- 1Department of Biology, University of Southern Denmark, Odense, Denmark
- 2Department of Palaeobiology, Swedish Museum of Natural History, Stockholm, Sweden
- 3Department of Earth Sciences, University of Hamburg, Hamburg, Germany
The exploration of Mars is largely based on comparisons with Earth analog environments and processes. The up-coming NASA Mars mission 2020 and ExoMars 2020 has the explicit aim to search for signs of life on Mars. During preparations for the missions, glaring gaps in one specific field was pointed out: the lack of a fossil record in igneous and volcanic rock. Earth’s fossil record is almost exclusively based on findings in sedimentary rocks, while igneous rocks have been considered barren of life, including a fossil record of past life. Since martian volcanic rocks will be targeted in the search for biosignatures, the lack of a terrestrial analog fossil record is an obvious impediment to the scientific aim of the mission. Here we will briefly review the knowledge of microscopic life in deep rock and deep time. Focus will be on underexplored environments in subseafloor crustal rocks, and on ancient environments harboring early prokaryotic and eukaryotic lineages. We will highlight some of the aspects that need immediate attention and further investigations to meet the scientific goals of the missions. The current paper is a first step toward the long-term aim to establish an atlas of the fossil record in volcanic rocks, which can be of use for the up-coming space missions.
Introduction
NASAs Mars mission 2020 is planned for launch in 2020 (National Research Council, 2011). One of the main scientific goals of the mission is to search for biosignatures including signs of past life on the planet (Farley and Williford, 2017). Previous missions have investigated the habitability of the planet and conditions favorable for life, but this will be the first mission dedicated to the search for actual signs of life, extant or fossil. The mission rover will search in situ and also assemble cache of samples to be returned to Earth by a later mission (McLennan et al., 2012). The landing site options are now down to three, and in 2018 the final destination will be selected. Beside the NASA mission, ESA is planning the ExoMars mission with the overall goal to establish if life ever was present on Mars, which is tentatively planned for launch 2020 (Vago et al., 2017). Thus, two major missions to Mars with astrobiological aims are planned for the next decade.
Discussions regarding the most promising landing sites and what geological environments that are most favorable from a context of harboring life, has preceded the planning- and are still on-going. In both Mars programs, an analogous terrestrial fossil record has been used to prepare for where geographically, the search should be focused, and what to search for (Westall et al., 2015; Farley and Williford, 2017; Vago et al., 2017). However, the fossil record on Earth is almost exclusively based on fossils from sedimentary rocks and represents aquatic environments. Though aquatic environments did exist on Mars in Noachian times (∼4.1 to 3.7 Ga), and sedimentary rocks from that period still remain (Mustard et al., 2008; Ehlmann et al., 2009; Fassett and Head, 2011; Carter et al., 2013; Ehlmann and Edwards, 2014; Grotzinger et al., 2015; Goudge et al., 2016), an overwhelming fraction of the potential habitats on Mars consists of volcanic igneous rocks (Des Marais, 2010; Ehlmann et al., 2011; Cockell, 2014a,b). In both Mars programs, a terrestrial fossil record of volcanic rocks as an analog to a potential martian fossil record is missing (Westall et al., 2015).
The exploration of life in deep environments has during the last two decades significantly enhanced our understanding of the distribution, abundance and diversity of life on Earth. This has also opened up the question for potential life beyond our own planet (Onstott, 2016). The continental crust, the marine sediments and the igneous oceanic crust represents together a vast volume, encompassing the largest microbial habitat on Earth (Schrenk et al., 2009; Orcutt et al., 2011). The organisms of the deep biosphere dwell within rocks and sediments in an endolithic life style, but can also occupy exposed parts of the ocean floor and hydrothermal vents. Life has been found throughout deep habitats to a maximum of ∼5 km depths in the continental crust, in the deepest marine sediments at ∼11,000 m water depth, and as fossilized remains at ∼1 km depths in igneous oceanic crust, thus expanding, by magnitudes, previous notions of the limits of life (McMahon and Parnell, 2014; Ivarsson et al., 2015c; Magnabosco et al., 2018).
The igneous oceanic crust consists exclusively of volcanically erupted basalts, geochemically very similar to the volcanic rocks that dominate the Martian geology. It is only recently that the igneous oceanic crust has been recognized as the largest aquifer on Earth and, with that, also potentially the world’s largest microbial habitat (Schrenk et al., 2009; Ivarsson et al., 2015c, 2016). As investigations of the microbial communities with traditional molecular methods have proven difficult due to contamination of seawater, paleontological material have been used as an alternative to explore this deep, hidden biosphere (Ivarsson et al., 2015c). However, the fossil record of the oceanic crust is far from comparable to the fossil record of sedimentary rocks, and it is somewhat of a paradox that the longevity of Earths most voluminous microbial habitat is more or less unexplored.
Endolithic (rock-dwelling) microorganisms use micro-fractures in the host rock for migration and colonization and grow complex and extended communities on the fracture walls (Bengtson et al., 2014; Ivarsson et al., 2015a,b,c,d). Upon death, the microbial communities become fossilized and preserved as microfossils in situ on the fracture walls. Fossilized microorganisms are to be seen as snapshots of the microbial habitats in which they once lived, and information on how they interacted with their surrounding is thus “frozen” in situ. The igneous oceanic crust contains a record of trace fossils in volcanic glass known from present to 3.5 Ga (Furnes et al., 2008; Staudigel et al., 2008) but such fossils are strongly debated, especially the ancient ones, and abiotic explanations have been put forward (Grosch and McLoughlin, 2014). Additionally, a patchy record of fossilized microorganisms from fractures, veins and open pore space in subseafloor basalts and ophiolites, stretching from present to Devonian, represent a more valid record and supports the continuum of deep life in those settings (Peckmann et al., 2008; Eickmann et al., 2009; Ivarsson et al., 2015c). Recent, evidence shows that this biosphere was active already at 2.4 Ga, and probably before that (Bengtson et al., 2017).
To be able to use the fossil record in volcanic rocks as an analogous system in Martian exploration, a more comprehensive understanding of the deep life is needed, as well as more information of how this life is fossilized and preserved. We need to establish a fossil record coherent over time, ranging from the Archean to present, in the form of an atlas to better classify fossil findings but also to show where and what to search for. Firm protocols are needed on how to study and prove biogenicity of such fossils. By doing this we will enhance our understanding of early and present life on Earth, but also how to approach the task of searching for signs of life on other planets. Thus, exploring the deep life of our planet is one of the great challenges of our time and will lead to a better understanding of the distribution of life on Earth and beyond.
The Deep Biosphere
The definition of the deep biosphere comprises ecosystems in both oceanic and continental deep settings, and the habitability with respect to depth is restricted by rock porosity, water availability and maximum temperature, depending on the local geothermal gradient (Heim, 2011). In this paper, we will focus on the volcanic portions of the subsurface and subseafloor (Figure 1).
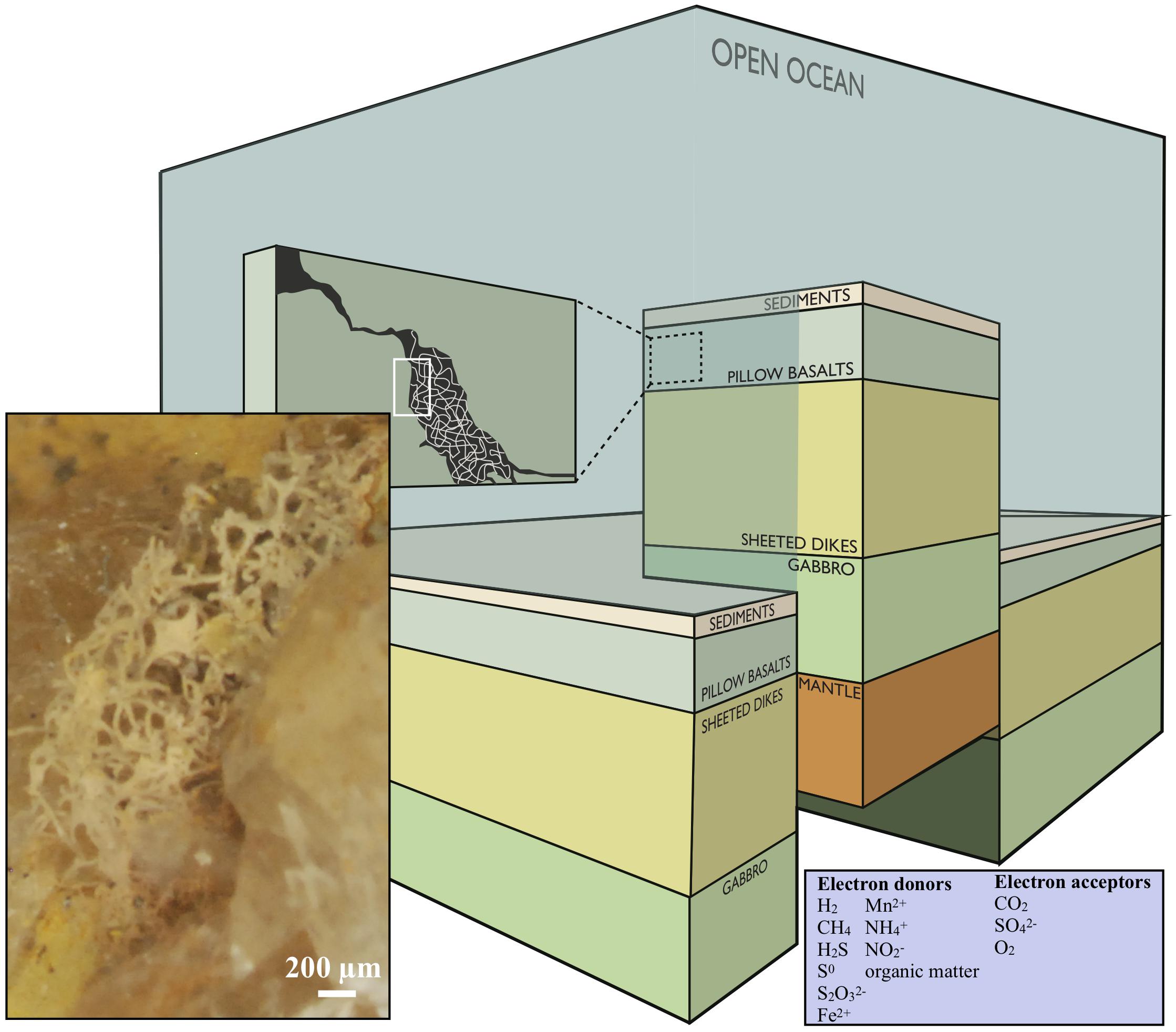
Figure 1. Conceptual image modified after Ivarsson et al. (2016), showing a cross section of the oceanic crust. Fungal hyphae colonize veins and vesicles in pillow basalts and form extensive three-dimensional mycelia [this is visualized in the dark-green box marked (A) and the enclosed stereomicroscope image]. The image is under the CC BY-NC-ND license (http://creativecommons.org/licenses/by-nc-nd/4.0/).
Rock dwelling microorganisms are termed endoliths, and can be divided into sub-categories depending on their occurrence in, and interaction with, the host rock. Microorganisms that actively create habitable cavities through chemical and/or physical processes are called euendoliths. Microorganisms that colonize pre-existent fissures and cracks are called chasmoendoliths, and microorganisms that colonize pre-existent structural cavities such as vesicles in basalts are called cryptoendoliths (Golubic et al., 1981; Ivarsson et al., 2015c).
