- Department of Marine Sciences, University of North Carolina, Chapel Hill, NC, USA
Extracellular enzymatic activities initiate microbially-driven heterotrophic carbon cycling in subsurface sediments. While measurement of hydrolytic activities in sediments is fundamental to our understanding of carbon cycling, these measurements are often technically difficult due to sorption of organic substrates to the sediment matrix. Most methods that measure hydrolysis of organic substrates in sediments rely on recovery of a fluorophore or fluorescently-labeled target substrate from a sediment incubation. The tendency for substrates to sorb to sediments results in lower recovery of an added substrate, and can result in data that are unusable or difficult to interpret. We developed a treatment using competitive desorption of a fluorescently-labeled, high molecular weight organic substrate that improves recovery of the labeled substrate from sediment subsamples. Competitive desorption treatment improved recovery of the fluorescent substrate by a median of 66%, expanded the range of sediments for which activity measurements could be made, and was effective in sediments from a broad range of geochemical contexts. More reliable measurements of hydrolytic activities in sediments will yield usable and more easily interpretable data from a wider range of sedimentary environments, enabling better understanding of microbially-catalyzed carbon cycling in subsurface environments.
Introduction
Heterotrophic microbial communities play an important role in organic carbon cycling in subsurface sediments. Increasing genomic evidence of the predominance of heterotrophy in the subsurface environment (Biddle et al., 2006; Fry et al., 2008; Lloyd et al., 2013) suggests that heterotrophic remineralization of organic matter plays a larger role in the sedimentary environment than previously appreciated. A key first step in the heterotrophic breakdown of organic carbon is extracellular enzymatic hydrolysis, in which compounds too large to be taken up directly are hydrolyzed to sizes small enough for transport into the cell. The need for measurements of enzymatic activities to quantify heterotrophic processes in subsurface sediments is evident, but the technical challenges associated with these measurements are such that comparatively few measurements have been made, particularly in subsurface environments.
Extracellular enzymatic activity is typically measured by addition of a fluorescently labeled substrate to an environmental sample, and hydrolysis is detected either as an increase in fluorescence as a fluorophore is cleaved (Hoppe, 1983) or as a change in molecular weight distribution as a fluorescent substrate is hydrolyzed into lower molecular weight products (Arnosti, 1996, 2003). In both cases, adequate recovery of the amended label or labeled substrate is necessary for interpretable results.
However, adequate recovery of fluorescent labels or labeled substrates is often difficult to achieve due to the tendency of organic compounds to sorb strongly to the sediment matrix. Sorption occurs when the organic substrates interact with sediment surfaces. Interaction mechanisms can include ligand exchange, cation bridges, or weak interactions including hydrophobic interactions, van der Waals forces, or H-bonding (Theng, 1979; Lutzow et al., 2006). High molecular weight substrates often adsorb more strongly than low molecular weight compounds (Podoll et al., 1987) and so pose a particular challenge for activity measurements, yet these measurements are especially important as most natural organic matter is biosynthesized as high molecular weight compounds.
The strength of sorption is dependent on the characteristics of the organic substrate as well as the sediment composition and mineralogy (Kaiser and Guggenberger, 2000). The interaction of these factors leads to great variation in the extent to which enzyme activities in sediments can be measured: in some sediments, activities are measured relatively easily, in other sediments sorption affects the quality of measurements to an extent that may affect the accuracy of results, and in some sediments there is such poor recovery of substrate that measurements of enzyme activities are not feasible. Thus, published data likely excludes sediments for which measurements are particularly challenging to obtain. Measuring activities in such sediments may be important for capturing the range of heterotrophic activities in the subsurface, however, and exclusion of such sediments may bias our understanding of microbial effects on environmental processes.
Several strategies have been used to attempt to overcome the effect of sorption on measurements of enzyme activity in sediments. Very dilute sediment slurries, for example 20:1 ratios of aqueous media to sediment, can be used to minimize sorption surface area relative to substrate concentration (Lloyd et al., 2013). Another approach has attempted to correct for adsorption by calculating the sorption affinity constant of the target molecule from a separate set of incubation standards with known concentrations of fluorophore, and back-calculating the total concentration of substrate hydrolyzed in the enzyme activity calculation (Coolen and Overmann, 2000; Coolen et al., 2002). Both of these approaches have limitations, however. High dilution of sediments necessarily reduces microbial interactions with the sediment matrix, but such interactions may be important, since the interactions of organic matter with sediment particles can affect the bioavailability of substrates (Keil et al., 1994; Chenu and Stotzky, 2002). Moreover, phenomena such as quorum sensing are dependent upon close spatial interactions of organisms and substrates (Hmelo et al., 2011), so quorum-sensing dependent enzymatic activities likely could not be measured in a dilute slurry. Ideally, experimental conditions should reflect natural conditions as much as possible. Correcting for sorption, aside from requiring additional time and resources to conduct incubations for sorption affinity constant calculations, assumes that sorption is at equilibrium within 8 h. Sorption can occur on both short and long timescales (Pignatello and Xing, 1996), however, and the factors affecting this vary by sediment type and characteristics, so correcting enzyme activities using sorption affinity constants may not always yield accurate results.
Here we present an alternative strategy to measure enzymatic activities in sediments, an approach that counteracts the effect of sorption by recovering adsorbed substrate. We adapted a method developed previously to measure extracellular enzymatic hydrolysis of high molecular weight organic matter in sediments and seawater (Arnosti, 1996, 2003). The original method involves addition of a fluorescently labeled, high molecular weight substrate to sediments. After incubation, sediment subsamples are centrifuged to obtain porewater containing the partially-hydrolyzed substrate, which are analyzed chromatographically to determine the molecular weight distribution of the hydrolysis products and thereby the hydrolysis rate. We have extended this method by developing a treatment to desorb amended labeled substrate from subsamples for better detection of enzymatic activities. We tested two desorption strategies, treatment of sediment slurry subsamples with extraction solutions at elevated pH, and treatment of subsamples with extraction solutions using competitive desorption. Elevated pH was tested because adsorption via ligand exchange occurs most strongly at acidic pH (Gu et al., 1994; Kaiser and Guggenberger, 2000), and compounds bound by this means may be more easily desorbed at high pH (Kaiser and Zech, 1999). Competitive desorption, addition of unlabeled substrate to a subsample in order to desorb the adsorbed fluorescently-labeled substrate, was tested since adsorption occurs when compounds compete to adsorb to a limited number of available sorption sites in the sediment matrix (Gu et al., 1994). The adsorption of a particular molecule is often reversible, and a given molecule can be displaced by other molecules that compete for the same sorption sites (Gu et al., 1994, 1996). Competitive displacement of adsorbed compounds has been demonstrated with mixtures of natural organic matter of similar or stronger adsorption affinities (Gu et al., 1996). We tested both pH and competitive desorption strategies, optimized a desorption extraction method, and demonstrated its effectiveness with a range of marine subsurface sediments. Here, we report the efficacy of our optimized extraction method and its applicability to subsurface sediments from a range of geochemical settings.
