- 1Department of Environmental Sciences, University of Basel, Basel, Switzerland
- 2Department for Environment, Constructions and Design, Institute of Earth Sciences, University of Applied Sciences of Southern Switzerland, Canobbio-Lugano, Switzerland
- 3Office for Water Protection and Supply, Bellinzona, Switzerland
The Lake Lugano North Basin has been meromictic for several decades, with anoxic waters below 100 m depth. Two consecutive cold winters in 2005 and 2006 induced exceptional deep mixing, leading to a transient oxygenation of the whole water column. With the ventilation of deep waters and the oxidation of large quantities of reduced solutes, the lake's total redox-balance turned positive, and the overall hypolimnetic oxygen demand of the lake strongly decreased. The disappearance of 150 t dissolved phosphorous (P) during the first ventilation in March 2005 is attributed to the scavenging of water-column-borne P by newly formed metal oxyhydroxides and the temporary transfer to the sediments. The fixed nitrogen (N) inventory was reduced by ~30% (~1000 t). The water-column turnover induced the nitratation of the previously NO-free deep hypolimnion by oxidation of large amounts of legacy NH and by mixing with NO-rich subsurface water masses. Sediments with a strong denitrifying potential, but NO-starved for decades, were brought in contact with NO-replete waters, invigorating benthic denitrification and rapid fixed N loss from the lake in spite of the overall more oxygenated conditions. Similarly, a large microbial aerobic CH4 oxidation (MOx) potential in the hypolimnion was capitalized upon ventilation of the deep basin. Almost all CH4, which had been built up over more than 40 years (~2800 t), was removed from the water column within 30 days. However, boosted MOx could only partly explain the disappearance of the CH4. The dominant fraction (75%) of the CH4 evaded to the atmosphere, through storage flux upon exposure of anoxic CH4-rich water to the atmosphere. As of today, the North Basin seems far from homeostasis regarding its fixed N and CH4 budgets, and the deep basin's CH4 pool is recharging at a net production rate of ~66 t y−1. The size of impending CH4 outbursts will depend on the frequency and intensity of exceptional mixing events in the future.
Introduction
Anthropogenic nutrient inputs into lakes have been widely recognized as the main driver of lacustrine eutrophication. A cascading set of negative consequences of eutrophication includes excessive algal growth, oxygen (O2) consumption as a result of enhanced aerobic organic matter (OM) degradation, the development of hypoxic and ultimately anoxic (or sulfidic) conditions in hypolimnetic waters, and possibly fish kills and biodiversity loss (e.g., Vitousek et al., 1997; Carpenter et al., 1998; Smith, 2003). Eutrophication-driven anoxia in the hypolimnion can be either a seasonal or permanent phenomenon, depending on water-column dynamics and stratification regimes. Meromictic lakes display permanent temperature- and/or solute-driven density stratification, where anoxia in the monimolimnion (i.e., the permanently stratified portion of the hypolimnion) can prevail for decades, and holomixis is only induced during extremely cold winters with exceptionally strong storm events (Boehrer and Schultze, 2008; Holzner et al., 2009). Deep fjord-like lakes with a low surface-to-volume ratio are particularly prone to the development of meromixis and permanent anoxia.
The mixing regime of a lake, through its influence on the redox state, can have profound indirect effects on the phosphorous (P) retention in lacustrine sediments, and hence on the trophic state of the overlying water column (e.g., Boström et al., 1982). The classical view is that P is retained effectively in the sediments if the hypolimnion is oxic, whereas P is released at the onset of sediment anoxia (e.g., Einsele, 1936; Mortimer, 1942). P retention is attributed to the redox-controlled formation of microlayers of Fe (and Mn) oxyhydroxides at the sediment-water interface and the strong adsorption of dissolved PO. The long-standing paradigm predicts that at concentrations below the O2 threshold for hypoxia (62.5 μmol L−1) in the overlying water column, Fe and Mn oxyhydroxides are reductively dissolved, and the previously bound P can diffuse into the water column (Mortimer, 1942), contributing to the lake-internal eutrophication. Many empiric investigations seem to support the P-vs.-redox paradigm (e.g., Prepas and Burke, 1997; review by Beutel and Horne, 1999), yet multiple other studies cast doubt on its general validity (Katsev et al., 2006; Moosmann et al., 2006; Hupfer and Lewandowski, 2008). For example, over longer timescales, aeration had no effect on the P content of two eutrophic lakes in Switzerland (Gächter and Wehrli, 1998; Gächter and Müller, 2003). The causal links between hypolimnetic O2 content, sedimentary P retention capacity, and internal P loading are still uncertain, as is the question regarding the reversibility of these links.
Anaerobic modes of microbial nitrate (NO) and nitrite (NO) reduction, such as denitrification (organotrophic or lithotrophic) or anammox, found in anoxic lake water and sediments are particularly important filters for anthropogenically fixed nitrogen (N) before it reaches the ocean (Galloway et al., 2003; Seitzinger et al., 2006). Although it is clear that NOx reduction to N2 and N2O is the ultimate sink for fixed N in freshwater systems (review in Seitzinger et al., 2006), the factors that control the rate of N loss, the active modes of denitrification under specific environmental conditions, and the impacts of environmental change on the rate of fixed N loss are not completely understood. For example, the impact of changes in ventilation rates and thus redox conditions on NO reduction in lakes is unclear. While reducing the O2 concentration can expand the volume of anoxic water where pelagic denitrification can occur (e.g., Jensen et al., 1994), the development of an anoxic buffer zone in the water column may reduce diffusive nitrate transport into the sediments and thus benthic denitrification rates in low-NO lakes. In contrast, increasing O2 concentrations (e.g., in artificially aerated lakes) may expand the oxic/suboxic zone where nitrification can supply NO and NO to denitrification, enhancing N loss. Seasonal cycles of water-column mixing and stagnation, and episodic mixing events influence the oxidation state of surface sediments and modulate the penetration of redox boundaries into the sediments, changing the redox conditions and substrate availability for nitrifiers and denitrifiers (Rasmussen and Jørgensen, 1992). Fluctuations of dissolved O2, particularly at low levels, probably have a substantial impact on the balance between the different reaction steps in nitrification, denitrification, and nitrifier-denitrification, regulating overall fixed N elimination rates, as well as the potential accumulation of intermediates such as nitric oxide (NO) and nitrous oxide (N2O) (McCrackin and Elser, 2010; Schreiber et al., 2012, 2014; Freymond et al., 2013).
Anaerobic microbial degradation of OM in sediments and the suboxic/anoxic depths of meromictic lakes is a source of reduced compounds, such as ammonium (NH), manganese (Mn2+), ferrous iron (Fe2+), hydrogen sulfide (H2S), and methane (CH4). CH4, which is produced by microbial methanogenesis in most lakes, is a trace gas with a high global warming potential, exceeding that of carbon dioxide (CO2) by more than 20-fold (Manne and Richels, 2001). Large amounts of CH4 can be stored within the anoxic hypolimnion of stratified lakes, where turbulent vertical mixing is strongly reduced, and the exchange of dissolved substances between the deep and surface waters is inhibited (e.g., Schmid et al., 2005; Crowe et al., 2011; Blees et al., 2014a). Up to 16% of natural CH4 emissions may originate from lakes (Bastviken et al., 2004), and the cyclic or episodic release of hypolimnetic CH4 to the atmosphere during water-column overturn events (i.e., storage flux) may represent one of the main emission mechanisms in many lakes (e.g., Schubert et al., 2012). Similarly, other OM degradation products, including toxic compounds like NH3 and H2S, which have accumulated over many years or decades in the deep portions of permanently stratified lakes, can have detrimental effects on aquatic biota when mixed into the epilimnion (Beutel, 2001; Duval and Ludlam, 2001; Katsev et al., 2010), and represent an imminent potential sink for O2.
The North Basin of Lake Lugano is a deep (max. depth 288 m) glacial lake located on the border between Switzerland and Italy (46°00′N, 9°00′E, 271 m above sea level) (Figure 1). In contrast to the southern basin of the lake, the North Basin was meromictic for several decades, with a quasi-permanent chemocline at ~100 m water depth. The stagnant, anoxic waters below (Wuest et al., 1992; Aeschbach-Hertig et al., 2007) were laden with high concentrations of CH4 derived from benthic methanogenesis (Liu et al., 1996). The chemical stratification was the result of eutrophication since the 1950s, with whole-basin phosphorous (P) concentrations up to 5 μmol L−1 in the early 1980s (Barbieri and Simona, 2001). Lake restoration measures to reduce the P and N loadings led to an improved water quality in the upper 100 m of the water column (i.e., the mixolimnion) (Barbieri and Simona, 2001), but high P and N concentrations and anoxia continued to prevail at greater depths [Commissione internazionale per la protezione delle acque Italo-Svizzere, (CIPAIS), 2005, 2006]. Two consecutive cold and windy winters in 2005 and 2006 destabilized the stratified water column to the point that exceptionally strong mixing was induced (holomixis in March 2005 and March 2006). The resulting deep-water renewal, as manifested in water-column profiles of environmental tracers (3H, 3He, SF6, and CFCs) that were measured shortly after the mixing events (Holzner et al., 2009) has been the only ventilation of the lake's deep anoxic hypolimnion dating back to the 1960's. Since then, the North Basin's water column has stabilized, and anoxia has been re-established (e.g., Blees et al., 2014a). However, the density stratification has been weakened with respect to the pre-overturn period (Holzner et al., 2009) and the chemocline is now situated at ~135 m (Wenk et al., 2013; Blees et al., 2014a).