A number of reviews of the continental and the oceanic deep realm have been published, the latter usually focused on sediments (Heim, 2011; Orcutt et al., 2011; Colwell and D’Hondt, 2013). Our understanding of the deep biosphere has substantially increased since the first investigations of cells collected from oil wells in the 1920s (Bastin et al., 1926), or from the first obtained drilled cores from the seafloor (Zobell and Anderson, 1936). During the last 30 years we have experienced a steady increase in literature much due to the construction of underground facilities for, i.e., nuclear waste repository, or through the work of international deep drilling programs such as the International Continental Scientific Drilling Program (ICDP) and the Deep Sea Drilling Project (DCDP), later Ocean Drilling Program (ODP), and International Ocean Discovery Program (IODP).
The subsurface realm is a highly variable environment with steep physical and chemical gradients. Ecologically distinct, specialized macro- and micro-niches are orchestrated by geological prerequisites such as plate tectonics, and confined by geochemical conditions like the composition of hydrothermal fluids. The ecological composition of microbial populations vary depending on if they live in an ultramafic environment subjected to serpentinization, a geothermal area, or in a subseafloor basalt. In the dark subsurface, microorganisms gain energy from coupling of thermodynamically favorable redox reactions. The basis of the deep ecosystems consist of chemoautotrophs; organisms that gain energy through the oxidation of electron donating molecules, as opposed to photoautotrophs, which use solar energy. Lithoautotrophs, in turn, utilize inorganic substrates like minerals to gain energy and heterotrophs feed on the organic biomass produced by, or available in, other organisms, such as chemoautotrophic communities and their extracellular substances.
H2, CH4, H2S, S0, S2O32−, Fe2+, Mn2+, NH4+, NO2− and organic matter are the most accessible electron donors in subsurface settings, and CO2, SO42−, or O2 are the most likely electron acceptors (Figure 1, inserted box). Microbial metabolic pathways vary substantially between different types of host rock. Basalt, the most common rock type within the oceanic crust, consists of roughly 9% FeO and 0.1% MnO and S, respectively. Serpentinization (alteration of ultramafic rocks), on the other hand, gives rise to fluids poor in Fe and S but enriched in H2, CH4, and hydrocarbons, while alteration of basalt results in fluids with reduced Fe and S compounds, and less H2 and CH4 (Bach and Edwards, 2004). In basalt-hosted systems reduced Fe, Mn, and S components are, therefore, the major energy sources for microorganisms, while H2 and CH4 are the most bio-available compounds in ultra-mafic environments. This is also reflected in molecular analyses and cultivation approaches on seafloor-exposed basalt (Ivarsson et al., 2015c).
Volcanic Rocks as Microbial Habitats
Endolithic microorganisms use interconnected networks of open pore space to migrate and colonize the host rock. The upper layers of oceanic crust (Figure 1) are characterized by extensive fracturing, about 10% porosity, and permeabilities ranging between 10−12 and 10−15 m2 (Bach and Edwards, 2004; Orcutt et al., 2011). Tension release and quick cooling creates fractures with varying size and frequency, while pressure release during magma extrusion produce vesicles. As a result, subseafloor volcanic rocks are characterized by coherent micro-fracture systems in which fluids of seawater and hydrothermal solutions circulate. The oceanic igneous crust is the largest aquifer system on Earth holding a total fluid volume that corresponds to 2% of the total ocean (Fisher and Becker, 2000; Orcutt et al., 2011). Wherever pore space and fluid flow permit, microorganisms are passively transported or actively migrating through this interconnected system. The fracture walls are used for colonization and anchoring of microbial communities, and the various minerals and host rock are scavenged by lithoautotrophic microorganisms for energy.
In contrast, the continental crystalline bedrock is of low porosity. Here, interconnected networks of fractures are formed and re-activated episodically by tectonic forces, surficial pressure release, and cooling (Drake et al., 2009).
In both oceanic and continental igneous crust, the initial stages of microbial colonization are characterized by a biofilm that is laid down on the inner walls of the open pore space and usually cover the entire surface (e.g., Figure 2A). Such biofilms are formed either by fungi or by prokaryotes (Ivarsson et al., 2015c). The fungal biofilms (Figure 2B) range between 10 and 30 μm in thickness, and generally have a smooth appearance, from which fungal sporophores or hyphae protrude. As hyphae grow further, complex mycelia will form (Figures 2C,D), which sometimes fill the entire pore space. Prokaryotic biofilms are different in nature, as they form in cycles overgrowing each other, resulting in micro-scale laminated stromatolitic structures (Figures 2A–D). Bacterial biofilms are usually overgrown by fungal biofilms and mycelia that scavenge the prokaryotic biomass (Ivarsson et al., 2015a).
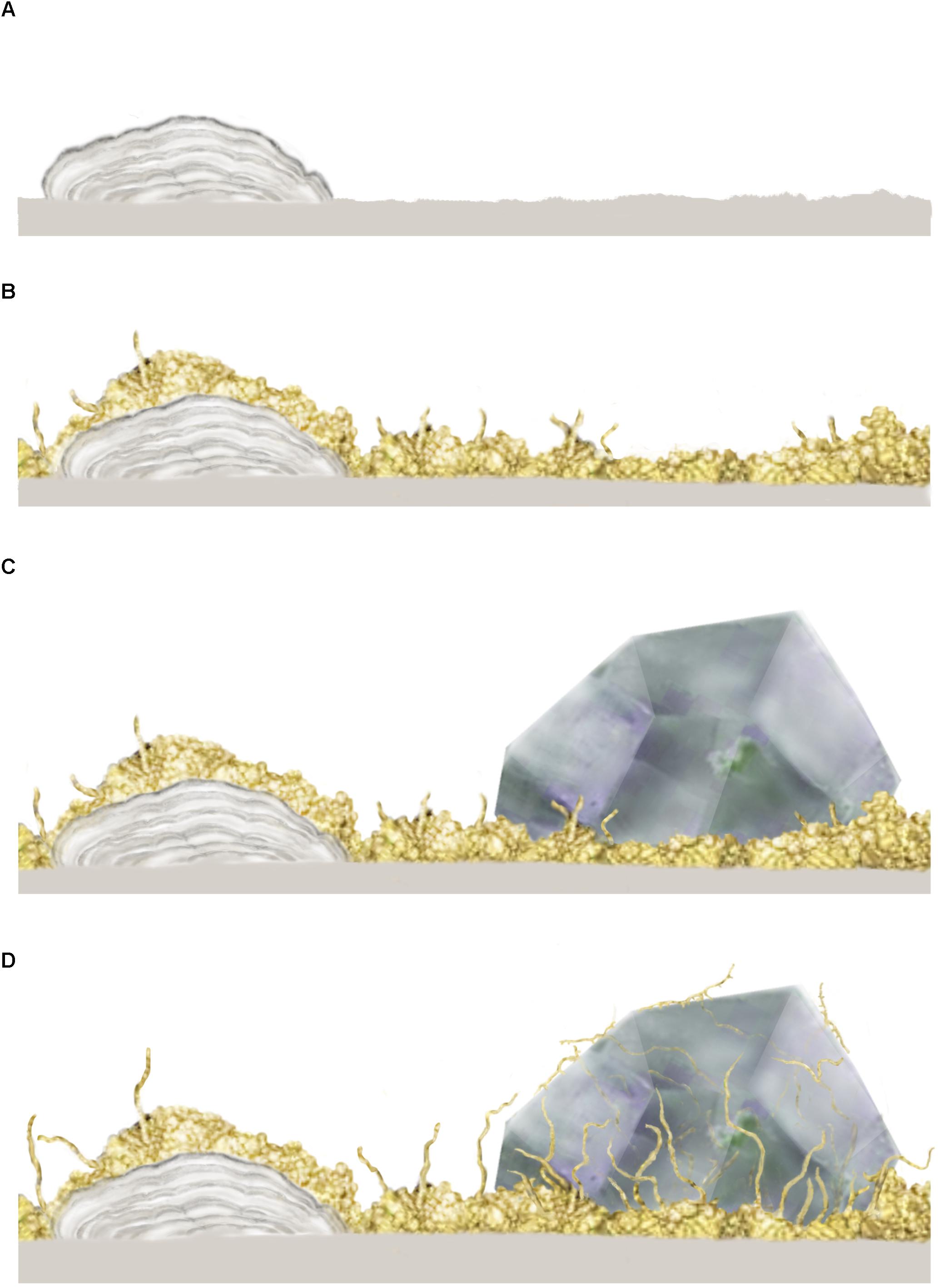
Figure 2. Conceptual image from Ivarsson et al. (2015c) showing the stepwise colonization of prokaryotic and fungal biofilms in an open crack formed in subsurface pillar basalt. (A) Initial establishment of a prokaryotic biofilm, presumably consisting of iron-oxidizing prokaryotes, which form iron, manganese and silica rich laminated crusts, or microstromatolites. (B) A heterotrophic fungal biofilm is established, over the prokaryotic base film, where fungal filaments protrude and form complex mycelia within the open pore spaces. (C) Secondary minerals in the form of zeolites form within with the fungal biofilm which eventually becomes partly buried. (D) Partly buried fungal filaments bore into a zeolite crystal, presumably as a survival strategy or for trophic reasons. The copyright holder has approved re-publishing and the image is under the terms of the Creative Commons Attribution License.
The microbial communities are clearly influenced by the physical nature of their habitats, since host rock and secondary mineralizations control the habitable space. At the same time, the microorganisms shape their own habitable environment by active bio-mediated weathering. Fungal hyphae frequently dissolve and penetrate secondary minerals (Figure 2D) like zeolites and carbonates, forming tunnel-like cavities (Bengtson et al., 2014; Ivarsson et al., 2015a; Drake et al., 2017). Carbonates have also been shown to dissolve passively by the growth of microstromatolites since the formation of iron oxides results in a lowered ambient pH (Bengtson et al., 2014). In contrast, fossilization of microbial consortia mediates mineralization of iron oxides and clays and will eventually contribute to the filling of pore space.
Micro-Fossils in Subseafloor Volcanic Rocks
Ichnofossils
Conspicuous granular and tubular cavities in volcanic glass are a widespread phenomenon in the oceanic crust, often attributed to microbial activity and etching (Furnes et al., 2008; Staudigel et al., 2008). These etch-marks are trace fossils, correctly referred to as ichnofossils (McLoughlin et al., 2007, 2009), after Greek ikhnos which translates to trace or track.
Already in 1986 Ross and Fisher (1986) reported etched grooves in glass shards from a Miocene tephra, which they compared to fungal borings in carbonate grains. During the 1990s, observations of granular and tubular microstructures were made in volcanic glass of drilled and dredged samples from the ocean floor (Thorseth et al., 1992, 1995; Furnes and Staudigel, 1999). Their biogenic origin were argued based on four criteria: (1) enrichments of C, N, and P in association with the etched cavities (2) C isotope values indicative of biologic activity (Furnes et al., 2001) (3) the presence of partially fossilized and encrusted microbial cells in the cavities, with forms and sizes matching those of the granular and tubular etch-marks, and (4) presence of nucleic acids and DNA from both bacteria and archaea in close association with the etch-marks (Giovannoni et al., 1996; Thorseth et al., 2001).
The direction of ichnofossils is perpendicular to a glass surface and inward into the glass. The granular type is made up of micron-sized, near-spherical cavities etched into the glass and later filled with authigenic minerals such as clays and Fe-oxides/hydroxides. The granular ichnofossils usually occur in clusters and are irregularly distributed on both sides of a fracture. The tubular ichnofossils consists of smoothly curved tubes, with varying morphologies including branching, spiraling, or segmented textures. Diameters are normally between one to a few microns, and ten to hundreds of microns in length. The traces usually occurs in assemblages irregularly distributed with respect to the opposing side of any cracks and are commonly associated with granular cavities. A comprehensive photographic atlas of the ichnofossils has been produced by Fisk and McLoughlin (2013).