Materials and Methods
Sediment Collection and Characteristics
Sediments for development of the extraction treatment protocol (see below) were collected from the Marmara Sea. Once finalized, the extraction treatment was applied to sediments from a range of geochemical environments in the Eastern Mediterranean Sea and the Guaymas Basin.
Marmara Sea and Eastern Mediterranean Sediments
Sediment from the Marmara Sea and the Eastern Mediterranean were collected during R/V Meteor cruise M84 in February 2011 (SI Table 1). Surficial sediments from the Marmara Sea (40°47.97′ N, 27°43.49′ E, 600 m water depth) were collected by multicorer, and sediments from 570–585 and 520–530 cm depth horizons were collected by gravity corer. Sediments from the Eastern Mediterranean (33°02.00′ N, 32°38.00′ E, 1424 m water depth) were collected by gravity corer at 365, 385, 440, 455, 575, and 582–590 cm depth horizons. Individual depth intervals were subsampled from the cores into 50 mL centrifuge tubes, which were stored at 4°C in anaerobic chambers until use. Eastern Mediterranean sediments contained five sapropel layers that were cross-referenced with those described by Calvert and Fontugne (2001). Those used in these experiments included S4 (from 385 cm), S5 (455 cm), and S7 (582–590 cm).
Guaymas Basin Sediments
Sediments from the Guaymas Basin, a spreading center within the Gulf of California, were collected aboard the R/V El Puma in October 2014 (Buckley et al., 2015). Sediments were collected at 5 and 55 cm sediment depth at six locations (P1, P3, P5, P8, P10, and P13) that vary in geological and environmental context (SI Table 1). Sediment intervals from cores were subsampled into airtight plastic containers and stored at 4°C until use in incubations.
Sediment Incubation Preparation
Incubations with Marmara Sea sediment were used for initial development of the extraction treatment protocol in three preliminary experiments—PreX1, PreX2, and PreX3—using sediments from 0–5, 570–585, and 520–530 cm depth intervals, respectively. Autoclaved artificial seawater was added to homogenized sediments to make a 2:1 seawater:sediment slurry. Twenty-one milliliter of slurry was dispensed into each of two 50 mL-volume serum vials; one vial was autoclaved as a killed control, and one vial was used as a live experimental vial. These incubations were set up under aerobic conditions, and due to limited availability of sediments only chondroitin was used as a substrate.
In Eastern Mediterranean and Guaymas Basin sediments, all sample preparation was carried out in an anaerobic chamber under N2 atmosphere. Each sediment sample was homogenized in a sterile beaker with a sterile spatula. Artificial seawater (Sigma S9883), autoclaved and cooled under N2, was added in a 2:1 ratio to homogenized sediments and mixed thoroughly. Twenty-one milliliter of sediment slurry was portioned into each 50 mL-volume, sterile serum vial using a sterile serological pipette, and sealed with a stopper and crimp. Nine serum vials were prepared from each sediment section—three live incubations and one killed control for each of two substrates (chondroitin and laminarin), and a live blank control. The sealed vials were removed from the anaerobic chamber, and two vials were autoclaved for 30 min, then cooled to room temperature to serve as killed controls. Substrate addition and subsequent subsampling of the incubations was carried out by opening the serum vials under a stream of N2, using aseptic technique. Substrate was added in 175 μM monomer-equivalent concentrations; three of the live incubations and one killed control received fluorescently labeled chondroitin sulfate; three live incubations and one killed control received fluorescently labeled laminarin, and one live incubation served as a blank and did not receive substrate. Time zero samples were collected immediately after substrate addition; vials were then resealed with stoppers, crimped and stored at 4°C in the dark until further subsampling. Subsamples were taken at 3, 6, and 9 week timepoints.
Development of the Extraction Protocol
The three preliminary experiments—PreX1, PreX2, and PreX3—were used to develop and optimize the extraction treatment protocol. In each case, fluorescently-labeled chondroitin substrate was added to incubations at 175 μM monomer-equivalent concentrations (PreX1) or 350 μM monomer-equivalent concentrations (PreX2 and PreX3), which is the concentration typically used in previous slurry incubations (e.g., Arnosti, 2003, 2008).
In the first experiment (PreX1), three desorption conditions were tested: competitive desorption, desorption with solution at pH = 10, and desorption with solution at pH = 11. The incubations were set up as described in section Sediment Incubation Preparation, and the fluorescently-labeled chondroitin substrate was added. Subsamples were taken at t0 and 2 days. At each subsampling point, 0.5 mL of sediment slurry was removed from each vial and added to a treatment tube containing 2 mL of either 700 μM unlabeled chondroitin (competitive desorption), carbonate buffer at pH = 10 (pH10), carbonate buffer at pH = 11 (pH11), or a no-treatment control of 2 mL DI H2O. The no-treatment tubes were immediately centrifuged, and the supernatant was filtered through a 0.2 um pore-size filter and stored at −20°C. The treatment tubes were incubated and periodically shaken for 2 h in a 30°C waterbath before centrifuging, filtering, and storing. Based on the results (see Results), competitive desorption was selected for use in subsequent experiments.
In the second experiment, PreX2, multiple concentrations of unlabeled substrate were tested for use in the competitive desorption approach. Three sediment incubations were again set up in serum vials—a live incubation, a killed incubation, and a live blank incubation, and fluorescently-labeled chondroitin substrate was added. Subsamples were taken at t0, 2 days, and 6 days. At each timepoint, 0.5 mL of sediment slurry was added to each treatment tube containing 2 mL of a solution of 700 μM unlabeled chondroitin, 1,400 μM unlabeled chondroitin, or 2,800 μM unlabeled chondroitin. There was also a no-treatment DI H2O control. No-treatment tubes were immediately processed, while treatment tubes were incubated and periodically shaken for 2 h in a 30°C waterbath before processing. Based on the results, 2,800 μM concentrations of unlabeled chondroitin was selected for subsequent experiments.