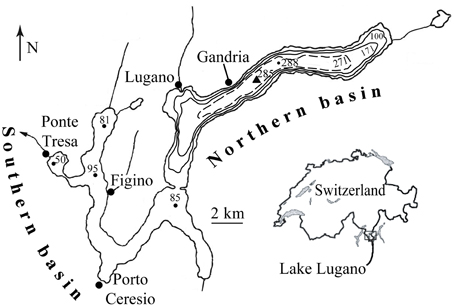
Figure 1. Map of Lake Lugano. The black triangle indicates the sampling station near the deepest part of the northern basin. Small dots refer to water depths in meters.
Here we investigate the short- and mid-term impacts of the exceptional mixing events in 2005/2006 on the lake's redox balance, as well as on the inventories of P, fixed N, CH4, and other elements. Although the change in the lake's oxygenation status was ephemeral, it had a profound effect on the internal P load and on redox-sensitive metabolic pathways and fluxes involving fixed N species and CH4. We will (1) discuss the possible mechanisms behind observed nutrient inventory changes and the short-term impacts of their internal redistribution on primary production, (2) demonstrate the effect of episodic deep-water renewal on the ecosystem-scale fixed-N elimination, and (3) address the consequences of exceptional mixing events for lacustrine CH4 oxidation and emission to the atmosphere. We will also examine the temporal context regarding the re-establishment of steady-state conditions, i.e., the potential return to homeostasis in the years after exceptional mixing events.
Materials and Methods
As part of a long-term monitoring campaign by the Commissione Internazionale per la Protezione delle Acque Italiano-Swizzere (CIPAIS), the Office for water protection and purification (UPDA; formerly Laboratorio Studi Ambientali, LSA) and the Scuola Universitaria Proffessionale delle Svizzera Italiana (SUPSI), water samples and multi-parameter CTD probe (Idronaut Ocean Seven 316 Plus) profiles (conductivity, temperature, pH, dissolved O2) were collected monthly at a station near the deepest part of the lake, close to the village of Gandria (Figure 1). Water samples were taken using 5-L Niskin bottles at 16 different depths, and sample aliquots for hydrochemical analyses were filtered (0.45 μm), stored in acid-washed 60-mL HDPE bottles, and analyzed within 24 h. CTD-derived O2 concentrations were calibrated against Winkler titration measurements. The concentrations of PO, NOx (NO + NO), NH, and H2S (i.e., ∑H2S, HS−, S2−) were determined with an autoanalyzer (TRAACS 2000; Bran and Lübbe) or with a spectrophotometer using standard colorimetric methods [American Public Health Association, (APHA), 1989]. Total dissolved N was analyzed using the persulfate oxidation method (Solorzano and Sharp, 1980), followed by colorimetric NO analysis. For the measurements of total dissolved Fe and Mn (i.e., Fe2+, Mn2+), filtered samples were immediately acidified (HNO3) and analyzed by ICP-MS (Agilent Series, 7700x). For CH4 concentration measurements, water samples (500 ml) were amended with sodium hydroxide (5 ml, 50%) in order to stop microbial activity and to expel dissolved CH4 into the headspace. Headspace [CH4] was determined using a gas chromatograph (Perkin Elmer, model 3920) with a 0. 25 mm fused silica column (Rxi® Guard/Retention Gap Column, Restek) and a flame ionization detector (FID). Finally, gross primary production (GPP) was measured by 14C-bicarbonate assimilation using the Steemann-Nielsen (1952) method, modified by Gächter and Marès (1979). Daily primary productivity was calculated according to Gächter (1972) by interpolation from short-interval, depth-integrating (0–20 m) measurements, taking into account diurnal solar radiation variability.
Concentration profiles at the sampling station were used to calculate the inventories of O2, PO, fixed N (NH, NO), Mn2+, Fe2+, H2S, and CH4 for the whole North Basin (0–288 m), and for specific water-column segments (e.g., 0–20; 20–100; 100–288 m) below and above the chemocline. Depth-integrated total solute contents were calculated according to Bührer (1979), taking into consideration the bathymetry of the lake basin and assuming linear concentration changes between sampled depths and horizontal uniformity. The total contents of dissolved O2 and reduced diagenetic by-products (NH, Mn2+, Fe2+, H2S, and CH4) were used to calculate redox balances, assessed as the respective difference between the total amount of O2 and the sum of reducing equivalents (not considering organic matter), expressed in metric tons (t) O2. The following stoichimetries were used (Van Cappellen and Wang, 1996):
Results
Hydrochemical Profiles—Seasonal Dynamics and Biogeochemical Processes
The seasonal dynamics in the surface and subsurface waters were qualitatively identical for the period before and after the de-stratification events in 2005/2006. Exemplary data from the year 2003 show the typical spatial and temporal solute concentration changes that highlight the importance of processes of main interest for this study (Figure 2). Thermal stratification (Figure 2A) during summer months limits the supply of O2 to water depths below until winter mixing of the upper water column occurs. Hypolimnetic O2 concentrations systematically decrease with depth and reach concentrations < 1 μmol L−1 at 100 ± 20 m (Figure 2B). The annual variation of the redox transition zone by several meters is modulated by the seasonal cycles of photosynthesis, respiration, and meromixis. That is, a shallower redoxcline is observed during late summer and fall, whereas in winter the oxygenated zone expands after partial water-column overturn and the injection of O2-laden water into mid depths. PO and NO concentrations in surface waters decrease to zero-levels during the productive season (Figures 2C,D). Below the photic zone, PO continuously increases to ~10 μmol L−1 in near-bottom waters. NO concentrations generally decrease from 30 to 40 μmol L−1 in subsurface waters to undetectable levels within the chemocline (Figure 2D) indicating active denitrification in suboxic waters within the redox transition zone. In previous work (Wenk et al., 2013), we have shown that NO reduction in the water column is largely driven by sulfide-dependent denitrifying bacteria. The highest NH concentrations (60–80 μmol L−1) were always measured in near-bottom waters (Figure 2E). The decline in NH concentration from the bottom of the lake basin to zero-levels (i.e., < 1 μmol L−1) in mid-depth waters is mainly due to aerobic NH oxidation near the redoxcline, but there is also molecular and geochemical evidence for anaerobic NH oxidation (anammox) below the chemocline (Wenk et al., 2013). The deep hypolimnion is sulfidic, with maximum H2S concentrations up to 18 μmol L−1 close to the lake bottom (Figure 2F). Similarly, maximum Fe2+ and Mn2+ accumulation is observed in near-bottom waters, with concentrations of up to 15 and 12 μmol L−1, respectively (data not shown). CH4 concentrations in the deepest water masses ranged between 110 and 190 μmol L−1 (Figure 2G), indicating CH4 production within the lacustrine sediments, and its diffusive efflux to the water column (Blees et al., 2014a). CH4 concentrations generally decreased to low nanomolar levels at, or even below, the chemocline, indicating its quantitative consumption by methanotrophic microorganisms within/near the redox transition zone (Blees et al., 2014a). Recent biomarker, phylogenetic and CH4 turnover rate measurements by Blees et al. (2014a) imply that CH4 consumption in the North Basin is driven by (micro-) aerobic CH4 oxidation (MOx), without evidence for an anaerobic mode of CH4 degradation. A strong bacterial MOx potential was measured throughout the anoxic water column (Blees et al., 2014a). This potential normally remains unexploited under in situ conditions due to lack of dissolved O2.
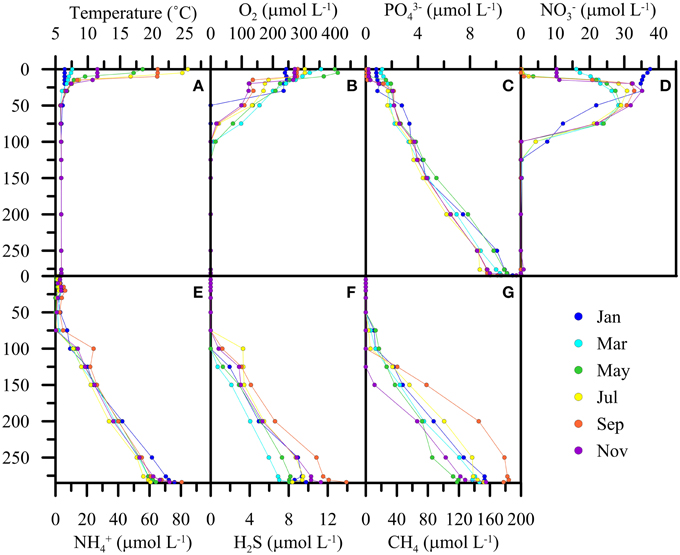
Figure 2. Exemplary bi-monthly water-column profiles of temperature (A) and solute concentrations (B–G) during the seasonal cycle 2003.