The biogenicity of ichnofossils has lately been challenged, especially ichnofossils in ancient ophiolites and greenstone belts (Schopf, 2006; Grosch and McLoughlin, 2014). Alternative abiotic formation processes have been suggested involving the remains of fluid inclusion trails, radiation damage trails, or ambient inclusion trails (AITs) (Lepot et al., 2009; McLoughlin et al., 2010). One major issue is that the microorganisms responsible for the production of these structures have not yet been identified in modern micro-borings. Despite the on-going debate, establishing biogenicity of ichnofossils in volcanic glass is crucial for understanding early life on Earth but also since they may be potential biosignatures on Mars (McLoughlin et al., 2007, 2010; Sapers et al., 2014).
Fossilized Microorganisms
Encrusted or fossilized coccoidal microorganisms have been observed in dredged samples of seafloor-exposed basalt (Thorseth et al., 2003), and in drilled subseafloor basalt (Ivarsson et al., 2015c). Fossilized filamentous microorganisms have been identified in veins and vesicles in ocean floor basalts (Ivarsson et al., 2015c), and in ophiolites of Devonian age (Peckmann et al., 2008; Eickmann et al., 2009). They are both attributed to an endolithic lifestyle, using the walls of the open pore space for anchoring and subsequent outwards growth into the open voids. The fossils either occur as single microbial or fungal filaments or, more often, in large groups, filling the entire pore space of a vein system (Figure 1, inserted box, Figures 2, 3A–D). Their morphology can be highly varied, comprising curvi-linear segmented and branched filaments that may form complex mycelium-like networks. Sometimes they are characterized by central swellings, a turgid appearance or bean shaped structures. The diameters vary excessively, ranging between 2 and ∼30 μm with lengths between 10 μm and <1 mm. The thinnest filaments often have the most basic morphologies, resembling simple bacterial trichomes. The thicker filaments on the other hand, can vary in complexity, and usually have conspicuous septa-like segmentation (Ivarsson et al., 2015c).
The fossilized microorganisms comprise crypto-, chasmo-, and euendoliths and they can be divided into two main types, depending on preservation; (1) microorganisms enclosed and preserved in vein-filling minerals, commonly carbonate, or (2) fossilized communities mineralized and preserved directly into an open pore space (thus not entombed in secondary minerals). The first type consists of live microorganisms that have been instantly petrified in secondary mineral phases due to hydrothermal activity or alteration of the crust. They are usually permineralized by poorly crystalline clay phases and iron oxides with a high organic content, including elevated carbon levels, phosphates, hydrocarbons, lipids and occasionally even chitin (Ivarsson et al., 2012, 2015c). The second type, consist of microorganisms fossilized and mineralized in open systems where organic matter are easily oxidized and scavenged by other microorganisms. Thus, the second fossil-type rarely contains organics and are usually mineralized by a clay phase and/or iron oxides, similar to that of (1) above.
Microbial colonization occurs relatively early after cooling of the volcanic rock, presumably while fluid-flow is still active, and prior to substantial formation of secondary minerals. Colonization is initiated by the formation of a pioneer-type biofilm lining the pore space interiors (Figure 2A), which can be seen today as a mineralized crust of various hematite, hematite-carbon, and clay layers (Ivarsson et al., 2013, 2015a; Bengtson et al., 2014). The mineralized biofilm can be either smooth or botryoidal in appearance due to assimilated spherical and cell-like aggregates (Figures 3E,F). Filamentous structures protrude from the basal film into the open pore space, either solely, or more commonly in great abundance, and form complex, mycelium-like networks. Thus, the fossils are always rooted at the fracture- or vesicle-wall, where they became permineralized upon death (Figure 3G).
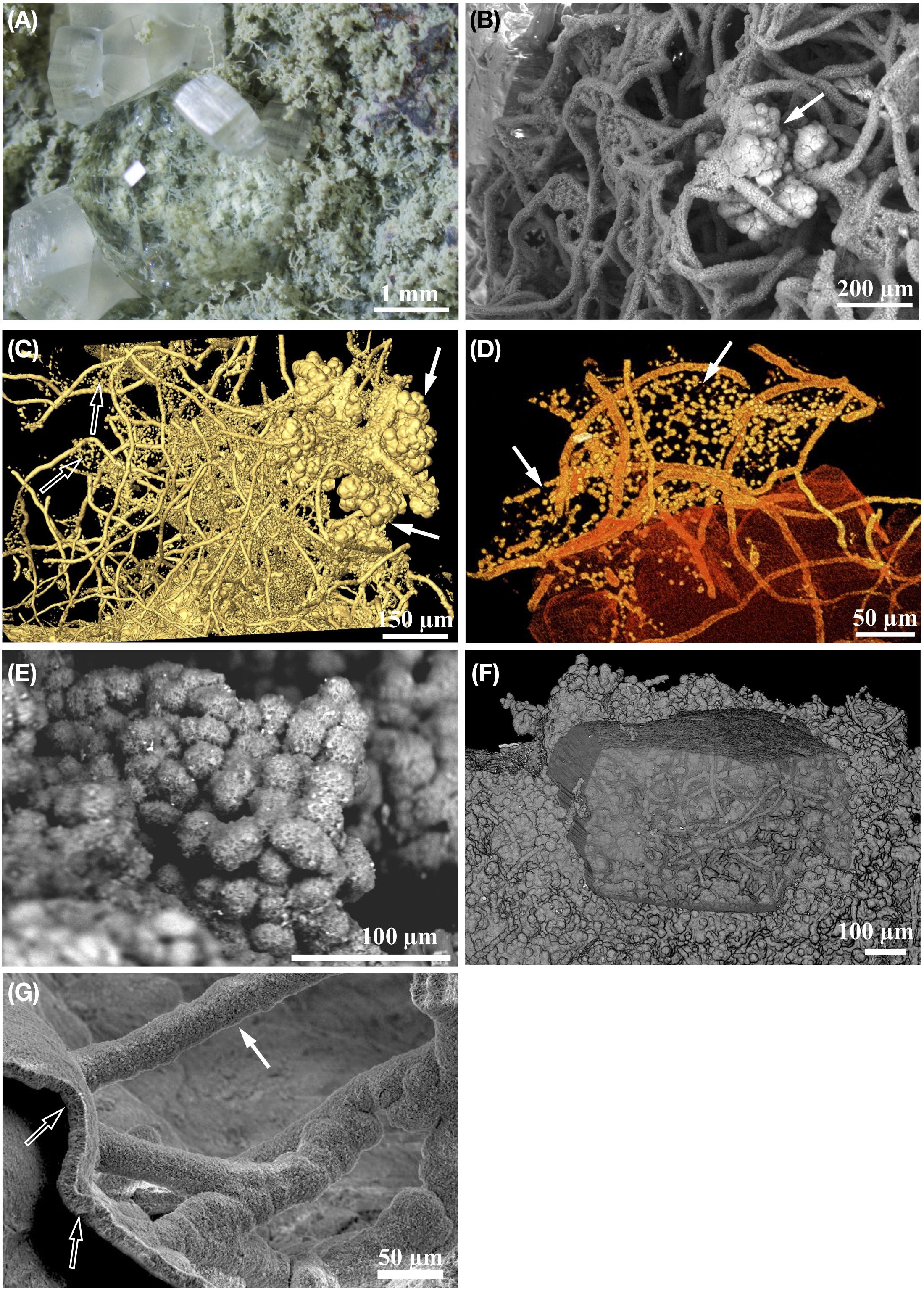
Figure 3. Eukaryotic and prokaryotic fossils found in deep subsurface basaltic habitats. Panels (A,E) are ODP sample 197-1205-34R-5 from Nintoku Seamount. Panel (F) is sample 197-1205-34R-5, 33 also from Nontoku Seamount. Panels (B,D) are ODP sample 197-1206-4R-2 from Koko Seamount, and (C) ODP sample 197-1206-4R-2 from Koko Seamount. Panel (G) is ODP sample 197-1204B-16R-01, 145 from Detroit Seamount. (A) Stereomicroscope image of one side of an open fracture that has mechanically been split open. The fracture wall is covered by fossilized fungal mycelium mineralized by clays, which becomes partly buried by secondary zeolite mineralization. (B) Backscatter electron micrograph (ESEM-BSE) showing the symbiotic relationship between clay permineralized fungal mycelia and cauliflower-like microstromatolites (arrow) from subsurface basalt. (C) Microtomographic (SRXTM) surface rendering showing a symbiotic relationship between three-dimensional fungal mycelium, globular microstromatolites (filled arrow) and unicellular prokaryotes suspended in a cobweb-like manner between the hyphae (hollow arrow). (D) Partly translucent microtomographic (SRXTM) volume rendering of unicellular prokaryotes (arrow) suspended in cobweb-like layers, or sheets, in between fungal hyphae. (E) Backscatter electron micrograph (ESEM-BSE) showing clay permineralized globular yeast cells in subsurface basalt. (F) Partly translucent microtomographic (SRXTM) volume rendering of a fossil fungal biofilm consisting of fungal hyphae and globular yeast-cells. Note that the biofilm has been partly overgrown by zeolite. (G) Backscatter electron micrograph (ESEM-BSE) showing clay permineralized fungal biofilm and attached hyphae.
Filamentous fossils in the deep crust, have almost exclusively been interpreted as the remains of fungal hyphae, and when found in complex extensive networks, as fungal mycelia. They commonly have characteristic fungal morphologies such as repetitive septa, anastomoses between branches, a central pore and, though rarely, chitin in the cell walls (Ivarsson et al., 2012, 2015c). Associated conglomerates of coccoidal fossils with diameters of ∼10 μm have been recognized as yeast-like growth states (Figures 3E,F) and transitions between hyphae- and yeast-like stages are common. The fungal fossils also contain sporophores, fruiting bodies and resting structures like sclerotia. Based on morphological traits and the presence of chitin, fungi fossils from the Emperor Seamounts in the Pacific Ocean were interpreted as Ascomycetes or stem-group Dikarya (Ivarsson et al., 2012). Other fossilized fungi collected at the Vesteris Seamount, the Greenland Basin, were interpreted as Zygomycetes based on the presence of zygospores and various morphological stages of the Zygomycetes reproduction cycle (Ivarsson et al., 2015d). Molecular studies of extant fungi in subseafloor igneous crust are rare with the exception of one fungal isolate from drill cores from North Pond, Mid-Atlantic Ridge. The isolate was affiliated to the genus Exophiala of the order Chaetothyriales (Hirayama et al., 2015). Eight yeast-like fungal species belonging to Ascomycetes and Basidiomycetes were isolated from seafloor-exposed basalt at Vailulu’u Seamount, Pacific Ocean (Connell et al., 2009). Fungal strains of Ascomycetes and Basidiomycetes were also reported from vent fluids at the Lost City, the Mid-Atlantic Ridge (López-García et al., 2007), and have been isolated from marine sediments (Orsi et al., 2013a,b) including some of the deepest sediments on Earth at the Mariana Trench (Takami et al., 1997). Chytridiomycota has been detected in hydrothermal vents (Le Calvez et al., 2009) and methane seeps (Nagahama et al., 2011). Thus, the fungal diversity seems to be high in both marine sediments and in subseafloor-exposed basalts, with representatives from all major fungal divisions.