The third experiment, PreX3, tested whether the addition of sodium dodecyl sulfate (SDS) provided additional improvement to the competitive desorption treatment method developed in PreX2 and PreX1. Three sediment incubations, a live, a kill, and a live blank, were used. Subsamples were taken at t0, 7, and 14 days. At each timepoint, 0.5 mL of sediment slurry was added to each treatment tube containing 2 mL of either 2,800 μM unlabeled chondroitin and 0.2% SDS, 2,800 μM unlabeled chondroitin only, or a no-treatment control of DI H2O. No-treatment tubes were immediately processed, and treatment tubes were incubated for 2 h in a 30°C waterbath before processing. Competitive desorption with addition of SDS was selected as the final extraction treatment method, and was applied to the sediments from the Eastern Mediterranean and Guaymas Basin.
Activity Measurements with Competitive Desorption Treatment
At each timepoint, subsamples were taken from each incubation to measure the potential activity of extracellular enzymes that hydrolyze chondroitin or laminarin (Figure 1). For each subsample, two 15 mL centrifuge tubes were prepared for an extraction treatment and for a no-treatment control, for a total of 18 falcon tubes per time point. Treatment tubes contained 0.5 mL of 14 mM unlabeled chondroitin or laminarin (2,800 μM in 2.5 mL), 0.5 mL 0.5% SDS (0.2% in 2.5 mL), and 1 mL DI H2O. No-treatment tubes contained 2 mL of DI H2O.
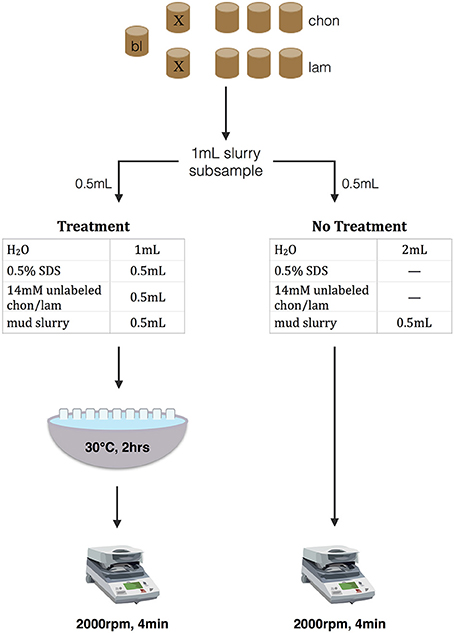
Figure 1. Conceptual figure of extraction treatment protocol for competitive desorption with SDS. Nine incubations were conducted for each sediment section: one live blank (“bl”), and for each substrate one kill control (“X”) and three live incubations. At subsampling, 1 mL of slurry is removed from each incubation; 0.5 mL of that subsample is treated with competitive desorption treatment (left), and 0.5 mL receives no treatment (right). After centrifuging both treatments are syringe filtered and stored at −20°C.
One Milliliter of sediment slurry was removed with a N2-flushed syringe from each serum vial under a N2 stream using aseptic technique, and 0.5 mL of slurry was added to each of the treatment and no-treatment tubes. No-treatment tubes were immediately centrifuged (2,000 rpm, 4 min), and the supernatant was filtered through a 0.2 μm pore size cellulose acetate syringe filter (Sterlitech CA0225) and stored in an epi tube at −20°C until analysis. Treatment tubes were allowed to process in a 30°C waterbath for 2 h, shaking manually every 10–15 min, to allow desorption to occur. The treatment tubes were then centrifuged and syringe filtered in the same manner as the no-treatment tubes, and stored at −20°C.
The proportion of fluorescently-labeled substrate that had been hydrolyzed into lower-molecular-weight products in each subsample was analyzed using gel permeation chromatography with fluorescence detection, after Arnosti (1996, 2003).
Fluorescent Substrate Preparation and Chromatogram Interpretation
The substrates laminarin and chondroitin were labeled with the fluorophore fluorosceinamine after the method of Arnosti (1996, 2003). In short, hydroxyl groups at multiple sites along the substrate are activated with cyanogen bromide, then coupled with the fluorophore fluoresceinamine, resulting in a high molecular weight substrate labeled with a fluorescent label, typically at multiple positions. The molecular weight distribution of a fluorescently-labeled substrate can be visualized using gel permeation chromatography with fluorescence detection. When a live incubation is amended with the substrate, hydrolytic activity shifts the molecular weight distribution of the fluorescent substrate from all high- to a mixture of high- and lower-molecular-weight hydrolysis products, and the hydrolysis rate can then be calculated from the change in molecular weight distribution (relative to standards of known molecular weight).
To visualize the molecular weight distribution of the substrate and any hydrolysis products, a sample is injected onto a 21 cm G50 Sephadex gel permeation chromatography column connected in series to a 19 cm G75 Sephadex column. These columns separate a sample by molecular weight such that the highest molecular weight compounds are excluded from the pores within a gel and the lower molecular weight compounds penetrate through the pores of the gel. The higher molecular weight compounds thus elute first from the columns, while the lower molecular weight compounds elute later. Standards of known molecular weight are used to determine elution times for different molecular weights. Elution time per sample in this study was 75 min, at a flow rate of 1 mL/min. Fluorescence of the column effluent was tracked at an emission wavelength of 530 nm (excitation at 490 nm) using a Hitachi fluorescence detector, and the molecular weight distribution was determined from the final chromatogram output of fluorescence signal vs. time. Hydrolysis rates were calculated from the change in molecular weight distribution from time zero to the time of sampling.
The added substrates, chondroitin and laminarin, are polysaccharides with different structures and characteristics: laminarin, a storage glucan in brown algae and diatoms, is a branched polymer of β-linked glucose units, while chondroitin is a sulfated polymer of n-acetyl glucosamine and glucuronic acid. The enzymes required to hydrolyze laminarin and chondroitin sulfate have been identified in marine bacteria (Alderkamp et al., 2007; Wegner et al., 2013; Xing et al., 2015), and activities of enzymes hydrolyzing these polysaccharides have been measured in a wide range of environments (e.g., Arnosti, 2008; Arnosti et al., 2009).
Statistical Analyses
When comparing whether the treatment resulted in increased total fluorescence intensity relative to no treatment in raw fluorescence units (FU; the detector signal in millivolts), a paired, one-sided t-test was used to compare chromatographic fluorescence intensities of incubations subjected to no treatment and treatment conditions. When comparing a percent improvement relative to zero, the no-treatment value was subtracted from the treatment value for a particular incubation, so an unpaired, one-sided t-test was used to test whether the percent improvement was greater than zero.
Reproducibility
The raw data from this project is stored in the BCO-DMO database (Hoarfrost and Arnosti, 2016). The scripts used to process and analyze the data, and generate the figures in this publication, can be found at the corresponding github repository (Hoarfrost, 2016).