Physical Parameters and Solute Concentrations during Mixing Events 2005/2006
The mixing events during the winters of 2005 and 2006 were part of a longer water-column destabilization trend associated with the reduction of density stratification due to a gradual deep-water temperature increase by geothermal heating (Aeschbach-Hertig et al., 2007; Holzner et al., 2009). A detailed description of the water-column stability changes that ultimately led to holomixis, is provided by Holzner et al. (2009). Briefly, marked changes in hypolimnetic water temperature and electrical conductivity (i.e., ion content) were observed during the winter of 2005 (Figure 3). The particularly strong surface water cooling in January/February 2005 reduced the vertical density gradient to the point where convective mixing, first of the upper (by 1 March), then of the complete water column (by 15 March), was induced. Deep mixing was indicated by a 0.3°C temperature decrease in the near-bottom water and the full disintegration of the chemocline (i.e., the loss of electrical conductivity and solute gradients) (Figures 3A,B, 4). After the mixing event, electrical conductivity in the hypolimnion was virtually constant throughout the water column (~238 μS cm−1). Deep-water renewal was also indicated by the occurrence of traces of O2 at the bottom of the lake (Figure 3C). The gradual water-column destabilization since 1995 (Holzner et al., 2009) (i.e., reduction of midwater density gradients) culminated in an even stronger mixing event in February 2006 (data not shown). The second holomixis event lasted for about 60 days (Holzner et al., 2009), and was indicated by an additional cooling of deep waters by 0.3°C. Water-column homogenization in February 2006 led to the oxygenation of the complete hypolimnion with O2 concentrations between 40 and 60 μmol L−1, and a marked drop in surface water [O2]. Elevated oxygen levels in the deep hypolimnion were sustained until autumn, when the near-bottom waters turned anoxic again, and stayed anoxic until today (2015; CIPAIS unpublished data).
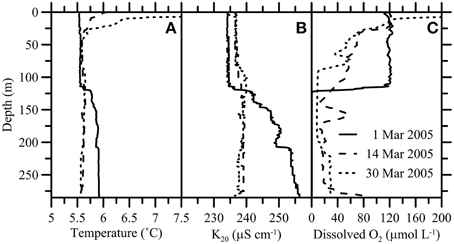
Figure 3. Hydrocast sensor profiles of (A) temperature, (B) conductivity (K20), and (C) dissolved O2 concentrations during the first mixing event in March 2005. Changes in hypolimnetic water temperature and electrical conductivity indicate convective mixing of the complete water column in March 2005. Deep-water renewal is also indicated by the occurrence of traces of O2 at the bottom of the lake.
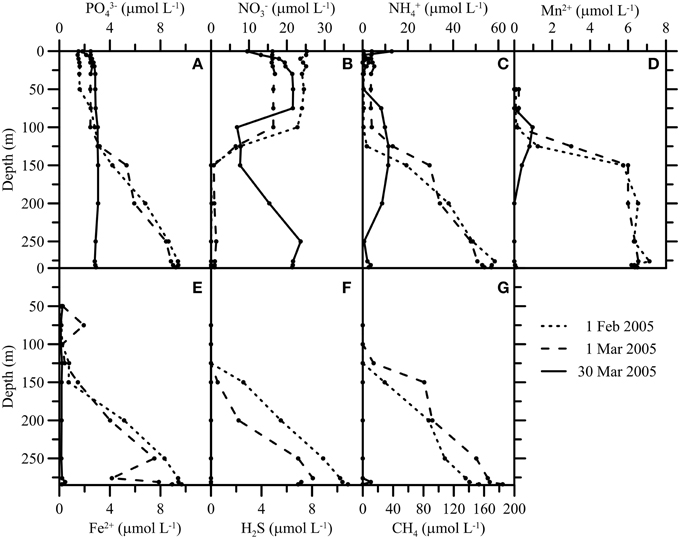
Figure 4. Hydrochemical profiles (PO, NO, NH, Mn2+, Fe2+, H2S, CH4) (A–G) before (1 February and 1 March) and after (30 March) the mixing event in 2005. The mixing had a strong impact on the biogeochemistry of the water column, redistributing nutrients and leading to the disappearance of large amounts of reduced solutes.
With the exceptional first water-column overturning period and ventilation of the deep waters in March 2005, hypolimnetic PO, NO, and NH gradients collapsed (Figures 4A–C). Water from the upper water column that was relatively PO and NH-depleted, and NO rich, mixed with water from the deep anoxic zone that was relatively NH- and PO-rich, and NO free. This caused a decrease in the NO concentration of the oxic zone above 100 m and an increase in the NO concentration of the water layers below. The opposite shifts were observed for NH and PO. The internal redistribution of PO led to a total P-concentration in the photic zone above 3.5 μmol L−1 (see next section). With the second holomixis event in February 2006, fixed N was evenly distributed throughout the water column. Elevated NO concentrations in the deep hypolimnion of the North Basin prevailed until 2008, and NH levels remained relatively low compared to the pre-ventilation period until today (data not shown). In 2005, the CH4 concentration at 285 m depth decreased from >180 μmol L−1 on 1 March to nanomolar-levels on 30 March (Figure 4G), so that within 1 month, nearly all CH4 had disappeared from the water column. H2S, Fe2+, and Mn2+ concentrations displayed qualitatively similar changes (Figures 4D–F).
Surface Water Nutrient State and Primary Production
For a short period of time, the mixing events caused elevated PO4-P concentrations in the upper water column (0–20 m), approximately doubling the average P availability in the photic zone during the productive season in 2006 before dropping to “normal” (i.e., pre-mixing) values thereafter (Figure 5). The surface water NO pool seemed less susceptible to the water-column overturn events in 2005 and 2006. The NO content may have decreased slightly between 2004 and 2007, but increased again to premixing-values in the subsequent years (Figure 5). The long-term average primary production data did not reveal any significant perturbation of lake productivity in the aftermath of the lake overturn events. That is, neither the annual mean primary production nor the maximum carbon assimilation rate during the spring blooms significantly increased in 2005 and 2006, in spite of enhanced P availability in the surface waters (Figure 5). Similarly, the intra- and inter-annual variability of chlorophyll a (Chl a) in the photic zone was equivalent before and after the mixing events, without any detectable Chl a anomaly during the spring blooms of 2005 and 2006 (data not shown).
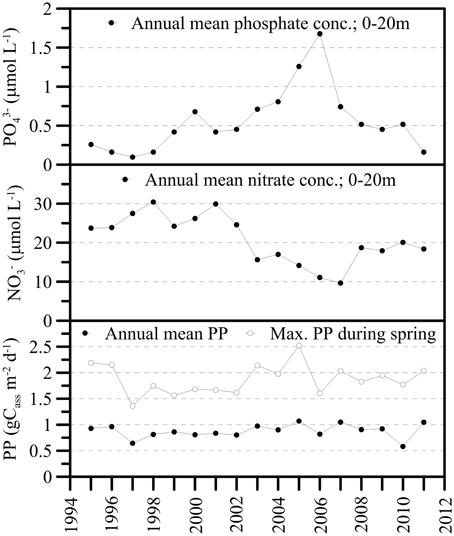
Figure 5. Annual mean nutrient concentrations (PO4-P, NO-N) in the upper water column (0–20 m) and long-term trends for gross primary production (GPP in g C assimilated per m2 per day). GPP data are given as annual mean values and maximum C assimilation rates during the spring bloom. In association with the holomixis events in 2005 and 2006, and the internal redistribution of nutrients, photic zone PO4-P concentrations increased strongly. NO concentrations decreased slightly between 2004 and 2007. The primary production data did not reveal any significant perturbation of lake productivity in immediate response to the changed nutrient availability in surface water.
Redox Balance and Elemental Inventories before and after the Mixing Events
During February and March 2005, the total O2 content in the North Basin water column decreased by about 6400 t O2 (12700 t O2 on 1 February, 6300 t O2 on 30 March; Table 1). However, by the year after the 2006 ventilation, the mean annual O2 content (>11500 t) had returned to levels that were typically observed before the holomixis events (1997–2004 average: 11365 t O2), suggesting rapid O2 recharge by atmospheric gas exchange. By 2008, the typical distribution of O2 in the North Basin's water column as observed before 2005 was restored again, with O2 concentrations ≥ atmospheric equilibrium levels in the surface and subsurface waters, a distinctive redoxcline at 120–150 m water depth, and permanently anoxic waters below. The average post-ventilation, whole-basin O2 content between 2007 and 2013 was approximately 13000 t O2 (Figure 6). Whereas, the change in the whole lake's oxygen content was ephemeral, the water-column mixing events had a profound effect on the redox balance. Figure 6 displays the long-term evolution of the redox balance in the North Basin. For the two decades between 1986 and 2006, it was negative, with a minimum of <-15000 t O2 equivalents at the beginning of this millennium. Between 2004 and 2008, the redox balance of the North Basin turned positive. This dramatic change in the lake's redox balance directly reflects the vanishing of large portions of reduced solutes in the hypolimnion (see below) in association with the ventilation of the deep hypolimnion during the winters of 2005 and 2006 (data for March 2005 in Table 2). Since then the overall redox-balance remained positive (>11000 t O2 equivalents; Figure 6).