A close ecological association between heterotrophic fungi and chemoautotrophic prokaryotes has been identified in fossilized material. The symbiotic nature of this relationship may be a prerequisite for eukaryotic colonization and persistence in the subseafloor crust (Bengtson et al., 2014; Ivarsson et al., 2015c,a). Fossilized fungal mycelia have been shown to function as a framework on which syngenetic prokaryotic communities grow. Microstromatolitic Frutexites with a characteristic cauliflower-like appearance are often seen attached to hyphae, while cell-like structures forming suspended sheeths of strings in between hyphae may be affiliated with iron-oxidizing bacteria, such as Pyrodictium or Euryarchaeon SM1 (Figures 3C,D). Microstromatolites (Frutexites), have also been observed lining the walls of veins and vesicles, often in close association with fungal hyphae. Frutexites usually consist of laminated iron, or occasional manganese, oxides and silica-rich phases (Figure 4). The overall interpretation is that they are the result of iron- or manganese-oxidizing bacterial activity (Bengtson et al., 2014; Ivarsson et al., 2015c,a). Bacterial biofilms with Frutexites can be overgrown by fungal biofilms and mycelia that graze on the prokaryotic biomass, thus enabling fungal colonization of oligotrophic subseafloor voids (Ivarsson et al., 2015a).
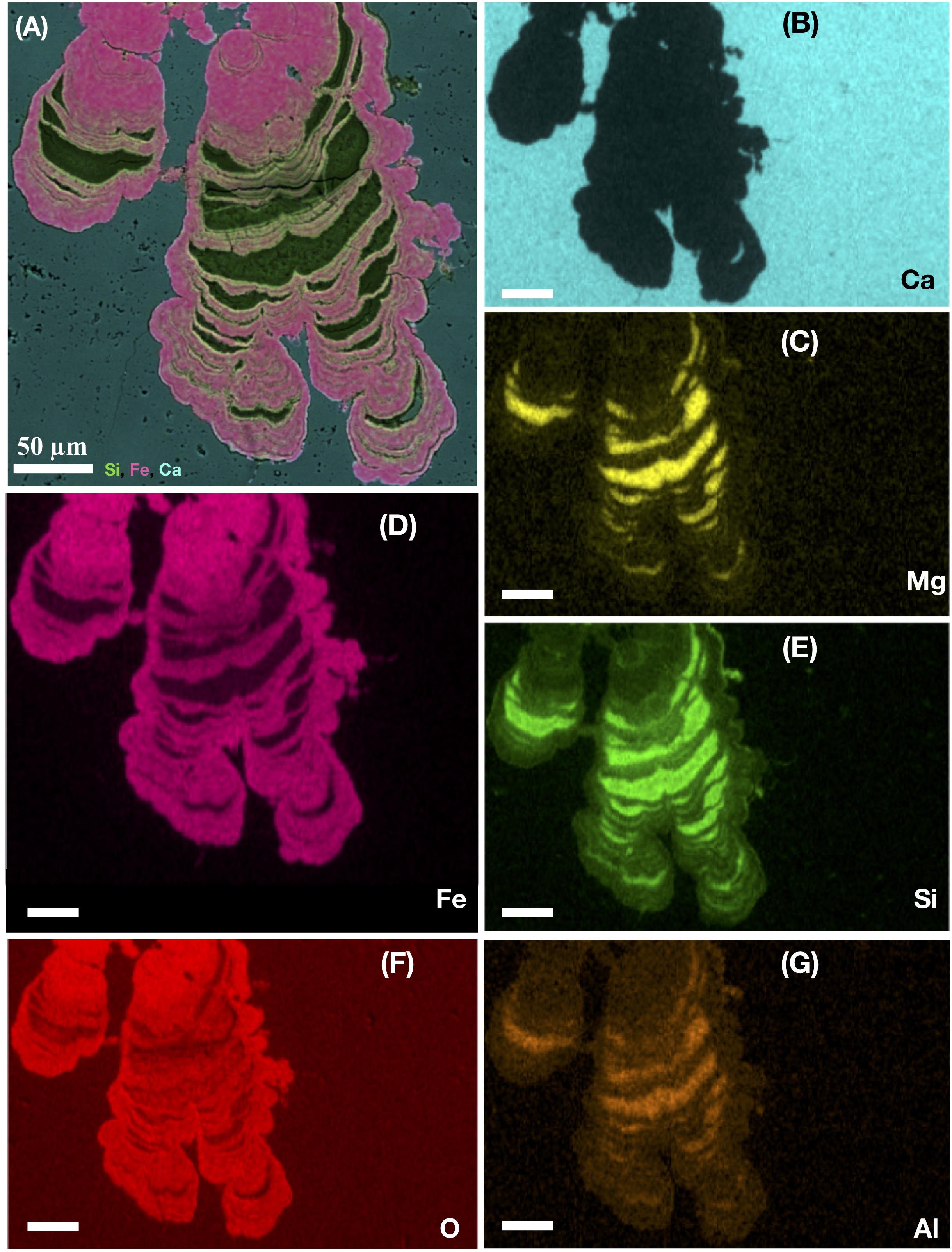
Figure 4. Ocean drilling program sample 197-1204A-7R4, 1 from Detroit Seamount. EDS-elemental map of a microstromatolite shown in cross section. The large image (A) represents a layered map of a laminated subseafloor microstromatolite from the Emperor seamount, Pacific Ocean. The layered map indicates the presence of silica (green), Iron (pink), and Ca (light blue). The following images show (B) the single-distribution of Ca (light blue), (C) Mg (yellow), (D) Fe (pink), (E) Si (green), (F) O (red), and (G) Al (orange) within the stromatolite. All scale bars are 50 μm.
The chemical and mineralogical composition of fossilized microorganisms in deep crustal habitats is often dominated by a poorly crystalline mix of Si, Fe, Al, Mg, and C with minor amounts of Na, K, Ti, and Ca, usually corresponding to smectite or montmorillonite-like clays (Ivarsson et al., 2015c), chamosite and illite (Peckmann et al., 2008). The clay permineralization is probably a result of microbial clay authigenesis (Peckmann et al., 2008). While filament exteriors are permineralized by clays, the interiors are usually characterized by iron oxides/oxyhydroxides (Bengtson et al., 2014; Ivarsson et al., 2015c,a). The carbon content of the fossils, ranging from ∼10 to 50 wt % C, varies greatly both between closely associated filaments as well as within the same filament. When the organic content is high, it may contain the presence of phosphates, hydrocarbons and lipids (Ivarsson et al., 2015c). Fossils have also successfully been stained by propidium iodide (PI), a dye that binds to cells with damaged cell membranes and traces of DNA (Ivarsson et al., 2008).
The fossilization process can be described as a transition in which the primary organic material of the organisms matures to a carbonaceous material prior to final mineralization by clays and Fe-oxides (Ivarsson et al., 2018). The negatively charged carbonaceous material attracts positively charged Si, Al, Mg, Fe (and minor Na, and Ca) cations dissolved in the fluids, which initiates subsequent adsorption and clay mineralization. Eventually, this can lead to complete mineralization by clays and Fe-oxides. Such a fossilization process is supported by the presence of partly mineralized fungal hyphae in deep granite settings (Drake et al., 2017). The fungal-precipitated clay may furthermore be altered to chlorite, if the fossils are subjected to metamorphosis (Bengtson et al., 2017).
The enrichment of C and the high degree of organic remains present in microbial fossils may be due to the distribution of elements within the fossils. Silicification is known to preserve microbial morphologies and cell content to some extent (Toporski et al., 2002). Metal cations, on the other hand, inhibit the autolysis (self-destruction of cells) and may thus preserve dead bacterial cells (Leduc et al., 1982). Ferric ions have been suggested to inhibit autolysis of bacterial cells and result in intact cellular preservation (Ferris et al., 1988). Iron is also known to form complexes, and stabilize organic compounds over geological time scales (Parenteau and Cady, 2010; Lalonde et al., 2012). Therefore, the high iron content of fossilized microorganisms in deep oceanic crust is most likely responsible for the high concentration of C and organic remains, such as lipids and chitin, found within many fossils.
The fossils in deep crystalline basement is not an exception and are, as all microfossils, tested against biogenicity criteria to exclude a possible abiotic explanation (Ivarsson, 2006). Filamentous biomorphs are known to form in laboratory experiments (Garcia-Ruiz et al., 2003), but still today, there are no reports of abiotic morphological equivalents produced within geological and geochemical conditions similar to that of subseafloor basalts. Microstromatolites occur on a morphological and structural continuum from bacterially mediated “shrubs” to abiotically precipitated dendrites (Chafetz and Guidry, 1999). The structures at the biological end of the spectrum are morphologically identical to Frutexites found in subseafloor environments (Bengtson et al., 2014), but it is important to stress that all fossil findings needs to be individually tested for biogenicity.
Martian Conditions
The most abundant rock type on Mars is mafic in composition with a volcanic origin, similar to terrestrial basalts (McSween and Treiman, 1998; Squyres et al., 2004; Poulet et al., 2009; Edwards et al., 2017). Most Martian meteorites have a distinct range in chemistry and are characterized by relatively Fe-rich, alkali-poor, undifferentiated basaltic rocks (Musselwhite et al., 2005; McSween et al., 2009). In situ investigations by Mars rovers have indicated a wider range in chemistry, showing a Fe-rich basaltic signature but with a more alkali-rich composition (McSween and Ruff, 2006; McSween et al., 2009). In Pre-Noachian and Noachian times, the martian basalts were subject to circulating groundwater. In places, they were covered by standing sea-like water-bodies, which permitted water percolation and fluid–rock interactions similar to ocean-covered basalts on Earth (Mustard et al., 2008; Ehlmann et al., 2009; Fassett and Head, 2011; Carter et al., 2013; Ehlmann and Edwards, 2014; Grotzinger et al., 2015; Goudge et al., 2016). Succeeding the Noachian eon, the “wet period” of Mars ended (Westall et al., 2015). However, parts of the martian bedrock were at times exposed to, and circulated by water due to ice melting associated with volcanic eruptions and meteorite impacts, occasional melting of ice at depths, and seasonal ice melting (Laskar et al., 2002; Tanaka et al., 2003; Velbel, 2012; Tornabene et al., 2013). Even though the martian surface was dry and cold the martian subsurface was subject to groundwater flow (Osinski et al., 2013; Grotzinger et al., 2015; Yen et al., 2017). Since the composition and alteration of martian basalts is similar to terrestrial counterparts (McSween and Ruff, 2006; Mustard et al., 2008; Ehlmann et al., 2009; McSween et al., 2009; Fassett and Head, 2011; Carter et al., 2013; Ehlmann and Edwards, 2014; Grotzinger et al., 2015; Goudge et al., 2016) it is reasonable to assume that any potential carbon-based life forms living in the deep martian rocks, would have the same geochemical and energetic prerequisites as life in basalts on Earth, and would be fossilized in a similar manner. If we assume the metabolic pathways and mode of life to be similar to that of life on Earth, the fossil record of volcanic rocks on Earth provide a valid analog to a potential martian fossil record.
A Fossil Record on Mars
Establishing an atlas of fossils in volcanic rocks on Earth seems a viable strategy for the search of fossils in martian volcanic rocks. However, it should be pointed out that the means to the search and detection of fossils on Mars is hugely different from that of Earth. Most analytical work on the terrestrial microfossil record is being done in laboratories while the fossils on Mars will be investigated, to a high degree, in situ. This means that the analytical accuracy and resolution on Mars differs by magnitudes to that of the Earth. The instruments of the Mars mission payload sets the boundaries for what is detectable and possible to analyze. Thus, when establishing a microfossil atlas, the resolution and analytical capabilities of the payload instruments needs to be considered. If the resolution is not high enough it might be necessary to formulate a strategy to identify macro-accumulations of fossilized microorganisms (millimeter sized microbial communities) and/or common mineral phases of fossilization. Later Mars sample return missions may increase the chances to perform more detailed micro-analytical investigations but are instead restricted by the limited number of samples (McLennan et al., 2012).