Results
The extraction treatment presented here was developed to reduce the effects of adsorption on substrate recovery when measuring extracellular enzymatic activity in sediments using a fluorescently-labeled high molecular weight substrate, and to broaden the range of sediments in which enzyme activities can be measured using these substrates. Competitive desorption with unlabeled substrate and SDS proved to be effective in improving key chromatogram characteristics, by decreasing peak width and increasing fluorescence intensities (Figure 2, SI Figure 1). Some of the improvements in chromatogram characteristics can be summarized by the difference in area under the chromatogram, referred to here as the total integrated fluorescence intensity, between treatment and no treatment controls, which was used as an overall measure of chromatogram quality (Figures 2, 3A,B, 4, 5).
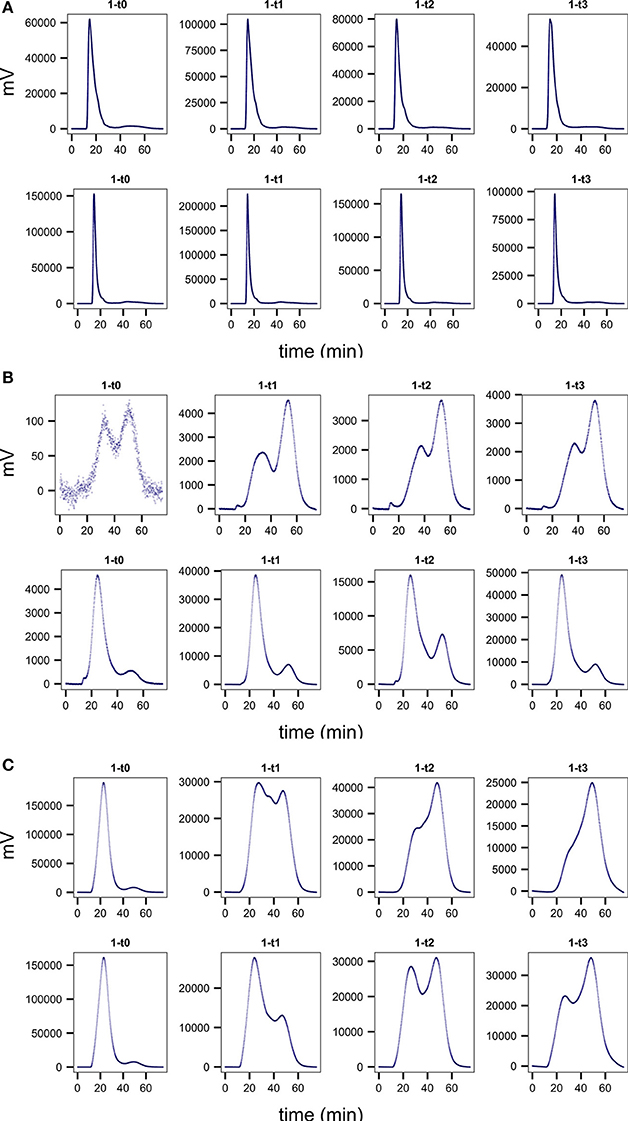
Figure 2. Representative chromatograms comparing treatment (bottom row) to no treatment controls (top row) in one of the live replicate incubations for (A) Mediterranean sapropel S4, 385 cm, chondroitin incubations (B) Guaymas core P13, 55 cm, laminarin, and (C) Guaymas core P1, 55 cm, laminarin. Note differences in scales on y axes. Improved chromatogram quality is seen in treatment incubations, with narrower peak widths, higher total integrated fluorescence, and a higher proportion of high- to low-molecular-weight substrate.
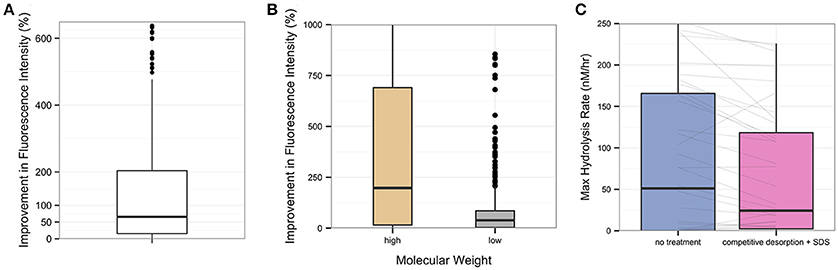
Figure 3. Overall improvement in fluorescence intensity by competitive desorption and SDS treatment, for all experiments in Guaymas Basin and Eastern Mediterranean sediment. (A) The percent improvement in total integrated fluorescence intensity in desorption-treated samples relative to their no-treatment controls. (B) Percent improvement in total integrated fluorescence intensity using desorption treatment for the high- and low- molecular weight portions of each subsample. High molecular weight is operationally identified as the first third of a chromatogram, while low molecular weight is the last third. (C) Change in calculated maximum hydrolysis rate (nM/hr) in treatment versus no-treatment controls. Gray lines connect treatment and no-treatment subsamples from a single incubation.
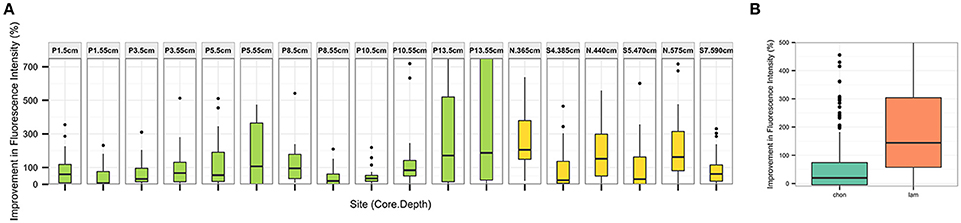
Figure 4. Percent improvement in total integrated fluorescence intensity using desorption treatment method (relative to no-treatment control) (A) for each sediment section tested. Sediments from Guaymas Basin in green, Eastern Mediterranean in yellow, and (B) for each substrate tested.
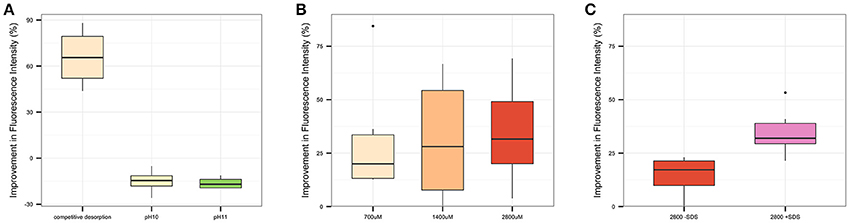
Figure 5. Percent improvement in total integrated fluorescence intensity for alternative extraction treatment candidates relative to a no-treatment control for (A) PreX1, in which competitive desorption, pH = 10, and pH = 11 extraction conditions are tested, (B) PreX2, in which three candidate concentrations of unlabeled substrate (700, 1400, and 2800 μM) in the extraction treatment are compared; and (C) PreX3, in which the presence or absence of SDS in competitive desorption are compared.