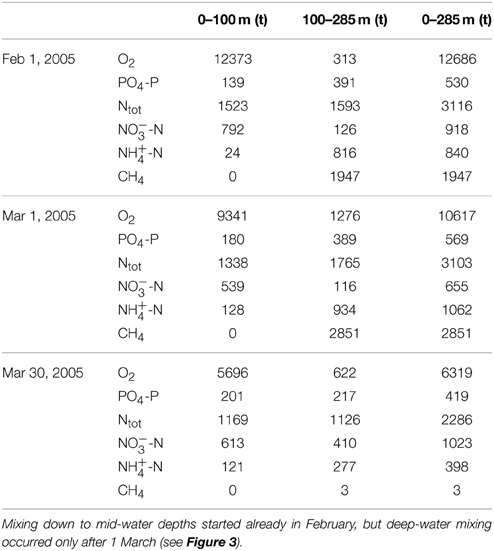
Table 1. Compartmental and whole-basin changes of the O2, PO-P, fixed N, and CH4 inventories (in metric tons, t) in the northern basin of Lake Lugano, associated with the destabilization of the water column during winter 2005.
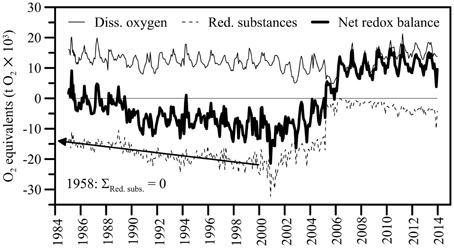
Figure 6. Long-term evolution of redox balance in the northern basin of Lake Lugano (0–284 m). O2 equivalents of the sum of reduced solutes (i.e., NH, Mn2+, Fe2+, H2S and CH4,) were calculated according to Van Cappellen and Wang (1996). Organic matter is not considered. The net redox balance represents the difference between the total amount of O2 and the sum of reducing equivalents, expressed in metric tons (t) O2. The annual variation of the whole-lake O2 and redox budgets follows the seasonal cycles of photosynthesis, respiration, and meromixis (i.e., lowered redox conditions during late summer and fall, high O2 content after partial water-column overturn and the injection of O2-laden water into mid depths in winter). Prior to the early 2000s, reduced substances accumulated at a relatively constant rate of 510 t O2 equivalents per year. Extrapolation of the long-term trend into the past indicates that the accumulation of reduced inorganic compounds in the water column started in 1958, marking the beginning of partial anoxia in the Lake Lugano North Basin.
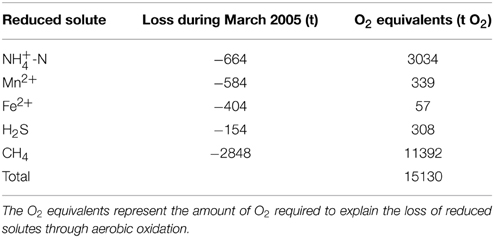
Table 2. Quantities of reduced substances that disappeared from the monimolimnion during lake overturn between 1 and 30 March.
A general declining trend in the total P-content from before the ventilation period (a reduction from ~680 t PO4-P in 1998 to ~550 t PO4-P in 2004) seems to have been enhanced by the two mixing events (an additional reduction by ~200 t PO4-P until 2007) (Figure 7A). During the most recent years, the total P-content has stabilized around 300 t PO4-P. Before 2005, total NO and NH contents varied between 700 t and 1000 t NO-N, and 1100 t and 1500 t NH-N, respectively. The longer-term annual means (1995–2004) were 880 t NO-N and 1320 t NH-N (Figures 7B,C). The annual average total fixed N content before 2005 (i.e., 1995–2004) steadily increased from ~3000 t N in 1995 to ~3800 t N in 2004, and the decadal mean was 3400 t N (Figure 7D). With the lake overturn in February/March 2005, NO appeared to be internally redistributed, with a distinct increase of NO in the monimolimnion (ΔN100−284m: ~+280 t NO-N), and a decrease in the upper water column (ΔN0−100m: ~-180 t NO-N) (Table 1; Figure 7B). However, the longer-term average whole-basin NO content after the holomixis events was essentially the same as before (2005–2013: 860 t NO-N). Total N declined from 3460 t N in 2004 to 2240 t N in 2006 (Figure 7D), a reduction that can almost entirely be attributed to the loss of NH-N (~1000 t; Figure 7C). Since 2006, the hypolimnetic NH-N inventory increased steadily at a rate of 40 t NH-N per year and reached ~400 t in 2013. Similarly, before the holomixis events in 2005/2006, the longer-term mean CH4 content in the North Basin was ~3000 t CH4 (1995–2004). With the holomixis in March 2005, almost all hypolimnetic CH4 was removed. Since 2006, the total annual mean CH4 inventory increased from ~140 t CH4 to ~600 t CH4 in 2013, corresponding to an average net production of 66 t CH4 per year.
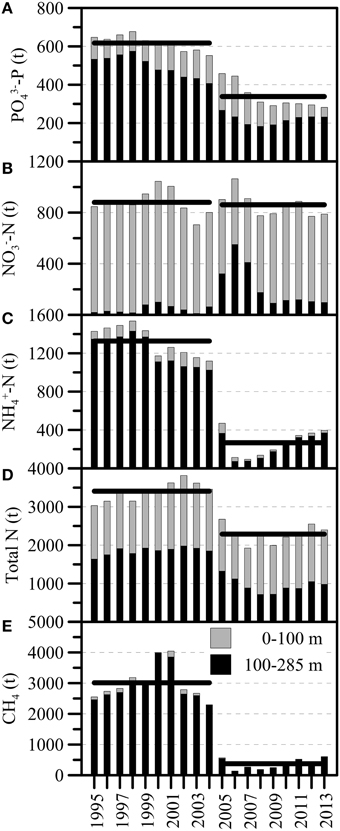
Figure 7. Annual average inventories (in metric tons) for (A) PO-P, (B) NO-N, (C) NH-N, (D) total dissolved fixed N, and (E) CH4 between 1995 and 2013 (light: 0–100 m and dark: 100–284 m; 100 m marks the long-term average (pre-holomixis) water depth of the chemocline/redoxcline). The first of two distinct whole-water-column mixing events occurred in March 2005. Horizontal bars indicate the multi-annual means for the pre- and post-mixing periods, 1997–2004 and 2005–2013, respectively.
Discussion
Perturbation of the Redox State and Reduction of the Hypolimnetic Oxygen Demand
For several decades, a persistent redoxcline has separated aerobic processes (aerobic OM degradation, nitrification) in the upper water column from anaerobic processes (anaerobic microbial OM degradation, denitrification) below the chemocline and in the sediments. Large amounts of reduced substances accumulated in the anoxic hypolimnion at a relatively constant rate of 510 t O2 equivalents per year (Figure 6; corresponding to an aerial reduced-compound efflux of 1.6 mmol O2 equivalents m−2 d−1), and increased the basin-wide O2 demand. First evidence for low oxygenation of the deep water column of the North Basin was already provided in Baldi et al. (1949). Based on the observed long-term trend, we can back-calculate the timing of the last shift from oxic to anoxic conditions in the deep hypolimnion. Our extrapolation indicates that the accumulation of reduced inorganic compounds in the water column started in 1958, marking the beginning of partial permanent anoxia (and meromixis) in the Lake Lugano North Basin (Figure 6). This estimate agrees well with Vollenweider (1965), who for the first time confirmed full anoxia of the water column below 150 m water depth in 1960.
For the first time in almost five decades, the March 2005 deep mixing event led to the homogenization of the entire water column, advecting O2 and NO down to the bottom of the basin. To date, the 2005 and 2006 oxygenation/nitratation events in the deep hypolimnion stand out as profound disturbances to the biogeochemical cycling and element budgets in the North Basin. The stark shift in the overall redox balance from strongly negative values before March 2005 to a positive balance in 2006 suggests that, with the two holomixis events, large amounts of O2 were transferred from the atmosphere into the lake. However, the O2 was primarily used up in the microbial or chemical oxidation of reduced compounds, so that in spite of an overall more positive redox balance, the mixing (i.e., the exposure of reduced solutes to oxic water masses) intermittently led to the decline of the lake-wide O2 content. The O2 intrusion into NH-laden deep waters induced the oxidation of much of the NH to NO by nitrifying bacteria, thus explaining the approximately antipodal shifts in the lake's NH (decrease) vs. NO (increase) contents that were associated with holomixis (Figures 4B,C). Similarly, the complete disappearance of CH4 in the hypolimnion after the 2005 mixing event (Figure 4G) can be partly attributed to aerobic oxidation by methanotrophic bacteria (see below). In qualitative terms, these hydrochemical changes are akin to those observed during annual ventilation of holomictic eutrophic lakes with a seasonal chemocline (e.g., the Lake Lugano South Basin; Lehmann et al., 2004; Blees et al., 2014b), with the obvious difference that lower frequency episodic/multi-decadal de-stratification events result in greater and more prolonged perturbation of the lake biogeochemistry. In total, the holomixis events in 2005/2006 have caused more than 2800 t of CH4, more than 1200 t of reduced N, and substantial amounts of H2S and reduced metals to be removed from the North Basin (see data for 2005 in Tables 1, 2). Soon after the ventilation, the hypolimnetic O2 depletion in the North Basin was restored again. The inventory of reduced substances, however, remained relatively low (< −4000 t O2 equivalents on average in 2013), and the whole-basin's redox balance remained positive until today (Figure 6). Thus, while the exceptional mixing events provided only temporary relief from long-term water-column anoxia, they have strongly and sustainably reduced the overall hypolimnetic O2 demand, potentially facilitating ongoing and future measures to mitigate O2 depletion in the lake basin.