To finalize a microfossil atlas for Mars missions, the fossil record of volcanic rocks on Earth should be investigated and described as detailed as possible. We need to enhance the current knowledge of fossilized microorganisms in deep igneous rock and in deep time. The ambition should be to establish a fossil record from the present and backward, in order to trace microbial components of the deep biosphere in time. This will involve samples from different subsurface and subseafloor settings; using samples from mines, subsurface research facilities and drill cores from IODP and ICDP. New analytical approaches need to be tested and evaluated to be able to extract as much paleobiological information as possible from fossils. This knowledge could be used for in situ detection on Mars, but also for investigations of returned samples. We suggest that fossil samples here on Earth are investigated by microanalytical and microimaging instrumentation, simulating a sample return scenario. In situ measurements of detailed microbial morphology, combined with chemical mapping and detection, as well as characterization of biomarkers indicative of life should be developed, tested and evaluated. All with the aim to produce firm protocols for handling of future Mars sample return missions.
In a second step, the acquired knowledge needs to be approached in such a way that it is possible to repeat on Mars, and thus adapted for Martian conditions and payload. Parameters such as camera resolution, minimum point detection by instrumentation and selection of the most prominent rock sample must be considered. Aspects like geological context, microbial morphology, mineralogy, chemical composition, fossilization conditions and the presence of biomarkers should also be taken into consideration in a final evaluation.
The current scientific aims in deep biosphere research can thus be summarized as follows:
• Establish a fossil record in volcanic rocks on Earth ranging from present to the Archaean. What microorganisms are most easily preserved? What geochemical conditions control fossilization? How is the fossil record altered over time?
• Can we detect and select samples of biological interest with the instrumentation available on the Mars 2020 rover and ExoMars? Is the resolution of the payload instruments enough? What parameters should protocols for biosignature detection be based on; mineralogy, textures, organic compounds?
• What would be the most ideal approach for Mars sample return? What samples should be targeted for sample return? What Earth-based methods are most suitable?
To address these aims and formulate an exploration strategy for microfossils in volcanic rocks we need to consider:
(1) Optical assessment and macro-studies of samples (simulation of mars rover conditions).
(2) In situ detection and characterization of mineralogy and biomolecules.
(3) In situ detection of isotopes as biomarkers.
(4) Ex situ microimaging of martian samples on Earth.
(5) Establishing a microfossil atlas of volcanic rocks.
Optical Assessment
The first step in the identification of possible in situ biosignatures, will be to optically recognize textures with a possible biological origin. Focus here should be on fossilized microbial colonies in open veins and vesicles, easily accessible for rover instrumentation. The NASA Mars mission 2020 will be equipped with 23 cameras, of which most cameras are for landing, driving and landscape monitoring, thus visualizing large geological features (Farley and Williford, 2017). Of specific interest for high-resolution investigations such as these, are the cameras MASTCAM-Z, SUPERCAM, PIXL, and WATSON on SHERLOC. MASTCAM-Z is a camera pair that provide color images and videos, three-dimensional stereo images, equipped with a powerful zoom lens. It has a pixel scale of ∼0.5 mm/pixel to ∼0.15 mm/pixel at 2 m, which is sufficient to resolve ∼1 mm features in the near-field (robotic arm workspace) and ∼3–4 cm features approximately 100 m away. SUPERCAM and PIXL are both combined instrument set-ups including cameras with resolution similar to MASTCAM-Z1.
SHERLOC (The Scanning Habitable Environments with Raman and Luminescence for Organics and Chemicals) is an arm-mounted, Deep UV (DUV) resonance Raman and fluorescence spectrometer capable of analysis at <100 μm spot size. The laser is integrated to an autofocusing/scanning optical system, and co-boresighted to a context imager, which at the most typical use has a resolution of 15 μm/pixel corresponding to a spatial resolution of approximately 50–60 μm (Kenneth Williford, personal communication). In addition to the combined spectroscopic and macro imaging component, SHERLOC also includes a near-field-to-infinity imaging component called WATSON (Wide Angle Topographic Sensor for Operations and eNgineering), which enables science operations and imaging. WATSON captures the larger context images for the very detailed information that SHERLOC collects.
ExoMars Panoramic camera (PanCam) (Coates et al., 2012, 2017; Cousins et al., 2012; Yuen et al., 2013) will perform digital terrain mapping for the mission rover. It is a suite that consists of a fixed-focus, wide-angle, stereoscopic, color camera pair (WAC) complemented by a focusable, high-resolution, color camera (HRC). PanCam will enable characterization of the geological environment, from panoramic (tens of meters) to millimeter scale, at the sites the rover will visit. It will be used for detailed investigations of outcrops, rocks, and soils, and to image samples collected by the drill before they are delivered to the analytical laboratory. For more detailed investigations, the ExoMars is equipped with the close-up imager (CLUPI) that will obtain high-resolution color images for exploration of the depositional environments, but also detailed textural features in the form of morphological biosignatures such as biolamination (Josset et al., 2017). CLUPI will be mounted on the drill box and be equipped with several viewing modes, allowing the study of outcrops, the fines produced during drilling, and also imaging collected samples in high resolution before delivering them to the analytical laboratory. Imaging resolution varies with distance to target; it is, 8 μm/pixel at 11.5 cm distance with view area 2.0 cm × 1.4 cm, 39 μm/pixel at 50 cm distance with view area 10 cm × 7 cm, and 79 μm/pixel at 100 cm distance with view area 21 cm × 14 cm (Vago et al., 2017).
PanCam and CLUPI can be used simultaneously to visualize outcrops and rocks at progressively higher resolution. PanCam and CLUPI can also view drilling operations. As the drill penetrates into the ground, fines will be collected of which CLUPI can obtain high resolution images at 39 and 13 (drill lowered) μm/pixel (Josset et al., 2017). Furthermore, borehole wall data can be obtained by the Ma_MISS IR spectrometer (Mars multispectral imager for subsurface studies) (De Angelis et al., 2013; De Sanctis et al., 2017), integrated in the drill tip. The PanCam HRC and CLUPI are capable of imaging samples placed by the drill on the Core Sample Transport Mechanism (CSTM), and finally CLUPI can obtain high-resolution images (∼8 μm/pixel) of the sample.
Resolution of the payload cameras controls the possible detection limit. Therefore, because of size limitations, identification and characterization of individual filaments and cell structures will not be possible with the current optical instrumentation. This also includes the detection of narrow ichnofossils and similar microbial traces. Instead, an approach involving structures <50–60 μm have to be developed. This includes fossilized communities with an internal morphological variation that may be detectable, or with a diagnostic mineralogy/geochemistry. Examples of possible targets are three-dimensional fungal mycelium with diameters exceeding 1 mm (Figure 5A). These mycelium often form a network of filamentous and/or yeast cells that grow perpendicular and markedly from a mineral or host rock surface. Another target can for example be extensive microstromatolites with well-defined and visible laminations that vary in color due to differences in mineralogy.
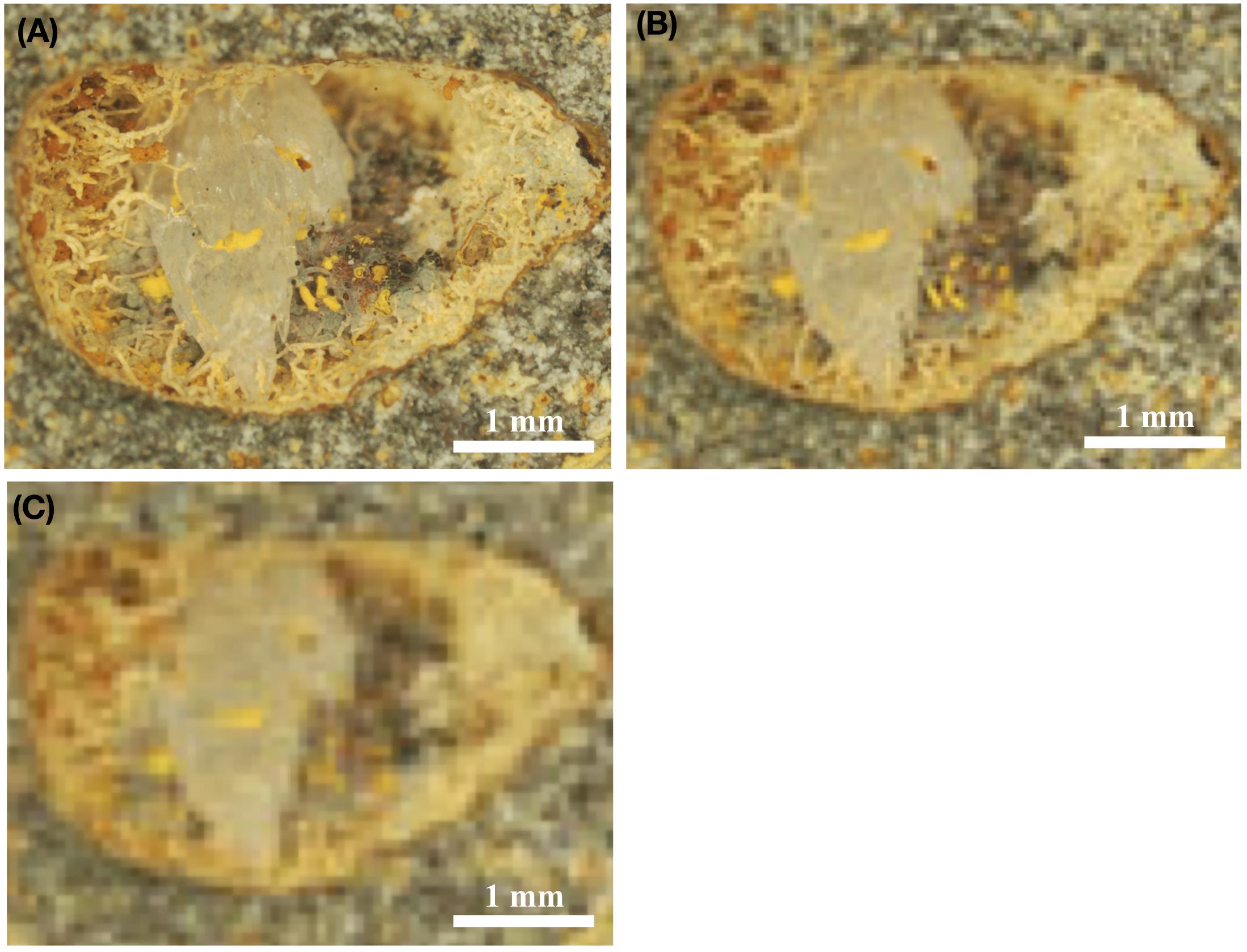
Figure 5. Stereomicroscopic images of fossilized fungal mycelia in a subseafloor basalt-vesicle (A–C). ODP sample 197-1206A- 4R-2, 0, from Koko Seamount. Panel (A) shows the original, high resolution, stereomicroscopic image, and Panel (B) shows how the same image would look with a resolution of 8 μm/pixel, which is the highest possible resolution by ESA’s ExoMars 2020 mission. In turn, (C) illustrates how this image would look using the optical resolution of 16 μm/pixel, which represent the possible resolution using NASAs’s Mars 2020 mission equipment. Panels (B,C) thus represents purposefully pixelated images of (A) to show how the same sample would look, with either the cameras used by ExoMars 2020 (B) or NASA Mars 2020 missions (C).