Competitive Desorption Treatment Effects on Chromatogram Quality
At all timepoints, desorption treatment improved several chromatogram characteristics (Figure 2, SI Figure 1). Overall, total integrated fluorescence intensities were higher in treatment relative to no treatment controls (Figure 3A), as can be seen by the difference in peak heights (Figure 2; note difference in scales on y axes). The desorption treatment was especially effective at desorbing the high molecular weight portion of the added substrate (Figure 3B), resulting in higher proportions of high- to low- molecular weight substrate, an effect that is particularly evident for laminarin in core P13 from Guaymas Basin (Figures 2B,C). Finally, the chromatogram peaks are sharper and peak width is narrower, as exemplified by the incubations with chondroitin in Mediterranean 385 cm sediments (Figures 2A,C). These characteristics result in higher quality chromatograms and lead to more easily interpretable rate calculations. In some cases, samples with no treatment applied resulted in very poor recovery of substrate and such low chromatogram intensities that they would be unusable (e.g., Figure 2B, panel 1-t0). In these cases, competitive desorption treatment enables measurement of enzymatic activities in sediments where such measurements otherwise could not be made.
Although the extraction protocol includes a 2-h incubation of a subsample treatment in a 30°C waterbath, this step does not appear to stimulate an increase in activity in the treatment subsamples that would otherwise bias our results. Treatment samples, in fact, yielded lower calculated hydrolysis rates (due to improvements in substrate recovery) than no-treatment controls, which are processed immediately without incubation in the waterbath (Figure 3C). The general activity patterns in the chromatograms, which may be summarized by how quickly the fluorescence in the low molecular weight portion of the chromatogram increased over time, were similar between no treatment and treatment controls despite differences in chromatogram quality and intensity (Figure 2). The relative rate of increase in fluorescence over time of low molecular weight substrate products within a particular incubation remained the same in the no treatment and treatment conditions (result of paired t-test, P = 0.98), even in highly active sediments.
Competitive Desorption Effects on Substrate Recovery and Calculated Hydrolysis Rates
Desorption treatment increased total integrated fluorescence intensity of the resultant chromatogram by a median of 66% (P < 0.001) relative to a no-treatment control (Figure 3A), with a median increase in fluorescence of 8.3 × 106 mV (P < 0.001). The improvement in fluorescence intensity is observed in both the high- and low-molecular weight portion of the chromatogram, but is particularly effective in improving recovery of the high molecular weight portion (Figure 3B). Recovery of high molecular weight substrate products is improved by a median of 200% (P = 0.01), while recovery of low molecular weight substrate products is improved by a median of 39% (P < 0.001).
The improved recovery of the substrate from the subsample results in a higher relative proportion of high- to low-molecular weight substrate than is observed in the no-treatment controls. The desorption treatment therefore results in a lower calculated hydrolysis rate in treatment samples (Figure 3C), with a median decrease in maximum hydrolysis rate of 5 nM/h in treatment subsamples relative to no-treatment controls (P < 0.01).
The competitive desorption treatment improves substrate recovery in all sediments and substrates tested (Figure 4), although there is some variation in the percent improvement dependent upon sampling site (Figure 4A) and substrate (Figure 4B).
Comparison of the Competitive Desorption Treatment with Low PH-Extraction
Several extraction treatment methods were tested in three preliminary experiments in order to develop and optimize the desorption protocol. Competitive desorption, using 700 μM unlabeled substrate, was compared to alternative extraction treatments using solutions with pH of either 10 or 11, as well as a no treatment control (Figure 5A). Competitive desorption was found to be most effective at recovering chondroitin, increasing integrated fluorescence intensity by a median of 66% (P < 0.01), while both pH extraction treatments actually decreased substrate recovery. Based on these results, competitive desorption was chosen as the basis of the extraction treatment method.
The concentration of unlabeled substrate to use during competitive desorption treatment was optimized in a second experiment (Figure 5B), comparing 700, 1,400, and 2,800 μM unlabeled substrate concentrations to a no-treatment control. Two thousand eight hundred micrometer concentrations improved integrated fluorescence intensity by a median of 32% (P < 0.01), a large improvement over 700 μM at 20% (P = 0.06) and a slight improvement over 1,400 μM concentrations at 28% (P = 0.07). Based on these results, competitive desorption with 2,800 μM concentrations of unlabeled substrate was chosen as the basis of the final extraction treatment protocol.
The addition of SDS to competitive desorption was compared to a no-treatment control in a third experiment (Figure 5C) to determine whether the inclusion of SDS with competitive desorption provides additional substrate recovery. While competitive desorption both with and without SDS improved substrate recovery, the addition of SDS increased integrated fluorescence intensity by a median of 32% (P < 0.001), whereas competitive desorption without SDS yielded a 17% increase (P < 0.001). Therefore, competitive desorption with SDS was chosen as the final extraction treatment protocol.
Applicability of Competitive Desorption Treatment Using Multiple Substrates in Sediments from Diverse Environments
We measured extracellular enzymatic hydrolysis in sediments from varied settings, and were able to detect hydrolysis at activities ranging from near-zero in the Eastern Mediterranean to more than 200 nM/h in parts of Guaymas Basin (Figure 6). The desorption treatment was more effective at increasing total integrated fluorescence intensities than a no-treatment control at every site (Figure 4A), with median percent improvement in total integrated fluorescence ranging from 7% (Guaymas core P1 depth 55 cm, P = 0.02) to 200% (Mediterranean non-sapropel, depth 365 cm, P < 0.001).