Effects on Internal Phosphorous Loading and Primary Production
The first deep mixing event in March 2005 caused a reduction of the total PO-P content in the North Basin by 150 t P (Figures 4A, 7A; Table 1). The aeration of the monimolimnion seemed to have at least temporarily accelerated the general declining trend in P content that was observed since the 1980s. That is, the average basin-scale P reduction in the years before 2005 was ~17 t P y−1, whereas it was ~60 t P y−1 between 2004 and 2007. The rapid decline of the total P content in the North Basin between 2004 and 2007 was thus not solely the result of the still on-going reduction of external P loading, but appears to be directly linked to changes in the lake-internal P-cycle, triggered by the transient aeration of the deep hypolimnion. This observation seems to support the long-standing paradigm, predicting that upon oxygenation of a lake water column, P from organic matter remineralisation is effectively retained, or trapped, in the sediments by adsorption to Fe(III) and Mn(IV) oxyhydroxide particles (Mortimer, 1942). Supporting this paradigm, several studies have demonstrated the short-term reduction of hypolimnetic P concentrations after natural or artificial ventilation of lakes (Ashley, 1983; Prepas and Burke, 1997). However, over longer timescales, oxygenation of the hypolimnion of eutrophic lakes in Switzerland did not seem to effect the water-column P content (Gächter and Wehrli, 1998; Gächter and Müller, 2003), suggesting that the ultimate benthic P flux does not depend solely on the hypolimnetic O2 concentration, but rather on the balance between the gross sedimentation of organic matter, the mineral composition of the sediment and the coupled S, Fe, and P cycling in the sediment (Gächter and Müller, 2003; Slomp et al., 2013). Therefore, there may not be a causal relationship between hypolimnetic anoxia and enhanced P release from lake sediments. Rather, these are concomitant side-effects of excessive organic matter and P sedimentation, and remineralization in eutrophic lakes (Gächter and Wehrli, 1998).
To some extent, the oxygenation of the monimolimnion and the formation of a Fe(III) and Mn(IV) oxyhydroxide layer at the sediment water interface, functioning as reactive-P barrier, may indeed have transiently suppressed the release of P from the sediments. However, the capacity of such a P sink is questionable, particularly in light of the fact that even if O2 is present in the overlying water, O2 penetration in a productive lake such as Lake Lugano may barely exceed 1–2 mm. We argue that rather than a lowered, or stalled, benthic P efflux, it was the efficient scavenging of water-column-borne P and its transfer to the sediments that contributed most to the enhanced P decline. Deep-water ventilation during winter 2005 (and to a lesser extent in 2006) led to the oxidation of Fe2+ and Mn2+, and the sorption of P onto settling Fe(III) and Mn(IV) oxyhydroxide particles. In March 2005 alone, ~350 t of dissolved Fe2+ and 580 t of Mn2+ were oxidized (Table 2), generating ample capacities for PO adsorption. However, shortly after the mixing events, the deeper waters turned anoxic again, so that the sedimented metal oxyhydroxides were reductively dissolved, and the previously adsorbed P was released again to the hypolimnion. The repositioning (i.e., deepening) of the chemocline has expanded the oxic sediment surface area that acts as P sink. Nevertheless, although the mixing-induced oxidative scavenging and burial of PO transiently removed P from the water column, this probably had no lasting effect on the overall P load in the lake basin, because the P-binding capacity of the sediments below ~135 m was temporary. Indeed, after 2007, the hypolimnetic PO content slowly increased again (Figure 7A). The decreasing P trend as observed before 2005, and predicted as a delayed response to the reduction in P load since the 1970s, now seems to be buffered by the slow reflux of Fe(III) and Mn(IV) oxyhydroxide-bound P from the sediments after return to anoxic conditions in the near-bottom waters. This observation confirms that oxygenation of hypolimnetic waters cannot (at least on a short-term) change P diagenesis in a way that the level of P deposition is permanently increased. Thus, it is unlikely to have a lasting effect on the overall P trophic state of a lake.
While the overall P load in the North Basin was substantially reduced as a result of adsorptive trapping and sedimentation of P, internal redistribution led to elevated PO4-P concentrations in the upper water column (0–20 m), temporarily doubling the average P availability in the photic zone during the productive season (Figure 5). During overturn in both 2005 and 2006, photic zone PO4-P concentrations transiently reached the threshold of 100 μg P L−1 (~3.2 μmol L−1), which was last exceeded in the early 1980's, shortly after the eutrophication peak (Barbieri and Mosello, 1992). The temporary internal P eutrophication and increase in trophic state did not appear to detectably stimulate lake productivity and biomass production, either in the short- or mid-term. That is, there was neither a substantial change in the average annual primary productivity, nor in peak primary production during the spring blooms in 2005 and 2006 (Figure 5). The question as to what primarily controls lacustrine eutrophication, P or fixed N, is long-standing. The paradigm of lakes being primarily P-limited (e.g., Schindler, 1974, 1977; Carpenter et al., 1992) seems to be contradicted by a large amount of observational and experimental data (see compilation in Sterner, 2008), indicating that lakes are co-limited by N and P (and possibly other elements/resources as well). While there are good arguments for whole-lake P limitation on longer time scales (Schindler, 1977), the primacy of P limitation on time scales relevant to this study is clearly not supported by our data. The fact that the transiently elevated P concentrations in the surface waters of the Lake Lugano North Basin (in the absence of any concomitant increase in fixed N) did not exacerbate eutrophication, suggests co-limitation of primary production in Lake Lugano by multiple nutrients rather than regulation by P alone, at least on a month-to-month time scale.
More Oxygen – More Denitrification
Both denitrification and anammox are believed to occur only in environments with O2 concentrations < 25 μmol L−1 (Rysgaard et al., 1994; Codispoti et al., 2005; Kalvelage et al., 2011). As for Lake Lugano, our previous work suggests that this threshold is even lower, given that denitrification was observed only at [O2] < 10 μmol L−1 (Wenk et al., 2013). Before the first mixing event in March 2005, the potential water-column denitrification/anammox zone (i.e., [O2] < 10 μmol L−1) extended from the lake floor to the chemocline at ~130 ± 10 m water depth (Figures 2, 3). However, pelagic NOx reduction was most likely limited to the suboxic portion of the redox-transition zone, where NO was still replete and/or NO could be supplied by microaerobic nitrification (Wenk et al., 2013). It is reasonable to assume that water-column denitrification (and anammox) was fully inhibited as soon as O2 was mixed into the deep waters during the first de-stratification event in March 2005, temporarily elevating near-bottom O2 concentrations to >50 μmol L−1. In the absence of suboxic N elimination by either denitrification or anammox, the hypolimnetic fixed N content should have increased after holomixis, but the opposite was observed: within less than 1 month more than 800 t of fixed N (approximately commensurate with the annual mean external N load to the North Basin) were eliminated from the hypolimnetic water column (Figure 7; Table 1). The decrease of the NH-N and the concomitant increase of the hypolimnetic NO-N pool suggests that large amounts of NH were oxidized to NO through nitrification (yet abiotic oxidation of at least some of the NH cannot be excluded). The fact that the NO-N content did not increase as would be expected if the NH-N was converted stoichiometrically to NO by nitrification, and that the overall NO-N pool did not decline much, indicates that large amounts of the newly regenerated NO were readily denitrified, in spite of oxic conditions in the hypolimnion. Apparently, rather than hampering ecosystem fixed N elimination by inhibiting water-column denitrification/anammox, the oxygenation of the deep hypolimnion enhanced the NOx availability and thus stimulated denitrification wherever it still occurred. We argue that, while water-column denitrification was prevented by inhibitory O2 levels, denitrification and coupled nitrification-denitrification proceeded at the sediment-water interface. Nitratation of the previously NO-free monimolimnion (by oxidation of the NH, and also by mixing with NO-rich mixolimnion water masses) brought NO in contact with sediments that had a strong denitrifying potential but were NO-starved prior to lake mixing, thus invigorating benthic denitrification by enhancing the NO flux into the anoxic sediments. For several decades, benthic denitrification in the Lake Lugano North Basin was restricted to the sediment surface area above the permanent redoxcline (< 10 km2), leaving a large part of the ecosystem fixed-N elimination potential unused. With holomixis in March 2005, there was a period during which benthic denitrification took place over the entire sediment area of the lake basin (>27 km2). When the volume of NO-laden water increased, the basin-wide NO elimination rate also increased. After the lake overturn, at least temporarily, the benthic NO reduction was highly efficient and the basin's denitrifying capacity was more fully exploited. The observed N loss within a short period of time (30 days) translates into a net areal benthic fixed N consumption rate of >3100 μmol m−2 h−1, which is high in comparison to many other lakes (Seitzinger, 1988). However, benthic nitrate reduction rates equal to or greater than this have been reported for other Swiss eutrophic lakes (Lake Zug: 1100 μmol m−2 h−1, Lake Baldegg: 6100 μmol m−2 h−1; Mengis et al., 1997). With the re-establishment of meromixis, permanent anoxia, and NO exhaustion in the monimolimnion, the ecosystem-scale N loss was greatly reduced again, and the total dissolved fixed N inventory of the North Basin stabilized at comparably low levels (Figure 7D).