Figure 5A shows a vesicle ∼5 mm in diameter, with fossilized fungal mycelium lining the walls. Microstromatolitic Frutexites, ∼30–100 μm in diameter, have grown and become fossilized on the fungal hyphae as orange or yellow accumulations. Figure 5B is modified to 8 μm/pixel, the resolution of CLUPI on ExoMars, indicating a decrease in resolution compared with the original image. It is, however, still high enough resolution for details like hyphae and even Frutexites to be identified. Figure 5C is modified to 15 μm/pixel, the best possible resolution of SHERLOCK on NASAs Mars mission 2020 (Kenneth Williford, personal communication), and shows a substantially decreased resolution and image quality, which makes identification of individual hyphae or microstromatolites difficult. It is, however, possible to see that the vesicle in whole carries interesting morphological features along the vein walls that should be analyzed in situ for mineralogy and organic content or targeted for later Mars sample return.
In situ Detection of Mineralogy and Biomolecules
During mineralization and fossilization of microorganisms, organic remains are incorporated and bound by mineral phases, especially iron oxides and clays. If the fossils only experience moderate taphonomic alterations during diagenesis, the organic content will remain more or less unaltered and easy to detect by in situ measurements. The organic content can be used as a strong argument when proving biogenicity of a putative microfossil or biomineral, but also, if complex biomolecules are preserved as biomarkers, to constrain the taxonomic affiliation of a fossil organism. Lipids and proteins, for instance, can be preserved during long periods of time and are usually specific for organismal affinity.
As discussed above, the optical system on SHERLOC is coupled with a Deep UV (DUV) resonance Raman and fluorescence spectrometer, utilizing a 248.6-nm DUV laser with <100 μm spot size. Deep UV-induced native fluorescence is very sensitive to condensed carbon and aromatic organics, with a detection-capacity at, or below, 10–6 w/w (1 ppm) at <100 μm. SHERLOC’s deep UV resonance Raman therefore enables detection and classification of aromatic and aliphatic organics with sensitivities of 10–2 to below 10–4 w/w at <100 μm spatial scales. Additionally, the deep UV Raman allows detection and classification of aqueous minerals with grain sizes below 20 μm.
The fungal hyphae and the Frutexites in Figure 5 have been analyzed by Raman and shown to contain carbonaceous matter (Bengtson et al., 2014). The spot size for these analysis were ∼1–3 μm, thus the analysis were done with higher precision than the instrumentation coupled to SHERLOCK will be. However, with a <100 μm spot size the overall mineralogy will be analyzed and give a composite spectrum including clays, iron oxides and carbonaceous matter, thus a comparable sample on Mars would be possible to analyze and detect organic remains within.
Pancam on ExoMars is coaligned with an IR-spectrometer (ISEM) that enables identification of mineral phases in bulk rock- or soil samples at a distance. As mentioned above, the Ma_MISS is a miniaturized IR spectrometer integrated in the drill tool for imaging of the borehole wall as the drill is being operated. Ma_MISS has a spectral range of 0.4–2.2 mm with spatial resolution of 120 μm. CLUPI, however, is not coupled with any instruments for chemical detection. When samples have been selected by Pancam, ISEM, and CLUPI, they are deposited in the core sample transport mechanism (CSTM) where PanCam HRC and CLUPI can image the sample during a few minutes. After the imaging exercise, the CSTM move the sample into the analytical laboratory where a rock crusher produce particulate matter having a Gaussian size distribution, with a median value of 250 μm. The implications of this maneuver is that any identified morphological structures of interest will be destroyed, and correlation between morphological biosignatures and molecular fossils will thus thereafter be impossible.
In situ Detection of Isotopes
Microorganisms continually interact with their environment through their metabolism, which itself fosters mineralization and may favor fossilization. Secondary minerals resulting from metabolic activity are commonly of intermediate to high specificity for the actual metabolism. Detailed in situ analysis at a micro scale can be performed on mineralized fossils and associated biominerals by laser ablation inductively coupled mass spectrometry (LA-ICP-MS). Such instruments are optimized to analyze isotopic contents of individual microorganisms and microbial associations on a micro-scale. Examining the elemental and isotopic composition of the mineralized microbial remains, will allow a constraint of primary and secondary metabolites and bioavailable energy sources, information that can be used to determine the biological affinity of microfossils. For example, δ13C values of authigenic calcite will indicate the presence, or past presence of anaerobic methane oxidation, or methanogenesis, while δ34S values in pyrite will indicate potential fractionation due to bacterial sulfate reduction (Drake et al., 2015, 2017). Isotopic investigations will therefore help to constrain metabolic pathways and can clarify the microbial involvement in the cycling of elements between rocks, microorganisms and water.
Miniaturized laser ablation ionization mass spectrometry (LIMS)-time-of-flight mass spectrometer for space missions capable of performing in situ isotope measurements of minerals at planet surfaces in vacuum have been developed and tested, but not included, in the payloads of ExoMars or NASAs mars mission 2020 (Wiesendanger et al., 2018). Isotope measurement thus have to be targeted for the subsequent Mars sample return mission.
Ex situ Microimaging of Martian Samples on Earth
Drill cores and other kind of samples retrieved from Mars offer the opportunity of a considerably more detailed chemical and morphological sample investigation. As long as the payload instrumentations enable sampling of material with a high potential for biosignature preservation, the chances of identifying and characterizing morphological and/or molecular fossils in a piece of rock is therefore higher by magnitudes in laboratories on Earth compared to in situ on the surface of Mars.
Microimaging techniques are central in all microfossil investigations, in order to resolve microbial morphology, variations in composition and relation to host rock and associated mineral phases. Methods and instrumentation can vary depending on access to equipment and facilities. For example, synchrotron-based X-ray tomographic microscopy (SRXTM) is a non-invasive technique that allows the study of rock and fossil structures in 3 dimensions, which has been decisive in the identification of deep-biosphere fossils of different ages (Ivarsson et al., 2012, 2013; Bengtson et al., 2014, Bengtson et al., 2015c,a). Recent identification of fungus-like fossils from 2.4 Ga vesicular basalts obtained by SRXTM (Bengtson et al., 2017) were instrumental in distinguishing pore-dwelling cryptoendoliths from rock-boring euendoliths. The physical interaction between microorganisms and minerals represented by either biomineralization or bioerosion structures have thus been successfully visualized and studied by SRXTM (Bengtson et al., 2014; Ivarsson et al., 2015c,a). So far, most tomographic work on deep biosphere fossils have been executed at the micro-tomography beamline TOMCAT at the Swiss Light Source synchrotron facility, Paul Scherrer Institute, Switzerland. The TOMCAT beamline has provided tomographic datasets with micron-sized sample resolution, which has resulted in increased insights into the spatial correlation between microbes and vein filling minerals as well as their association with vesicle/fracture surfaces within the basalt. In addition, new facilities such as the synchrotron Max IV in Lund, in particular the beamlines DanMAX and NanoMAX, will have the capacity to resolve nanometer-scale features, which will open a new path toward the investigation of cryptic microfossils and their ultrastructural features.
For the examination of volcanic biosignatures and fossils, initial mineralogical and fossil identification should be performed using a combination of light and fluorescence microscopy, scanning electron microscopy (SEM) coupled with energy dispersive X-ray spectroscopy (EDS), X-ray diffraction (XRD), and X-ray fluorescence (XRF). Further detection and characterization of organic material in fossils may be targeted by Raman spectroscopy and Time-of-Flight secondary ion mass spectrometry (ToF-SIMS) (Ivarsson et al., 2015c).
The above analysis should also be complemented by bulk analyses. The extraction/characterization of biomarkers may be performed using gas chromatography (GC), coupled gas chromatography/mass spectrometry (GC/MS), and coupled high-pressure liquid chromatography/mass spectrometry (HPLC/MS). For compound-specific stable carbon isotope compositions, gas chromatography-isotope ratio monitoring-mass spectrometry (GC-irm-MS) can be used.
Toward the Establishment of a Microfossil Atlas for Mars Exploration
To establish an atlas of volcanic-based microfossils for the upcoming Mars missions, we need to enhance the current knowledge of fossilized microorganisms in deep igneous rock and in deep time, as reviewed above. The ambition should be to establish a fossil record from the present and backward, to trace microbial components of the deep biosphere in time, and to establish an improved framework for the timing of major evolutionary lineages. The main goal is to apply this knowledge to future exploration missions on Mars, with the possibility of identifying extra-terrestrial fossilized microorganisms from volcanic habitats.
Summary
Based on the contents of this review and the research covered herein, we propose to establish a microfossil atlas, covering all known aspects of the ecology of volcanic habitats on Earth, including prevalent information about trace and body fossils of prokaryotic and eukaryotic nature. To accomplish this, we need the combining results from all working areas as reviewed above; including information about microbial morphology, organic microfossil-content (biomarkers) and elemental and isotopic content of igneous-dwelling fossils and their associated biominerals. A first rough classification will primarily be based on morphology, but biomarkers and relevant isotopic fractionations will be added to this scenario to enhance the classification and to make it taxonomically robust. A combination of biomarkers and isotopes will make it possible to discriminate between groups of microorganisms based on metabolisms such as, for example, methanogens and methanotrophs. Species discrimination is made possible by the presence and detection of different lipids and δ13C values within fossil microorganisms and/or associated biominerals (in the case of δ13C) (Drake et al., 2015). Ultimately, however, the volcanic microfossil atlas will be classified based on taxonomy. Rough discriminations between prokaryotes and eukaryotes will be possible, as will hopefully also more precise classifications, down to class-level.
The current payloads of NASAs Mars 2020 and the ExoMars missions are capable of analyzing structures <60 μm, possibly somewhat smaller. Therefore, both missions will be able to target larger biogenic structures from volcanic rocks, such as mm-sized mineralized fungal mycelia, or larger microstromatolites in open vesicles. The ExoMars cameras with a resolution of 8 μm/px has a greater chance of identifying small features and individual hyphae, as seen in Figure 5B, but in turn, the NASA mission has the possibility of collecting samples for later ex situ investigation on Earth- a resolution of 15 μm/pixel may therefore be sufficient to resolve enough features to select samples with a high probability of containing biosignatures. Our hope with establishing a volcanic microfossil atlas is that it may act as a complement to more established sedimentary-based fossil charts by providing a robust assessment of microbial diversity in the igneous oceanic crust. Apart from providing general guidelines for microfossil studies on Earth, we envision the atlas to specifically aid in the search for relevant target-sites for planetary missions, such as the NASA Mars mission 2020 and ExoMars.
Author Contributions
MI designed and implemented the initial study, contributed with manuscript conception and manuscript writing. TS contributed with manuscript conception, manuscript writing, and ESEM-EDS analysis. D-TC contributed with manuscript conception and writing. All authors aware of, read and approved, the submitted version of the manuscript.
Funding
This work was funded by the Swedish Research Council (Contracts No. 2007-4483, 2012-4364, 2013-4290, and 2017-04129), a Villum Investigator Grant to Donald Canfield No. 16518, and a grant from Carl Tryggers Foundation (CTS 18: 167), to MI and TS.
Conflict of Interest Statement
The authors declare that the research was conducted in the absence of any commercial or financial relationships that could be construed as a potential conflict of interest.