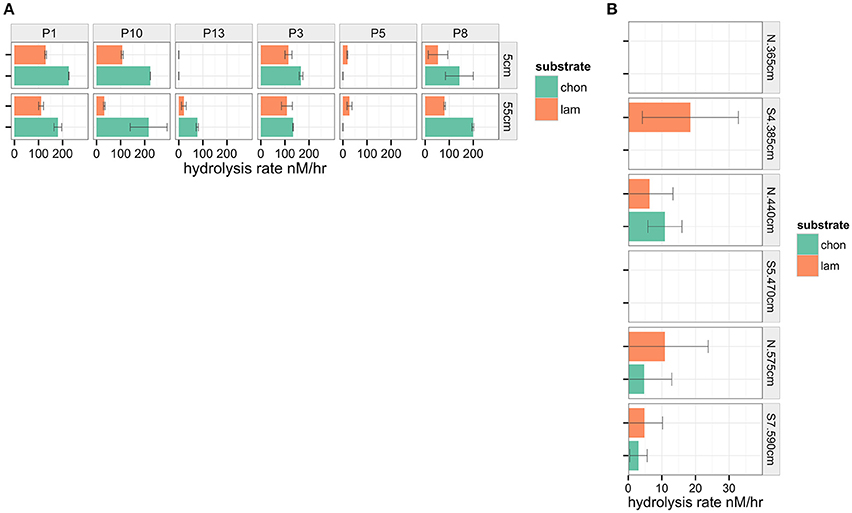
Figure 6. Maximum hydrolysis rate (nM/hr) measured in sediments from (A) Guaymas basin and (B) Eastern Mediterranean. Note differences in scale on y axes.
The greatest improvements in total integrated fluorescence intensities were observed in the sediments where the no treatment controls were uninterpretable due to very low substrate recovery. One such example is Guaymas core P13 at 55 cm (Figure 2B), with a median improvement in integrated fluorescence intensities of 190%. The high quality chromatograms observed after desorption treatment in these cases demonstrates that this protocol can expand the range of sediments in which enzymatic activities can be measured.
Even in cases where the median improvement in fluorescence was relatively minor, for example Guaymas core P1 at depth 55 cm, the treatment often improved the chromatogram quality in other tangible ways (Figure 2C). Guaymas P1-55 cm exhibited very high hydrolytic activity, but the treatment recovered a large portion of high molecular weight substrate that was not recovered in the no treatment control, such that the final calculated hydrolysis rate was 110.6 nM/hr in the treatment incubation relative to the much higher 169.8 nM/hr in the no-treatment control.
The desorption treatment was effective in improving total integrated fluorescence intensity for both chondroitin and laminarin (Figure 4B, e.g., Figure 2). The treatment had a greater effect on laminarin recovery than chondroitin recovery: laminarin integrated fluorescence intensity was improved by a median of 140% (P < 0.001), while chondroitin fluorescence was improved by a median of 20% (P = 0.01).
Discussion
Microbial communities in sediments play an important role in driving key biogeochemical cycles. Organic carbon cycling is often the dominant metabolic function of microbial communities in subsurface environments (e.g., Biddle et al., 2006; Fry et al., 2008; Lloyd et al., 2013). Reliable measurements of enzymatic activities in sediments are fundamental to our understanding of microbial carbon-cycling potential in sedimentary environments. However, our ability to measure heterotrophic enzymatic activities directly in sediments, particularly subsurface sediments, has been hampered by the tendency for amended substrates to sorb to the sediment matrix. The extraction treatment presented here facilitates the measurement of enzyme activities in sediments by improving recovery of fluorescently labeled substrates in sediments from a range of geochemical settings. This treatment further enables the measurement of enzyme activities in sediments that might not otherwise yield usable data due to effects of sorption. This approach can be used to directly link microbial potential activities to genetic potential or biogeochemical processes, to better understand the role of microbial communities in subsurface carbon cycling. This approach may also be useful in remediation applications, where, for example, one would like to quantify the bioavailability of sediment-sorbed organic contaminants (e.g., Alexander, 2000; Megharaj et al., 2011), or in agricultural applications where the rate of recycling of nitrogen- or phosphorous-containing organic substrates by soil microbial communities is of interest for applications in optimizing food production (Berg, 2009) or minimizing fertilizer use (Adesemoye and Kloepper, 2009).
Treatment of sediment-sorbed fluorescently labeled substrates using competitive desorption and SDS proved effective in all sediments and substrates tested (Figure 4) improving chromatogram fluorescence intensities (Figure 3A) and leading to hydrolysis rate measurements reflecting improved recovery on high and low molecular weight substrate products (Figure 3B). Both highly active and near-zero activities were detected using this procedure (Figure 6) and desorption treatment improved chromatogram characteristics in both types of sediments (e.g., Figure 2A vs. c). The geochemical and environmental contexts of the source sediments used to test the extraction treatment were varied, encompassing sapropelic sediments from the Mediterranean, with high concentrations of highly recalcitrant organic carbon; non-sapropelic, oligotrophic Mediterranean sediments; and sediments from Guaymas Basin ranging from highly compacted and sulfidic, to hemipelagic and diatom-rich, to coarse and sandy terrestrially-influenced sediments. The two substrates tested were distinct polysaccharides with distinct compositions and conformations, but both yielded improved recoveries when treated with the competitive desorption treatment (Figure 4B). The rates obtained by the competitive desorption treatment were not significantly affected by the incubation at 30°C in extraction buffer for 2 h, while the improvements in chromatogram quality and hydrolysis rate calculations were substantial.
The hydrolysis rates measured in subsurface sediments highlighted the contrasting potential activities of sediment microbial communities in the Gulf of Mexico and Mediterranean Sea. Most locations in Guaymas Basin were much more active (ca. 100–200 nM/hr) than in the Eastern Mediterranean (ca. 0–20 nM/h, Figure 6). Both of these subsurface sites exhibited lower activities than have been observed in surficial sediments (ca. upper 15 cm of sediments) in previous studies, including Arctic sites (Arnosti, 2008) and sediments from the Gulf of Mexico (Arnosti et al., 2009). While the effect of desorption treatment on producing lower hydrolysis rates may have contributed to the difference in hydrolysis rates observed in this study, the 2–4 order of magnitude difference in microbial community abundance between shallow surface sediments and deeper subsurface sediments (Kallmeyer et al., 2012) likely underlies the considerable difference in measured rates. The activities measured here are potential enzymatic activities, and thus reflect the relative capacities of the nascent microbial communities to access the added substrate. The difference in rates observed between Guaymas and Mediterranean sediments suggests that these lower rates may also be indicative of differences in microbial communities between surficial and subsurface depths.
The efficacy of the extraction treatment in all of these settings suggests that the competitive desorption approach may be useful as a general (and therefore standardizable) approach to substrate recovery in sediment enzyme activity measurements. The competitive desorption treatment was especially effective at recovering the high molecular weight fraction of substrate products (Figure 3B). Recovery of high molecular weight substrate products is particularly useful in natural settings, because high molecular weight compounds are more likely to sorb to sediment than low molecular weight compounds (Podoll et al., 1987). The rates calculated from treatment samples may better reflect the potential hydrolysis rate occurring in the incubation, whereas the higher rate calculated for the untreated sample may be exaggerated due to disproportional sorption of the high molecular weight fraction. The demonstrated applicability of this treatment in sediments from broad environmental settings may be due to the mechanism of competitive desorption, which directly competes for sorption sites with a substrate of interest, regardless of the complex combination of factors that may affect adsorption rates (Pignatello and Xing, 1996) that may vary widely depending on the sediment and substrate characteristics.