Impact of Deep-water Ventilation on the Methane Budget
Before overturn in the winter of 2005, aerobic water-column CH4 oxidation was restricted to a few meters in the open water column, at the redoxcline where both CH4 and O2 overlapped. The redox transition zone functioned as an effective CH4 filter so that the aerobic water body contained only trace amounts of CH4 (Liu et al., 1996; Blees et al., 2015), whereas below the redoxcline, CH4 accumulated over the decades to bottom water concentrations up to 190 μmol L−1. With the ventilation of the hypolimnion, essentially all CH4 disappeared from the water column, as indicated by the uniformly low CH4 concentrations (Figure 4). The decline from ~2800 t CH4 to ~3 t CH4 within only 1 month can partly be attributed to aerobic oxidation of CH4 (MOx) upon exposure of the CH4 pool and the MOx bacterial stock to O2–replete waters during water-column mixing. Indeed, Blees et al. (2014a) measured high potential MOx rates of up to 2.5 μmol CH4 L−1 d−1, particularly in the upper monimolimnion (in spite of the apparent absence of O2). Phylogenetic analyses confirmed that aerobic methanotrophic bacteria mainly related to Methylobacter sp. thrive under micro- and anoxic conditions, maintaining a “stand-by mode” throughout the anoxic water column in the Lake Lugano North Basin. When O2 is injected into anoxic hypolimnetic water masses that contain a relatively high abundance of aerobic methanotrophs, the MOx pontential in the hypolimnion is more fully exploited, and at least temporarily high in situ MOx rates can be expected. Nevertheless, the disappearance of the CH4 during March 2005 cannot be fully attributed to MOx. Irrespective of whether the volumetric MOx rates that were needed to explain the total CH4 loss are realistic, the mere aerobic oxidation of the CH4 would have required more O2 than was available. Taking into account also the aerobic oxidation of all the other reduced substances that disappeared from the water column in March 2005, the total O2 requirement would have been >15000 t O2 (Table 2). The actual whole-basin net O2 decline in March 2005 amounted to 4300 t O2 only (Table 1), suggesting that (i) much of the CH4 was oxidized by anaerobic methane oxidation (AOM), that (ii) large amounts of O2 were resupplied from the atmosphere into the lake upon lake mixing, and advected into the deep water column, and/or that (iii) conversely, a large portion of the CH4 was released to the atmosphere by storage flux. AOM can be excluded as important CH4 loss mechanism. In previous work, we could find neither geochemical nor molecular evidence for AOM (Blees et al., 2014a), and it would be difficult to explain why AOM would have been powered up under more oxygenated conditions after deep mixing. More likely, the injection of O2 into hypolimnetic waters may have resupplied some of the O2 that was required for the continuing oxidation of the large reservoir of CH4 (and other reduced compounds). Holzner et al. (2009) demonstrated that considerable deep-water renewal and gas exchange with the atmosphere has taken place during the winters of 2005 and 2006. During these mixing events, the renewed water volumes exceeded the volume of the monimolimnion (i.e., the water volume that was permanently stratified before 2005) by a factor of two (2005) and seven (2006), and low-[O2] waters were continuously exposed to the atmosphere. Using gas exchange velocities of 0.32 m d−1 for O2, and the observed O2 concentration and temperature data during mixing, Holzner et al. (2009) calculated a mixing-associated net flux of 80 t O2 d−1 into the lake. Thus, through “solubility pumping” not more than 2400 t O2 could have been injected into the Lake Lugano North Basin between 1 and 30 March, still insufficient, by far, to account for the oxidation of the remaining pool of CH4 in the monimolimnion, and the observed turnaround from a strongly negative to a positive whole-basin redox balance (Figure 6; Table 2). We argue that large amounts of CH4 were lost from the lake basin through storage flux. Unfortunately, the Lake Lugano monitoring program does not include the measurement of CH4 in surface waters, which normally contain only traces of CH4 (Blees et al., 2015). Yet, it is reasonable to assume that deep water renewal not only stimulated MOx by injecting O2 into the monimolimnion, but also led to the exposure of CH4-rich deep water to the atmosphere, which resulted in strongly enhanced pCH4 gradients across the lake surface, and hence the evasion of CH4 from the water column to the atmosphere. Storage flux of CH4 is known from mono- or dimictic lakes during seasonal lake overturn (e.g., Bastviken et al., 2004; Juutinen et al., 2009; Schubert et al., 2012), yet given the long-term accumulation of CH4 in the meromictic North Basin prior to 2005, the CH4 emission rate in March 2005 has likely been significantly higher than in annually overturning lakes. By O2-equivalent mass balance, we calculate that about 2100 t CH4 have been lost from the North Basin to the atmosphere by storage flux within only 30 days. This corresponds to a temporary emission rate of >160 mmol CH4 m−2 d−1. In conclusion, in a single ventilation event during March 2005, ~25% (~700 t CH4) of the total CH4 inventory of Lake Lugano, which had been building up in the deep North Basin over several decades, was eliminated by boosted aerobic CH4 oxidation, while ~75% of the CH4 evaded rapidly to the atmosphere. Hence, the normally efficient microbial water-column CH4 filter in Lake Lugano can be temporarily suspended during episodic de-stratification events, rendering the lake a strong source of CH4.
Synthesis and Conclusions
The mixing events in 2005 and 2006 marked the temporary end of an almost 50-year long period of water-column anoxia in the Lake Lugano North Basin. On multiple time scales, the deep-water ventilation that was associated with the water-column turnover induced a profound disturbance of the element budgets in the lake. The total redox balance, which had been negative for two decades, turned positive with the oxidation of large amounts of reduced substances in the hypolimnion. On a shorter time-scale, the O2 injection into the monimolimnion led to the scavenging of large amounts of PO by metal oxyhydroxides that formed upon oxidation of their reduced counterparts (i.e., Fe2+, Mn2+), and their coupled transfer to the sediments, resulting in a marked, but only temporary, P decline within the water column. The impact on the fixed N and CH4 inventories was longer-lasting. The episodic hypolimnetic aeration and nitrate regeneration, and reallocation of the upper-water-column NO pool, induced a temporary shift in the denitrification mode from pelagic to benthic. With the boosting of the lake's sedimentary fixed-N filter, the ecosystem N-elimination potential was temporarily more fully exploited, sustainably reducing the lake's overall N pool and efficiently mitigating external N loading. Similarly, a high MOx potential was capitalized upon aeration of the CH4-loaded monimolimnion, so that a considerable fraction of Lake Lugano's total CH4 inventory, which had been built up over several decades, was rapidly removed by aerobic CH4 oxidation. Our study thus demonstrates that both the fixed N elimination and the aerobic CH4 oxidation capacities in a meromictic lake can be largely underutilized for decades. Denitrifying and CH4-oxidizing bacteria in stand-by mode can survive long periods of starvation under stratified conditions and rapidly resume high rates of activity without any delay, when conditions are more favorable upon de-stratification.
Our data add evidence that lakes can be a very strong source of CH4 to the atmosphere, in particular when the microbial CH4 filter in the water column is temporarily bypassed during exceptional mixing events, and large amounts of CH4 that resided in the deep waters for tens of years evade rapidly to the atmosphere by storage flux. Today, a decade after the mixing events, the North Basin is still far away from homeostasis regarding the monimolimnetic inventories of reduced solutes. For example, since 2006, the total annual mean CH4 pool increased at an average basin-wide accumulation rate of 66 t CH4 per year, and a return to steady state in the upcoming years or decades seems unlikely. Without seasonal turnover events, hypolimnetic CH4 recharge will once again increase the potential for major storage fluxes of CH4 to the atmosphere.
The ratio of hypolimnetic O2 consumption to diffusive O2 resupply in the North Basin has remained high until today, maintaining the anoxia below 130 m that was restored shortly after the 2005/2006 overturning events. Nevertheless, the legacy of long-term eutrophication has been reduced dramatically by the oxidation of a large pool of reduced solutes. The resulting transition to a positive redox balance has meant that the lake basin's total oxidative/respiratory demand has also been reduced. Whether the past and ongoing efforts to control lake productivity in Lake Lugano will ultimately lead to hypoxic or even oxic conditions in the deep North Basin will partly depend on the rate at which reduced substances are released from the sediments, re-charging the hypolimnetic O2 buffer, as well as on the frequency and intensity of future episodic mixing. The observed water-column destabilization trend associated with the reduction of density stratification (Holzner et al., 2009) should make the water column in the North Basin more susceptible to future large-scale convective mixing events. As of today, the water-column turnovers in 2005 and 2006 still present themselves as isolated events. Nevertheless, the “biogeochemical engine” of Lake Lugano will surely roar into overdrive again in the future.
Author Contributions
ML, MS, and MV planned research; MS and MV were responsible for hydrochemical measurements and data acquisition; ML, SW, MS, and MV made budget calculations, compiled and interpreted data; ML wrote the manuscript; JB, CF, HN, SW, and JZ contributed to data interpretation and writing/editing of the manuscript.