Acknowledgments
The authors wish to thank Stefan Bengtson and Veneta Belivanova, Swedish Museum of Natural History, for production of SRXTM, ESEM and optical microscopy images. Federica Marone, Swiss Light Source and Paul Scherrer Institute, Switzerland for synchrotron imaging and reconstruction. Anna Neubeck, Uppsala University, for contributing with the original image for Figure 1, and Pollyanna von Knorring, Swedish Museum of Natural History, for illustrating Figure 2.
Footnotes
References
Bach, W., and Edwards, K. J. (2004). Iron and sulphide oxidation within the basaltic ocean crust: implications for chemolithoautotrophic microbial biomass production. Geochim. Cosmochim. Acta 67, 3871–3887. doi: 10.1016/s0016-7037(03)00304-1
Bastin, E. S., Greer, F. E., Merritt, C. A., and Moulton, G. (1926). The presence of sulphate reducing bacteria in oil field waters. Science 63, 21–24. doi: 10.1126/science.63.1618.21
Bengtson, S., Ivarsson, M., Astolfo, A., Belivanova, V., Broman, C., Marone, F., et al. (2014). Deep-biosphere consortium of fungi and prokaryotes in Eocene sub-seafloor basalts. Geobiology 12, 489–496. doi: 10.1111/gbi.12100
Bengtson, S., Rasmussen, B., Ivarsson, M., Muhling, J., Broman, C., Marone, F., et al. (2017). Fungus-like mycelial fossils in 2.4 billion-year-old vesicular basalt. Nat. Ecol. Evol. 1:0141. doi: 10.1038/s41559-017-0141
Carter, J., Poulet, F., Bibring, J.-P., Mangold, N., and Murchie, S. (2013). Hydrous minerals on Mars as seen by the CRISM and OMEGA imaging spectrometers: updated global view. J. Geophys. Res. 118, 831–858. doi: 10.1029/2012JE004145
Chafetz, H. S., and Guidry, S. A. (1999). Bacterial shrubs, crystal shrubs, and ray-crystal shrubs: bacterial vs. abiotic precipitation. Sed. Geol. 126, 57–74. doi: 10.1016/s0037-0738(99)00032-9
Coates, A. J., Griffiths, A. D., Leff, C. E., Schmitz, N., Barnes, D. P., Josset, J.-L., et al. (2012). Lunar PanCam: adapting ExoMars PanCam for the ESA Lunar Lander. Planet Space Sci. 74, 247–253. doi: 10.1016/j.pss.2012.07.017
Coates, A. J., Jaumann, R., Griffiths, A. D., Leff, C. E., Schmitz, N., Josset, J.-L., et al. (2017). The PanCam instrument for the ExoMars rover. Astrobiology 17, 511–541. doi: 10.1089/ast.2016.1543
Cockell, C. S. (2014a). “The subsurface habitability of terrestrial rocky planets: mars,” in Microbial Life of the Deep Biosphere, eds J. Kallmeyer and D. Wagners (Berlin: DeGruyter), 225–260.
Cockell, C. S. (2014b). Trajectories of martian habitability. Astrobiology 14, 182–203. doi: 10.1089/ast.2013.1106
Colwell, F. S., and D’Hondt, S. (2013). Nature and extent of the deep biosphere. Rev. Miner. Geochem. 75, 547–574. doi: 10.2138/rmg.2013.75.17
Connell, L., Barrett, A., Templeton, A., and Staudigel, H. (2009). Fungal diversity associated with an active deep sea volcano: vailulu’u Seamount, Samoa. Geomicrobiol. J. 26, 597–605. doi: 10.1080/01490450903316174
Cousins, C. R., Gunn, M., Prosser, B. J., Barnes, D. P., Crawford, I. A., Griffiths, A. D., et al. (2012). Selecting the geology filter wavelengths for the ExoMars panoramic camera instrument. Planet. Space Sci. 71, 80–100. doi: 10.1016/j.pss.2012.07.009
De Angelis, S., De Sanctis, M. C., Ammannito, E., Di Iorio, T., Carli, C., Frigeri, A., et al. (2013). VNIR spectroscopy of mars analogues with the ExoMars-Ma_miss instrument. Memorie della Societa Astronomica Italiana Supplement 26, 121–127.
De Sanctis, M. C., Altieri, F., Ammannito, E., Biondi, D., De Angelis, S., Meini, M., et al. (2017). Ma_MISS on ExoMars: mineralogical characterization of the martian subsurface. Astrobiology 17, 612–620. doi: 10.1089/ast.2016.1541
Des Marais, D. J. (2010). Exploring Mars for evidence of habitable environments and life. Proc. Am. Phil. Soc. 154, 402–421.
Drake, H., Åström, M. E., Heim, C., Broman, C., Åström, J., Whitehouse, M., et al. (2015). Extreme 13C-depletion of carbonates formed during oxidation of biogenic methane in fractured granite. Nat. Comm. 6:7020. doi: 10.1038/ncomms8020
Drake, H., Ivarsson, M., Bengtson, S., Heim, C., Siljeström, S., Whitehouse, M., et al. (2017). Anaerobic consortia of fungi and sulfate reducing bacteria in deep granite fractures. Nat. Comm. 8:55. doi: 10.1038/s41467-017-00094-6
Drake, H., Tullborg, E.-L., and Page, L. (2009). Distinguished multiple events of fracture mineralisation related to far-field orogenic effects in Paleoproterozoic crystalline rocks, Simpevarp area, SE Sweden. Lithos 110, 37–49. doi: 10.1016/j.lithos.2008.12.003
Edwards, P. H., Bridges, J. C., Wiens, R., Anderson, R., Dyar, D., Fisk, M., et al. (2017). Basalt-trachybasalt samples in Gale Crater, Mars. Meteor. Planet. Sci. 52, 2391–2410.
Ehlmann, B. L., and Edwards, C. S. (2014). Mineralogy of the martian surface. Annu. Rev. Earth Planet. Sci. 42, 291–315. doi: 10.1146/annurev-earth-060313-55024
Ehlmann, B. L., Mustard, J. F., Murchie, S. L., Bibring, J. P., Meunier, A., Fraeman, A. A., et al. (2011). Subsurface water and clay mineral formation during the early history of Mars. Nature 479, 53–60. doi: 10.1038/nature10582
Ehlmann, B. L., Mustard, J. F., Swayze, G. A., Clark, R. N., Bishop, J. L., Poulet, F., et al. (2009). Identification of hydrated silicate minerals on Mars using MRO-CRISM: geologic context near Nilli Fossae and implications for aqueous alteration. J. Geophys. Res. Planets 114:E00D08.
Eickmann, B., Bach, W., Kiel, S., Reitner, J., and Peckmann, J. (2009). Evidence for cryptoendolithic life in Devonian pillow bas-alts of Variscan orogens, Germany. Palaeogeogr. Palaeoclimatol. Palaeoecol. 283, 120–125. doi: 10.1016/j.palaeo.2009.09.006
Farley, K. A., and Williford, K. H. (2017). Seeking signs of life, and more: NASA’s Mars 2020 mission. EOS 98. doi: 10.1029/2017EO066153
Fassett, C. I., and Head, J. W. (2011). Sequence and timing of conditions on early Mars. Icarus 211, 1204–1214. doi: 10.1016/j.icarus.2010.11.014
Ferris, F. G., Fyfe, W. S., and Beveridge, T. J. (1988). Metallic ion binding by Bacillus subtilis: implications for the fossilization of microorganisms. Geology 16, 153–157.
Fisher, A. T., and Becker, K. (2000). Channelized fluid flow in oceanic crust reconciles heat-flow and permeability data. Nature 403, 71–74. doi: 10.1038/47463
Fisk, M., and McLoughlin, N. (2013). Atlas of alteration textures in volcanic glass from the ocean basins. Geosphere 9, 317–341. doi: 10.1130/ges00827.1
Furnes, H., McLoughlin, N., Muehlenbachs, K., Banerjee, N., Staudigel, H., Dilek, Y., et al. (2008). “Oceanic pillow lavas and hyaloclastites as habitats for microbial life through time-A review,” in Links Between Geological Processes, Microbial Activities and Evolution of Life, eds Y. Dilek, H. Furnes, and K. Muehlenbachs (Berlin: Springer), 1–68. doi: 10.1007/978-1-4020-8306-8_1
Furnes, H., Muehlenbachs, K., Tumyr, O., Torsvik, T., and Xenophontos, C. (2001). Biogenic alteration of volcanic glass from the Trodoos ophiolite. Cyprus J. Geol. Soc. Lond. 158, 75–84. doi: 10.1089/ast.2014.1259
Furnes, H., and Staudigel, H. (1999). Biological mediation in ocean crust alteration: how deep is the deep biosphere? Earth Planet. Sci. Lett. 166, 97–103. doi: 10.1016/s0012-821x(99)00005-9
Garcia-Ruiz, J. M., Hyde, S. T., Carnerup, A. M., Christy, A. G., van Kranendok, M. J., and Welham, N. J. (2003). Self-assembled silica-carbonate structures and deoetction of ancient microfossils. Science 302, 1194–1197. doi: 10.1126/science.1090163
Giovannoni, S. J., Fisk, M. R., Mullins, T. D., and Furnes, H. (1996). Genetic evidence for endolithic microbial life colonizing basaltic glass-seawater interfaces. Proc. Ocean Drill. Prog. Sci. Res. 148, 207–214.
Golubic, S., Friedmann, I., and Schneider, J. (1981). The lithobiontic ecological niche, with special reference to microorganisms. J. Sed. Res. 51, 475–478.
Goudge, T. A., Fassett, C. I., Head, J. W., Mustard, J. F., and Aureli, K. L. (2016). Insights into surface runoff on early Mars from paleolake basin morphology and stratigraphy. Geology 44, 419–422. doi: 10.1130/g37734.1
Grosch, E. G., and McLoughlin, N. (2014). Reassessing the biogenicity of Earth’s oldest trace fossil with implications for biosignatures in the search for early life. PNAS 111, 8380–8385. doi: 10.1073/pnas.1402565111
Grotzinger, J. P., Gupta, S., Malin, M. C., Rubin, D. M., Schieber, J., Siebach, K., et al. (2015). Deposition, exhumation, and paleoclimate of an ancient lake deposit. Gale Crater, Mars. Science 350:aac7575. doi: 10.1126/science.aac7575
Heim, C. (2011). “Terrestrial deep biosphere,” in Encyklopedia of Geobiology, eds J. Reitner and V. Thiel (Berlin: Springer), 871–876. doi: 10.1007/978-1-4020-9212-1_65
Hirayama, H., Abe, M., Miyazaki, J., Sakai, S., Nagano, Y., and Takai, K. (2015). Data report: cultivation of microorganisms from basaltic rock and sediment cores from the North Pond on the western flank of the Mid-Atlantic Ridge. IODP Expedition 336. Proc. Int. Oc. Drill. Prog. 336, doi: 10.2204/iodp.proc.336.204.2015
Ivarsson, M. (2006). Advantages of doupbly polished thin sections for the study of microfossils in volcanic rock. Geochem. Trans. 7:5.
