. Rev. Mex. Cienc. Geol. 21, 120–132.
Petronille, M., Goguitchaichvili, A., Henry, B., Alva-Valdivia, L. M., Rosas-Elguera, J., Urrutia-Fucugauchi, J., et al. (2005). Paleomagnetism of Ar-Ar dated lava flows from the Ceboruco-San Pedro volcanic field (western Mexico): evidence for the Matuyama-Brunhes transition precursor and a fully reversed geomagnetic event in the Brunhes chron. J. Geophysical Res. 110, 1–11. doi: 10.1029/2004JB003321
Prévot, M., and Camps, P. (1993). Absence of preferred longitude sectors for poles from volcanic records of geomagnetic reversals. Nature 366, 53–57.
Quidelleur, X., Carlut, J., Gillot, P. Y., and Soler, V. (2002). Evolution of the geomagnetic field prior to the Matuyama-Brunhes transition: radiometric dating of a 820 ka excursion at La Palma. Geophys. J. Int. 151, F6–F10. doi: 10.1046/j.1365-246X.2002.01841.x
Quidelleur, X., and Valet, J. P. (1996), Geomagnetic changes across the last reversal recorded in lava flows from La Palma (Canary Islands). J. Geophys. Res. 101, 13755–13773.
Righter, K., Carmichael, I. S. E., and Becker, T. (1995). Pliocene-Quaternary volcanism and faulting at the intersection of the Gulf of California and the Mexican Volcanic Belt. Geol. Soc. Am. Bull. 107, 612–626.
Rivas, J., Ortega, B., Sedov, S., Solleiro, E., and Sycheva, S. (2006). Rock magnetism and pedogenetic processes in Luvisol profiles: examples from Central Russia and Central Mexico. Q. Int. 156/157, 212–223. doi: 10.1016/j.quaint.2006.05.007
Sagnotti, L., Scardia, G., Giaccio, B., Liddicoat, J. C., Nomade, S., Renne, P. R., et al. (2014). Extremely rapid directional change during Matuyama-Brunhes geomagnetic polarity reversal. Geophys. J. Int. 199, 1110–1124. doi: 10.1093/gji/ggu287
Sedov, S., Solleiro-Rebolledo, E., Terhorst, B., Solé, J., Flores-Delgadillo, M. L., Werner, G., et al. (2009). The Tlaxcala basin paleosol sequence: a multiscale proxy of middle to late Quaternary environmental change in central Mexico. Rev. Mex. Cienc. Geol. 26, 448–465.
Singer, B. S., Hoffman, K. A., Coe, R. S., Brown, L. L., Jicha, B. R., Pringle, M. S., et al. (2005). Structural and temporal requirements for geomagnetic reversal deduced from lava flows. Nature 434, 633–636. doi: 10.1038/nature03431
PubMed Abstract | Full Text | CrossRef Full Text | Google Scholar
Singer, B. S., and Pringle, M. S. (1996). Age and duration of the Matuyama-Brunhes geomagnetic polarity reversal from 40Ar/39Ar incremental heating analyses of lavas. Earth Planet. Sci. Lett. 139, 47–61.
Singer, B. S., Relle, M. K., Hoffman, K. A., Battle, A., Laj, C., Guillou, H., et al. (2002). Ar/Ar ages from transitionally magnetized lavas on La Palma, Canary Island, and the geomagnetic instability timescale. J. Geophys. Res. 107, EPM 7-1–EPM 7-20. doi: 10.1029/2001JB001613
Solis-Castillo, B., Sedov, S., Solleiro-Rebolledo, E., and Salcido-Berkovich, C. (2012). Paleosuelos en secuencias coluvio-aluviales del Pleistoceno –Holoceno en Tlaxcala: registros paleoambientales del poblamiento temprano en el centro de México. Bol. Soc. Geol. Mexi. 64, 91–108.
Spell, T. L., and McDougall, I. (1992). Revisions to the age of the Brunhes-Matuyama boundary and the pleistocene geomagnetic polarity timescale. Geophys. Res. Lett. 19, 1181–1184. doi: 10.1029/92GL01125. issn: 0094-8276
Suganuma, Y., Okuno, J., Heslop, D., Roberts, A. P., Yamazaki, T., and Yokoyama, Y. (2011). Post-depositional remanent magnetization lock-in for marine sediments deduced from 10Be and paleomagnetic records through the Matuyama–Brunhes boundary. Earth Planet. Sci. Lett. 311, 39–52. doi: 10.1016/j.epsl.2011.08.038
Tauxe, L., Herbert, T., Shackleton, N. J., and Kok, Y. S. (1996). Astronomical calibration of the Matuyama-Brunhes boundary consequences for magnetic remanence acquisition in marine carbonates and the Asian loess sequences. Earth Planet. Sci. Lett. 140, 133–146.
Thompson, R., and Oldfield, F. (1986). “Environmental Magnetism,” in eds Allen and Unwin (London: Springer), 227.
Tramp, K. L., Soreghan, G. S. L., and Elmore, R. D. (2004). Paleoclimatic inferences from paleopedology and magnetism of the Permian Maroon Formation loessite, Colorado, USA. Geol. Soc. Am. Bull. 116, 671–686. doi: 10.1130/B25354.1
Valet, J. P., Fournier, A., Courtillot, V., and Herrero-Bervera, E. (2012). Dynamical similarity of geomagnetic field reversals. Nature 490, 89–94. doi: 10.1038/nature11491
PubMed Abstract | Full Text | CrossRef Full Text | Google Scholar
Keywords: paleomagnetism, rock-magnetism, geomagnetic reversal, paleosols, central Mexico
Citation: Soler-Arechalde AM, Goguitchaichvili A, Carrancho Á, Sedov S, Caballero-Miranda CI, Ortega B, Solis B, Morales Contreras JJ, Urrutia-Fucugauchi J and Bautista F (2015) A detailed paleomagnetic and rock-magnetic investigation of the Matuyama-Brunhes geomagnetic reversal recorded in the tephra-paleosol sequence of Tlaxcala (Central Mexico). Front. Earth Sci. 3:11. doi: 10.3389/feart.2015.00011
Received: 22 December 2014; Accepted: 04 March 2015;
Published: 20 April 2015.
Edited by:
Fabio Florindo, Istituto Nazionale di Geofisica e Vulcanologia, UKReviewed by:
Eric Font, University of Lisbon, PortugalLeonardo Sagnotti, Istituto Nazionale di Geofisica e Vulcanologia, Italy
Copyright © 2015 Soler-Arechalde, Goguitchaichvili, Carrancho, Sedov, Caballero-Miranda, Ortega, Solis, Morales Contreras, Urrutia-Fucugauchi and Bautista. This is an open-access article distributed under the terms of the Creative Commons Attribution License (CC BY). The use, distribution or reproduction in other forums is permitted, provided the original author(s) or licensor are credited and that the original publication in this journal is cited, in accordance with accepted academic practice. No use, distribution or reproduction is permitted which does not comply with these terms.
*Correspondence: Ana M. Soler-Arechalde, Instituto de Geofisica, Universidad Nacional Autónoma de México, Circuito Institutos s/n, Ciudad Universitaria, Coyoacán, Mexico City, ZC 04510 DF, MexicoYW5lc29sZXJAZ2VvZmlzaWNhLnVuYW0ubXg=