Limitations of this extraction treatment will therefore most likely occur when sorption is more irreversible, i.e., where the decrease in entropy due to complexation of a substrate with sediment is prohibitively large. In this case, once sorbed a substrate is less likely to exchange with the aqueous environment, leaving less opportunity for an unlabeled “competitive desorber” to replace it and release the labeled substrate. Although the extraction treatment was effective in all settings, the overall percent improvement in total integrated fluorescence intensities was variable across sites (Figure 4A). For example, in the Eastern Mediterranean core substrate recovery was much better in the non-sapropel (“N” segments) than the sapropel (“S”) segments, perhaps due to the high concentrations of organic carbon in the sapropel segments. Future experiments may test a wider range of substrates, and additional sediments and soils with geological histories and contexts not investigated in this study, to better estimate the true variance of this extraction treatment approach and its overall applicability.
This method has been developed to improve measurements made with high molecular weight fluorescent substrates prepared after the method of Arnosti (1996, 2003), and is focused on improving recovery of hydrolyzed fragments of high molecular weight substrates. Other common methods of measuring enzymatic activities include use of low molecular weight substrate proxies, typically consisting of monomers linked to MUF or MCA fluorophores, after the method of Hoppe (1983). Measurement of enzyme activities with substrate proxies rely on the release of a fluorophore that becomes fluorescent upon hydrolysis from the attached monomer. The method is thus affected by sorption of the freed fluorophore to the sediment matrix. In this case, an adaptation of the competitive desorption treatment would require use of a non-fluorescent analog of the MCA or MUF fluorophore.
Measuring the rate at which microbial communities hydrolyze organic compounds in subsurface sediments is essential to our understanding of subsurface ecosystems and their influence on biogeochemistry, environmental remediation, and agricultural productivity. The method presented here provides a promising means to more reliably and accurately measure heterotrophic extracellular enzymatic activities in sediments not otherwise amenable to these measurements.
Author Contributions
AH and CA designed the experiments. AH and RS set up and subsampled sediment incubations, and processed subsamples. AH analysed data. AH, CA, and RS wrote the manuscript.
Funding
This work was supported by a CDEBI Graduate Fellowship to AH and a CDEBI research grant to CA, NSF award number OCE-0939564. Additional grants from NSF OCE-1449604 (to Andreas Teske) for sample collection in the Guaymas Basin, and NSF OCE-1332881 (to CA) provided partial support of this work. The collection (by Andreas Teske) of sediments from the Eastern Mediterranean and Marmara Sea was made possible by funding from the DARCLIFE project to Andreas Teske (UNC) and Kai Hinrichs (MARUM, Bremen).
Conflict of Interest Statement
The authors declare that the research was conducted in the absence of any commercial or financial relationships that could be construed as a potential conflict of interest.
Acknowledgments
The authors would like to thank Andreas Teske and Luke McKay who collected and generously provided the sediments used in this study. This publication is CDEBI Contribution 355.
Supplementary Material
The Supplementary Material for this article can be found online at: http://journal.frontiersin.org/article/10.3389/feart.2017.00013/full#supplementary-material
References
Adesemoye, A., and Kloepper, J. (2009). Plant microbe interactions in enhanced fertilizer use efficiency. Appl. Microbiol. Biotechnol. 85, 1–12. doi: 10.1007/s00253-009-2196-0
Alderkamp, A.-C., van Rijssel, M., and Bolhuis, H. (2007). Characterization of marine bacteria and the activity of their enzyme systems involved in degradation of the algal storage glucan laminarin. FEMS Microbiol. Ecol. 59, 108–117. doi: 10.1111/j.1574-6941.2006.00219.x
Alexander, M. (2000). Aging, bioavailability, and overestimation of risk from environmental pollutants. Environ. Sci. Technol. 34, 4259–4265. doi: 10.1021/es001069+
Arnosti, C. (1996). A new method for measuring polysaccharide hydrolysis rates in marine environments. Org. Geochem. 25, 105–115. doi: 10.1016/S0146-6380(96)00112-X
Arnosti, C. (2003). Fluorescent derivatization of polysaccharides and carbohydrate-containing biopolymers for measurement of enzyme activities in complex media. J. Chromatogr. B Analyt. Technol. Biomed. Life Sci. 793, 181–191. doi: 10.1016/S1570-0232(03)00375-1
Arnosti, C. (2008). Functional differences between Arctic seawater and sedimentary microbial communities: contrasts in microbial hydrolysis of complex substrates. FEMS Microbiol. Ecol. 66, 343–351. doi: 10.1111/j.1574-6941.2008.00587.x
Arnosti, C., Ziervogel, K., Ocampo, L., and Ghobrial, S. (2009). Enzyme activities in the water column and in shallow permeable sediments from the northeastern Gulf of Mexico. Estuar. Coast. Shelf Sci. 84, 202–208. doi: 10.1016/j.ecss.2009.06.018
Berg, G. (2009). Plant-microbe interactions promoting plant growth and health: perspectives for controlled use of microorganisms in agriculture. Appl. Microbiol. Biotechnol. 84, 11–18. doi: 10.1007/s00253-009-2092-7
Biddle, J. F., Lipp, J. S., Lever, M. A., Lloyd, K. G., Sørensen, K. B., Anderson, R., et al. (2006). Heterotrophic Archaea dominate sedimentary subsurface ecosystems off Peru. Proc. Natl. Acad. Sci. U.S.A. 103, 3846–3851. doi: 10.1073/pnas.0600035103
Buckley, A., McKay, L. J., Turner, T., Chanton, J., Hensen, C., Benninger, L., et al. (2015). “Biogeochemical and Microbial Survey of Gravity Cores from the Guaymas Basin and Sonora Margin”, in Talk and Abstract. Fall Meeting American Geophysical Union, (San Francisco, CA). 14–18.
Calvert, S., and Fontugne, M. (2001). On the late Pleistocene-Holocene sapropel record of climatic and oceanographic variability in the eastern Mediterranean. Paleoceanography 16, 78–94. doi: 10.1029/1999PA000488
Chenu, C., and Stotzky, G. (2002). “Interactions between microorganisms and soil particles: an overview”, in Interactions between Soil Particles Microorganisms: Impact Terrestrial Ecosystem, eds P. M. Huang, J.-M. Bollag, and N. Senesi (John Wiley & Sons, Ltd.), 3–40.