Conflict of Interest Statement
The authors declare that the research was conducted in the absence of any commercial or financial relationships that could be construed as a potential conflict of interest.
Acknowledgments
We thank Stefano Müller for technical assistance. This study was supported by Swiss National Science Foundation grants 21-121861 and 21-121491.
References
Aeschbach-Hertig, W., Holzner, C. P., Hofer, M., Simona, M., Barbieri, A., and Kipfer, R. (2007). A time series of environmental tracer data from deep, meromictic Lake Lugano, Switzerland. Limnol. Oceanogr. 52, 257–273. doi: 10.4319/lo.2007.52.1.0257
American Public Health Association, (APHA). (1989). Standard Methods for the Examination of Water and Wastewater. Washington, DC: American Water Works Association; Water Environment Federation.
Ashley, K. I. (1983). Hypolimnetic aeration of a naturally eutrophic lake - physical and chemical effects. Can. J. Fish. Aquat. Sci. 40, 1343–1359. doi: 10.1139/f83-157
Baldi, E., Pirocchi, L., and Tonolli, V. (1949). Relazione Preliminare Sulle Ricerche Idrobiologiche Condotte Sul Lago di Lugano (1946–1947). Berna: Ispettorato Federale Svizzero Per La Pesca.
Barbieri, A., and Mosello, R. (1992). Chemistry and trophic evolution of Lake Lugano in relation to nutrient budget. Aquat. Sci. 54, 219–237. doi: 10.1007/BF00878138
Barbieri, A., and Simona, M. (2001). Trophic evolution of Lake Lugano related to external load reduction: changes in phosphorus and nitrogen as well as oxygen balance and biological parameters. Lake Reserv. Manage. 6, 37–47. doi: 10.1046/j.1440-1770.2001.00120.x
Bastviken, D., Cole, J., Pace, M., and Tranvik, L. (2004). Methane emissions from lakes: dependence of lake characteristics, two regional assessments, and a global estimate. Global Biogeochem. Cycles 18, GB4009. doi: 10.1029/2004GB002238
Beutel, M. W. (2001). Oxygen consumption and ammonia accumulation in the hypolimnion of Walker Lake, Nevada. Hydrobiologia 466, 107–117. doi: 10.1023/A:1014533909086
Beutel, M. W., and Horne, A. J. (1999). A review of the effects of hypolimnetic oxygenation on lake and reservoir water quality. Lake Reserv. Manage 15, 285–297. doi: 10.1080/07438149909354124
Blees, J., Niemann, H., Wenk, C. B., Zopfi, J., Schubert, C. J., Kirf, M. K., et al. (2014a). Micro-aerobic bacterial methane oxidation in the chemocline and anoxic water column of deep south-Alpine Lake Lugano (Switzerland). Limnol. Oceanogr. 59, 311–324. doi: 10.4319/lo.2014.59.2.0311
Blees, J., Niemann, H., Wenk, C. B., Zopfi, J., Schubert, C. J., Jenzer, J. S., et al. (2014b). Bacterial methanotrophs drive the formation of a seasonal anoxic benthic nepheloid layer in an alpine lake. Limnol. Oceanogr. 59, 1410–1420. doi: 10.4319/lo.2014.59.4.1410
Blees, J. H., Niemann, H., Erne, M., Zopfi, J., Schubert, C. J., and Lehmann, M. F. (2015). Spatial variations in surface water methane super-saturation and emission in Lake Lugano, southern Switzerland. Aquat. Sci. doi: 10.1007/s00027-015-0401-z. Available online at: http://link.springer.com/article/10.1007/s00027-015-0401-z
Boehrer, B., and Schultze, M. (2008). Stratification of lakes. Rev. Geophys. 46, RG2005. doi: 10.1029/2006rg000210
Boström, B., Jansson, M., and Forsberg, C. (1982). Phosphorus release from lake sediments. Arch. Hydrobiol. Beih. Ergebn. Limnol. 18, 5–59.
Bührer, H. (1979). Formula for estimation of the total content of a substance in lakes. Schweiz. Z. Hydrol. 41, 418–420.
Carpenter, S. R., Caraco, N. F., Correll, D. L., Howarth, R. W., Sharpley, A. N., and Smith, V. H. (1998). Nonpoint pollution of surface waters with phosphorus and nitrogen. Ecol. Appl. 8, 559–568. doi: 10.1890/1051-0761(1998)008[0559:NPOSWW]2.0.CO;2
Carpenter, S. R., Cottingham, K. L., and Schindler, D. E. (1992). Biotic feedbacks in lake phosphorus cycles. Trends Ecol. Evol. 7, 332–336. doi: 10.1016/0169-5347(92)90125-U
Codispoti, L. A., Yoshinari, T., and Devol, A. H. (2005). “Suboxic respiration in the oceanic water column,” in Respiration in Aquatic Ecosystems, eds P. A. Del Giorgio and P. J. le B. Williams (Oxford: Oxford University Press), 225–247.
Commissione internazionale per la protezione delle acque Italo-Svizzere, (CIPAIS). (2005). Stato Limnologico del Lago di Lugano: Circolazione Invernale 2004–2005. Bellinzona: Bollettino dei Laghi Maggiore e Lugano No. 6.
Commissione internazionale per la protezione delle acque Italo-Svizzere, (CIPAIS). (2006). Stato Limnologico del Lago di Lugano: Circolazione Invernale 2005–2006. Bellinzona: Bollettino dei Laghi Maggiore e Lugano No. 7.
Crowe, S. A., Katsev, S., Leslie, K., Sturm, A., Magen, C., Nomosatryo, S., et al. (2011). The methane cycle in ferruginous Lake Matano. Geobiology 9, 61–78. doi: 10.1111/j.1472-4669.2010.00257.x
Duval, B., and Ludlam, S. D. (2001). The black water chemocline of meromictic Lower Mystic Lake, Massachusetts, USA. Int. Rev. Hydrobiol. 86, 165–181. doi: 10.1002/1522-2632(200104)86:2<165::AID-IROH165>3.0.CO;2-Y
Einsele, W. (1936). Ueber die Beziehungen des Eisenkreislaufs zum Phosphatekreislauf im eutrophen See. Arch. Hydriobiol. 29, 664–686.
Freymond, C. V., Wenk, C. B., Frame, C. H., and Lehmann, M. F. (2013). Year-round N2O production by benthic NOx reduction in a monomictic south-alpine lake. Biogeosciences 10, 8373–8383. doi: 10.5194/bg-10-8373-2013
Gächter, R. (1972). Die Bestimmung der Tagesraten der planktischen Primärproduktion: Modelle und In-situ-messungen. Schweiz. Z. Hydrol. 34, 211–244. doi: 10.1007/bf02502518
Gächter, R., and Marès, A. (1979). Comments on the acidification and bubbling method for determining phytoplankton production. Oikos 33, 69–73. doi: 10.2307/3544513
Gächter, R., and Müller, B. (2003). Why the phosphorus retention of lakes does not necessarily depend on the oxygen supply to their sediment surface. Limnol. Oceanogr. 48, 929–933. doi: 10.4319/lo.2003.48.2.0929
Gächter, R., and Wehrli, B. (1998). Ten years of artificial mixing and oxygenation: no effect on the internal phosphorus loading of two eutrophic lakes. Environ. Sci. Technol. 32, 3659–3665. doi: 10.1021/es980418l
Galloway, J. N., Aber, J. D., Erisman, J. W., Seitzinger, S. P., Howarth, R. W., Cowling, E. B., et al. (2003). The nitrogen cascade. Bioscience 53, 341–356. doi: 10.1641/0006-3568(2003)053[0341:TNC]2.0.CO;2
Holzner, C. P., Aeschbach-Hertig, W., Simona, M., Veronesi, M., Imboden, D. M., and Kipfer, R. (2009). Exceptional mixing events in meromictic Lake Lugano (Switzerland/Italy), studied using environmental tracers. Limnol. Oceanogr. 54, 1113–1124. doi: 10.4319/lo.2009.54.4.1113
Hupfer, M., and Lewandowski, J. (2008). Oxygen controls the phosphorus release from lake sediments - a long-lasting paradigm in limnology. Int. Rev. Hydrobiol. 93, 415–432. doi: 10.1002/iroh.200711054
Jensen, K., Sloth, N. P., Risgaard-Petersen, N., Rysgaard, S., and Revsbech, N. P. (1994). Estimation of nitrification and denitrification from microprofiles of oxygen and nitrate in model sediment systems. Appl. Environ. Microbiol. 60, 2094–2100.