Coolen, M. J. L., Cypionka, H., Sass, A. M., Sass, H., and Overmann, J. (2002). Ongoing modification of Mediterranean Pleistocene sapropels mediated by prokaryotes. Science 296, 2407–2410. doi: 10.1126/science.1071893
Coolen, M. J. L., and Overmann, J. (2000). Functional Exoenzymes as indicators of metabolically active bacteria in 124,000-year-old sapropel layers of the eastern Mediterranean Sea. Appl. Environ. Microbiol. 66, 2589–2598. doi: 10.1128/AEM.66.6.2589-2598.2000
Fry, J. C., Parkes, R. J., Cragg, B. A., Weightman, A. J., and Webster, G. (2008). Prokaryotic biodiversity and activity in the deep subseafloor biosphere. FEMS Microbiol. Ecol. 66, 181–196. doi: 10.1111/j.1574-6941.2008.00566.x
Gu, B. H., Schmitt, J., Chen, Z. H., Liang, L. Y., and McCarthy, J. F. (1994). Adsorption and desorption of natural organic-matter on iron-oxide - mechanisms and models. Environ. Sci. Technol. 28, 38–46. doi: 10.1021/es00050a007
Gu, B., Mehlhorn, T. L., Liang, L., and McCarthy, J. F. (1996). Competitive adsorption, displacement, and transport of organic matter on iron oxide: II. Displacement and transport. Geochim. Cosmochim. Acta 60, 2977–2992. doi: 10.1016/0016-7037(96)00157-3
Hmelo, L. R., Mincer, T., and Van Mooy, B. A. S. (2011). Possible influence of bacterial quorum sensing on the hydrolysis of sinking particulate organic carbon in marine environments. Environ. Microbiol. Rep. 3, 682–688. doi: 10.1111/j.1758-2229.2011.00281.x
Hoarfrost, A. (2016). SedS. Github Repository. doi: 10.5281/zenodo.233018. Available online at: https://github.com/ahoarfrost/SedS
Hoarfrost, A., and Arnosti, C. (2016). Investigating microbial activities driving organic matter transformations in the deep subsurface. Biol. Chem. Oceanogr. Data Manag. Off. Available online at: http://www.bco-dmo.org/project/662055
Hoppe, H. (1983). Significance of exoenzymatic activities in the ecology of brackish water: measurements by means of methylumbelliferyl-substrates. Mar. Ecol. Prog. Ser. 11, 299–308. doi: 10.3354/meps011299
Kaiser, K., and Guggenberger, G. (2000). The role of DOM sorption to mineral surfaces in the preservation of organic matter in soils. Org. Geochem. 31, 711–725. doi: 10.1016/S0146-6380(00)00046-2
Kaiser, K., and Zech, W. (1999). Release of natural organic matter sorbed to oxides and a subsoil. Soil Sci. Soc. Am. J. 63:1157. doi: 10.2136/sssaj1999.6351157x
Kallmeyer, J., Pockalny, R., Adhikari, R. R., Smith, D. C., and D'Hondt, S. (2012). Global distribution of microbial abundance and biomass in subseafloor sediment. Proc. Natl. Acad. Sci. U.S.A. 109, 16213–16216. doi: 10.1073/pnas.1203849109
Keil, R., Montluçon, D., Prahl, F., and Hedges, J. (1994). Sorptive preservation of labile organic matter in marine sediments. Nature 370, 549–552. doi: 10.1038/370549a0
Lloyd, K. G., Schreiber, L., Petersen, D. G., Kjeldsen, K. U., Lever, M. A., Steen, A. D., et al. (2013). Predominant archaea in marine sediments degrade detrital proteins. Nature 496, 215–218. doi: 10.1038/nature12033
Lutzow, M. V., Kogel-Knabner, I., Ekschmitt, K., Matzner, E., Guggenberger, G., Marschner, B., et al. (2006). Stabilization of organic matter in temperate soils: mechanisms and their relevance under different soil conditions - A review. Eur. J. Soil Sci. 57, 426–445. doi: 10.1111/j.1365-2389.2006.00809.x
Megharaj, M., Ramakrishnan, B., Venkateswarlu, K., Sethunathan, N., and Naidu, R. (2011). Bioremediation approaches for organic pollutants: a critical perspective. Environ. Int. 37, 1362–1375. doi: 10.1016/j.envint.2011.06.003
Pignatello, J. J., and Xing, B. (1996). Mechanisms of slow sorption of organic chemicals to natural particles. Environ. Sci. Technol. 30, 1–11. doi: 10.1021/es940683g
Podoll, R. T., Irwin, K. C., and Brendlinger, S. (1987). Sorption of water-soluble oligomers on sediments. Environ. Sci. Technol. 21, 562–568. doi: 10.1021/es00160a006
Theng, B. K. G. (1979). Clay-Polymer interactions: summary and perspectives. Clays Clay Miner. 30, 1–10. doi: 10.1346/CCMN.1982.0300101
Wegner, C.-E., Richter-Heitmann, T., Klindworth, A., Klockow, C., Richter, M., Achstetter, T., et al. (2013). Expression of sulfatases in Rhodopirellula baltica and the diversity of sulfatases in the genus Rhodopirellula. Mar. Genomics 9, 51–61. doi: 10.1016/j.margen.2012.12.001
Keywords: carbon degradation, microbial activity, deep biosphere, hydrolysis, polysaccharides
Citation: Hoarfrost A, Snider R and Arnosti C (2017) Improved Measurement of Extracellular Enzymatic Activities in Subsurface Sediments Using Competitive Desorption Treatment. Front. Earth Sci. 5:13. doi: 10.3389/feart.2017.00013
Received: 27 October 2016; Accepted: 02 February 2017;
Published: 16 February 2017.
Edited by:
Donato Giovannelli, Earth-Life Science Institute, Tokyo Institute of Technology, JapanReviewed by:
Jason B. Sylvan, Texas A&M University, USAElena Manini, ISMAR-Istituto di Scienze Marine (CNR), Italy
Copyright © 2017 Hoarfrost, Snider and Arnosti. This is an open-access article distributed under the terms of the Creative Commons Attribution License (CC BY). The use, distribution or reproduction in other forums is permitted, provided the original author(s) or licensor are credited and that the original publication in this journal is cited, in accordance with accepted academic practice. No use, distribution or reproduction is permitted which does not comply with these terms.
*Correspondence: Adrienne Hoarfrost, YWRyaWVubmUubC5ob2FyZnJvc3RAdW5jLmVkdQ==