Juutinen, S., Rantakari, M., Kortelainen, P., Huttunen, J. T., Larmola, T., Alm, J., et al. (2009). Methane dynamics in different boreal lake types. Biogeosciences 6, 209–223. doi: 10.5194/bg-6-209-2009
Kalvelage, T., Jensen, M. M., Contreras, S., Revsbech, N. P., Lam, P., Güenter, M., et al. (2011). Oxygen sensitivity of anammox and coupled N-cycle processes in oxygen minimum zones. PLoS ONE 6:e29299. doi: 10.1371/journal.pone.0029299
Katsev, S., Crowe, S. A., Mucci, A., Sundby, B., Nomosatryo, S., Haffner, G. D., et al. (2010). Mixing and its effects on biogeochemistry in the persistently stratified, deep, tropical Lake Matano, Indonesia. Limnol. Oceanogr. 55, 763–776. doi: 10.4319/lo.2009.55.2.0763
Katsev, S., Tsandev, I., L'Heureux, I., and Rancourt, D. G. (2006). Factors controlling long-term phosphorus efflux from lake sediments: exploratory reactive-transport modeling. Chem. Geol. 234, 127–147. doi: 10.1016/j.chemgeo.2006.05.001
Lehmann, M. F., Bernasconi, S. M., McKenzie, J. A., Barbieri, A., Simona, M., and Veronesi, M. (2004). Seasonal variation of the delta C-13 and delta N-15 of particulate and dissolved carbon and nitrogen in Lake Lugano: constraints on biogeochemical cycling in a eutrophic lake. Limnol. Oceanogr. 49, 415–429. doi: 10.4319/lo.2004.49.2.0415
Liu, R. M., Hofmann, A., Gulacar, F. O., Favarger, P. Y., and Dominik, J. (1996). Methane concentration profiles in a lake with a permanently anoxic hypolimnion (Lake Lugano, Switzerland-Italy). Chem. Geol. 133, 201–209. doi: 10.1016/S0009-2541(96)00090-3
Manne, A. S., and Richels, R. G. (2001). An alternative approach to establishing trade-offs among greenhouse gases. Nature 410, 675–677. doi: 10.1038/35070541
McCrackin, M. L., and Elser, J. J. (2010). Atmospheric nitrogen deposition influences denitrification and nitrous oxide production in lakes. Ecology 91, 528–539. doi: 10.1890/08-2210.1
Mengis, M., Gachter, R., Wehrli, B., and Bernasconi, S. (1997). Nitrogen elimination in two deep eutrophic lakes. Limnol. Oceanogr. 42, 1530–1543. doi: 10.4319/lo.1997.42.7.1530
Moosmann, L., Gaechter, R., Mueller, B., and Wuest, A. (2006). Is phosphorus retention in autochthonous lake sediments controlled by oxygen or phosphorus? Limnol. Oceanogr. 51, 763–771. doi: 10.4319/lo.2006.51.1_part_2.0763
Mortimer, C. H. (1942). The exchange of dissolved substances between mud and water in lakes. J. Ecol. 30, 147–201. doi: 10.2307/2256691
Prepas, E. E., and Burke, J. M. (1997). Effects of hypolimnetic oxygenation on water quality in Amisk Lake, Alberta, a deep, eutrophic lake with high internal phosphorus loading rates. Can. J. Fish. Aquat. Sci. 54, 2111–2120. doi: 10.1139/f97-125
Rasmussen, H., and Jørgensen, B. B. (1992). Microelectrode studies of seasonal oxygen-uptake in a coastal sediment - role of molecular-diffusion. Mar. Ecol. Prog. Ser. 81, 289–303. doi: 10.3354/meps081289
Rysgaard, S., Risgaard-Petersen, N., Sloth, N. P., Jensen, K., and Nielsen, L. P. (1994). Oxygen regulation of nitrification and denitrification in sediments. Limnol. Oceanogr. 39, 1643–1652. doi: 10.4319/lo.1994.39.7.1643
Schindler, D. W. (1974). Eutrophication and recovery in experimental lakes - implications for lake management. Science 184, 897–899. doi: 10.1126/science.184.4139.897
Schindler, D. W. (1977). Evolution of phosphorus limitation in lakes. Science 195, 260–262. doi: 10.1126/science.195.4275.260
Schmid, M., Halbwachs, M., Wehrli, B., and Wüest, A. (2005). Weak mixing in Lake Kivu: new insights indicate increasing risk of uncontrolled gas eruption. Geochem. Geophys. Geosys. 6, Q07009. doi: 10.1029/2004gc000892
Schreiber, F., Stief, P., Kuypers, M. M. M., and De Beer, D. (2014). Nitric oxide turnover in permeable river sediment. Limnol. Oceanogr. 59, 1310–1320. doi: 10.4319/lo.2014.59.4.1310
Schreiber, F., Wunderlin, P., Udert, K. M., and Wells, G. F. (2012). Nitric oxide and nitrous oxide turnover in natural and engineered microbial communities: biological pathways, chemical reactions, and novel technologies. Front. Microbiol. 3:372. doi: 10.3389/fmicb.2012.00372
Schubert, C. J., Diem, T., and Eugster, W. (2012). Methane emissions from a small wind shielded lake determined by Eddy covariance, flux chambers, anchored funnels, and boundary model calculations: a comparison. Environ. Sci. Technol. 46, 4515–4522. doi: 10.1021/es203465x
Seitzinger, S. P. (1988). Denitrification in freshwater and coastal marine ecosystems: ecological and geochemical significance. Limnol. Oceanogr. 33, 702–724.
Seitzinger, S., Harrison, J. A., Böhlke, J. K., Bouwman, A. F., Lowrance, R., Peterson, B., et al. (2006). Denitrification across landscapes and waterscapes: a synthesis. Ecol. Appl. 16, 2064–2090. doi: 10.1890/1051-0761(2006)016[2064:DALAWA]2.0.CO;2
Slomp, C. P., Mort, H. P., Jilbert, T., Reed, D. C., Gustafsson, B. G., and Wolthers, M. (2013). Coupled dynamics of iron and phosphorus in sediments of an oligotrophic coastal basin and the impact of anaerobic oxidation of methane. PLoS ONE 8:e62386. doi: 10.1371/journal.pone.0062386
Smith, V. H. (2003). Eutrophication of freshwater and coastal marine ecosystems - A global problem. Environ. Sci. Pollut. Res. Int. 10, 126–139. doi: 10.1065/espr2002.12.142
Solorzano, L., and Sharp, J. H. (1980). Determination of total dissolved nitrogen in natural waters. Limnol. Oceanogr. 25, 751–754. doi: 10.4319/lo.1980.25.4.0751
Steemann-Nielsen, E. (1952). The use of radioactive carbon (C14) for measuring organic production in the sea. ICES J. Mar. Sci. 18, 117–140. doi: 10.1093/icesjms/18.2.117
Sterner, R. W. (2008). On the phosphorus limitation paradigm for lakes. Int. Rev. Hydrobiol. 93, 433–445. doi: 10.1002/iroh.200811068
Van Cappellen, P., and Wang, Y. F. (1996). Cycling of iron and manganese in surface sediments: a general theory for the coupled transport and reaction of carbon, oxygen, nitrogen, sulfur, iron, and manganese. Am. J. Sci. 296, 197–243. doi: 10.2475/ajs.296.3.197
Vitousek, P. M., Aber, J. D., Howarth, R. W., Likens, G. E., Matson, P. A., Schindler, D. W., et al. (1997). Human alteration of the global nitrogen cycle: sources and consequences. Ecol. Appl. 7, 737–750. doi: 10.1890/1051-0761(1997)007[0737:haotgn]2.0.co;2
Vollenweider, R. A. (1965). Materiali ed idee per una idrochimica delle acque insubriche. Mem. Ist. Ital. Idrobiol. 19, 213–286.
Wenk, C. B., Blees, J., Zopfi, J., Veronesi, M., Bourbonnais, A., Schubert, C. J., et al. (2013). Anaerobic ammonium oxidation (anammox) bacteria and sulfide-dependent denitrifiers coexist in the water column of a meromictic south-alpine lake. Limnol. Oceanogr. 58, 1–12. doi: 10.4319/lo.2013.58.1.0001
Keywords: lake mixing, anoxia, phosphorous, nitrate, denitrification, methane oxidation, storage flux, eutrophication
Citation: Lehmann MF, Simona M, Wyss S, Blees J, Frame CH, Niemann H, Veronesi M and Zopfi J (2015) Powering up the “biogeochemical engine”: the impact of exceptional ventilation of a deep meromictic lake on the lacustrine redox, nutrient, and methane balances. Front. Earth Sci. 3:45. doi: 10.3389/feart.2015.00045
Received: 04 May 2015; Accepted: 27 July 2015;
Published: 17 August 2015.
Edited by:
Timothy Ferdelman, Max Planck Institute for Marine Microbiology, GermanyReviewed by:
Scott David Wankel, Woods Hole Oceanographic Institution, USAWilliam Patrick Gilhooly III, Indiana University – Purdue University Indianapolis, USA
Copyright © 2015 Lehmann, Simona, Wyss, Blees, Frame, Niemann, Veronesi and Zopfi. This is an open-access article distributed under the terms of the Creative Commons Attribution License (CC BY). The use, distribution or reproduction in other forums is permitted, provided the original author(s) or licensor are credited and that the original publication in this journal is cited, in accordance with accepted academic practice. No use, distribution or reproduction is permitted which does not comply with these terms.
*Correspondence: Moritz F. Lehmann, Department of Environmental Sciences, University of Basel, Bernoullistrasse 30, CH-4056 Basel, Switzerland,bW9yaXR6LmxlaG1hbm5AdW5pYmFzLmNo
†Present Address: Silvia Wyss, AquaPlus AG, Zug, Switzerland;
Jan Blees, Laboratory of Atmospheric Chemistry, Paul Scherrer Institute, Villigen, Switzerland