Ivarsson, M., Bengtson, S., Belivanova, V., Stampanoni, M., Marone, F., and Tehler, A. (2012). Fossilized fungi in subseafloor Eocene basalts. Geology 40, 163–166. doi: 10.1111/gbi.12100
Ivarsson, M., Bengtson, S., Drake, H., and Francis, W. (2018). Fungi in deep subsurface environments. Adv. Appl. Microbiol. 102, 83–116. doi: 10.1016/bs.aambs.2017.11.001
Ivarsson, M., Bengtson, S., and Neubeck, A. (2016). The igneous oceanic crust – Earth’s largest fungal habitat? Fung. Ecol. 20, 249–255. doi: 10.1016/j.funeco.2016.01.009
Ivarsson, M., Bengtson, S., Skogby, H., Belivanova, V., and Marone, F. (2013). Fungal colonies in open fractures of subseafloor basalt. Geo Mar. Lett. 33, 233–243. doi: 10.1007/s00367-013-0321-7
Ivarsson, M., Bengtson, S., Skogby, H., Lazor, P., Broman, C., Belivanova, V., et al. (2015a). A fungal-prokaryotic consortium at the basalt-zeolite interface in subseafloor igneous crust. PLoS One 10:e014016. doi: 10.1371/journal.pone.0140106
Ivarsson, M., Broman, C., Gustafsson, H., and Holm, N. G. (2015b). Biogenic Mn-oxides in subseafloor basalts. PLoS One 10:e0128863. doi: 10.1371/journal.pone.0128863
Ivarsson, M., Holm, N. G., and Neubeck, A. (2015c). “The deep biosphere of the subseafloor igneous crust,” in Trace Metal Biogeochemsitry and Ecology of Deep-Sea Hydrothermal Vent Systems, eds L. L. Demina and S. V. Galkin (Berlin: Springer), 143–166. doi: 10.1007/698_2015_5014
Ivarsson, M., Peckmann, J., Tehler, A., Broman, C., Bach, W., Behrens, K., et al. (2015d). Zygomycetes in vesicular basanites from Vesteris Seamount, Greenland Basin – a new type of cryptoendolithic fungi. PLoS One 10:e01333368. doi: 10.1371/journal.pone.01333368
Ivarsson, M., Lindblom, S., Broman, C., and Holm, N. G. (2008). Fossilized microorganisms associated with zeolite-carbonate interfaces in sub-seafloor hydrothermal environments. Geobiology 6, 155–170. doi: 10.1111/j.1472-4669.2007.00139.x
Josset, J.-L., Westall, F., Hofmann, B. A., Spray, J., Cockell, C., Kempe, S., et al. (2017). The Close-Up Imager onboard the ESA ExoMars Rover: objectives, description, operations, and science validation activities. Astrobiology 17, 595–611. doi: 10.1089/ast.2016.1546
Lalonde, K., Mucci, A., Ouellet, A., and Gélinas, Y. (2012). Preservation of organic matter in sediments promoted by iron. Nature 483, 198–200. doi: 10.1038/nature10855
Laskar, J., Levrard, B., and Mustard, J. F. (2002). Orbital forcing of the martian polar layered deposits. Nature 419, 375–377. doi: 10.1038/nature01066
Le Calvez, T., Burgaud, G., Mahé, S., Barbier, G., and Vandenkoornhuyse, P. (2009). Fungal diversity in deep sea hydrothermal ecosystems. Appl. Environ. Microbiol. 75, 6415–6421. doi: 10.1128/AEM.00653-09
Leduc, M., Kasra, R., and Van Neijenoort, J. (1982). Induction and control of the autolytic system of Escherichia coli. J. Bacteriol. 152, 26–34.
Lepot, K., Philippot, P., Benzerara, K., and Wang, G.-Y. (2009). Garnet-filled trails associated with carbonaceous matter mimicking microbial filaments in Archean basalt. Geobiology 7, 393–402. doi: 10.1111/j.1472-4669.2009.00208.x
López-García, P., Vereshchaka, A., and Moreira, D. (2007). Eukaryotic diversity associated with carbonates and fluid-seawater interface in Lost-City hydrothermal field. Environ. Microbiol. 9, 546–554. doi: 10.1111/j.1462-2920.2006.01158.x
Magnabosco, C., Lin, L.-H., Dong, H., Bomberg, M., Ghiorse, W., Stan-Lotter, H., et al. (2018). The biomass and biodiversity of the continental subsurface. Nat. Geosci. 11, 707–717. doi: 10.1038/s41561-018-0221-6
McLennan, S. M., Sephton, M. A., Allen, C., Allwood, A. C., Barbieri, R., Beaty, D. W., et al. (2012). Planning for Mars Returned Sample Science: Final Report of the MSR End-to-End International Science Analysis Group (E2E-iSAG). Available at: https://www.liebertpub.com/doi/10.1089/ast.2011.0805 (accessed April 02, 2012).
McLoughlin, N., Brasier, M. D., Wacey, D., Green, O. R., and Perry, R. S. (2007). On biogenicity criteria for endolithic microborings on early Earth and beyond. Astrobiology 7, 10–26. doi: 10.1089/ast.2006.0122
McLoughlin, N., Furnes, H., Banerjee, N. R., Muehlenbachs, K., and Staudigel, H. (2009). Ichnotaxonomy of microbial trace fossils in volcanic glass. J. Geol. Soc. Lond. 166, 159–169. doi: 10.1144/0016-76492008-049
McLoughlin, N., Staudigel, H., Furnes, H., Eichmann, B., and Ivarsson, M. (2010). Mechanisms of microtunneling in rock substrates – Distinguishing endolithic biosignatures from abiotic microtunnels. Geobiology 8, 245–255. doi: 10.1111/j.1472-4669.2010.00243.x
McMahon, S., and Parnell, J. (2014). Weighing the deep biosphere. FEMS Microbiol. Ecol. 87, 113–120. doi: 10.1111/1574-6941.12196
McSween, H. Y., and Ruff, S. W. (2006). Alkaline volcanic rocks from the Columbia Hills, Gusev crater, Mars. J. Geophys. Res. Plan. 111, 1–15.
McSween, H. Y., Taylor, G. J., and Wyatt, M. B. (2009). Elemental composition of the Martian crust. Science 324, 736–739. doi: 10.1126/science.1165871
Musselwhite, D. S., Dalton, H. A., Kiefer, W. S., and Treiman, A. H. (2005). Experimental petrology of the basaltic shergottite Yamato-980459: implications for the thermal structure of the Martian mantle. Meteorit. Planet. Sci. Arch. 41, 1271–1290. doi: 10.1111/j.1945-5100.2006.tb00521.x
Mustard, J. F., Murchie, S. L., Pelkey, S. M., Ehlmann, B. L., Milliken, R. E., Grant, J. A., et al. (2008). Hydrated silica minerals on Mars observed by the mars reconnaissance orbiter CRISM instrument. Nature 454, 305–309. doi: 10.1038/nature07097
Nagahama, T., Takahashi, E., Nagano, Y., Abdel-Wahab, M. A., and Miyazaki, M. (2011). Molecular evidence that deep-branching fungi are major fungal components in deep-sea methane cold-seep sediments. Environ. Microbiol. 13, 2359–2370. doi: 10.1111/j.1462-2920.2011.02507.x
National Research Council (2011). Vision and Voyages for Planetary Science in the Decade 2013–2022. Washington, DC: The National Academies Press, doi: 10.17226/13117
Onstott, T. C. (2016). Deep Life: The Hunt for the Hidden Biology of Earth, Mars and Beyond. Princeton, NJ: Princeton University Press.
Orcutt, B. N., Sylvan, J. B., Knab, N. J., and Edwards, K. J. (2011). Microbial ecology of the dark ocean above, at, and below the seafloor. Microbiol. Mol. Biol. Rev. 75, 361–422. doi: 10.1128/MMBR.00039-10
Orsi, W. D., Biddle, J. F., and Edgcomb, V. D. (2013a). Deep sequencing of subseafloor eukaryotic rRNA reveals active fungi across marine subsurface provinces. PLoS One 8:e56335. doi: 10.1371/journal.pone.0056335
Orsi, W. D., Edgcomb, V. D., Christman, G. D., and Biddle, J. F. (2013b). Gene expression in the deep biosphere. Nature 499, 205–208. doi: 10.1038/nature12230
Osinski, G., Tornabene, L., Banerjee, N., Cockell, C., Flemming, R., Izawa, M., et al. (2013). Impact-generated hydrothermal systems on Earth and Mars. Icarus 224, 347–363. doi: 10.1038/srep03487
Parenteau, M. N., and Cady, S. L. (2010). Microbial biosignatures in iron-mineralized phototrophic mats at chocolate pots hot springs, Yellowstone national park, United States. Palaios 25, 97–111. doi: 10.2110/palo.2008.p08-133r
Peckmann, J., Bach, W., Behrens, K., and Reitner, J. (2008). Putative cryptoendolithic life in devonian pillow basalt, rheinisches schiefergebirge, Germany. Geobiology 6, 125–135. doi: 10.1111/j.1472-4669.2007.00131.x
Poulet, F., Mangold, N., Platevoet, B., Bardintzeff, J.-M., Sautter, V., Mustard, J. F., et al. (2009). Quantitative compositional analysis of Martian mafic regions using the MEx/OMEGA reflectance data. Icarus 201, 84–101. doi: 10.1016/j.icarus.2008.12.042
Sapers, H. M., Osinski, G. R., Banerjee, N. R., and Preston, L. J. (2014). Enigmatic tubular features in impact glass. Geology 42, 471–474. doi: 10.1130/g35293.1
Schopf, J. W. (2006). Fossil evidence of Archaean life. Philos. Trans. R. Soc. B 361, 869–885. doi: 10.1098/rstb.2006.1834
Schrenk, M. O., Huber, J. A., and Edwards, K. J. (2009). Microbial provinces in the subseafloor. Ann. Rev. Mar. Sci. 2, 279–304. doi: 10.1146/annurev-marine-120308-081000
Squyres, S. W., Arvidson, R. E., Bell, J. F., Brückner, J., Cabrol, N. A., Calvin, W., et al. (2004). The Spirit Rover’s Athena science investigation at Gusev Crater, Mars. Science 305, 794–799. doi: 10.1126/science.1100194
Staudigel, H., Furnes, H., McLoughlin, N., Banerjee, N. R., Connell, L. B., and Templeton, A. (2008). 3.5 billion years of glass bioal-teration: volcanic rocks as a basis for microbial life? Earth Sci. Rev. 89, 156–176. doi: 10.1016/j.earscirev.2008.04.005
Takami, H., Inoue, A., Fuji, F., and Horikoshi, K. (1997). Microbial flora in the deepest sea mud of the Mariana Trench. FEMS Microbiol. Lett. 152, 279–285. doi: 10.1016/s0378-1097(97)00211-5
Tanaka, K. L., Skinner, J. A. Jr., Hare, T. M., Joyal, T., and Wenker, A. (2003). Resurfacing history of the Northern Plains of Mars based on geologic mapping of Mars Global Surveyor data. J. Geophys. Res. 108:8043. doi: 10.1029/2002JE00190
Thorseth, I. H., Furnes, H., and Heldal, M. (1992). The importance of microbiological activity in the alteration of natural basaltic glass. Geochim. Cosmochim. Acta 56, 845–850. doi: 10.1016/0016-7037(92)90104-q
Thorseth, I. H., Pedersen, R. B., and Christie, D. M. (2003). Microbial alteration of 0-30-Ma seafloor basaltic glasses from the Australian Antarctic Discordance. Earth Planet. Sci. Lett. 215, 237–247. doi: 10.1016/s0012-821x(03)00427-8
Thorseth, I. H., Torsvik, T., Furnes, H., and Muehlenbachs, K. (1995). Microbes play an important role in the alteration of oceanic crust. Chem. Geol. 126, 137–146. doi: 10.1016/0009-2541(95)00114-8
Thorseth, I. H., Torsvik, T., Torsvik, V., Daae, F. L., and Pedersen, R. B. (2001). Diversity of life in ocean floor basalt. Earth Planet. Sci. Lett. 194, 31–37. doi: 10.1016/s0012-821x(01)00537-4
Toporski, J. K. W., Steele, A., Westall, F., Thomas-Keprta, K. L., and McKay, D. S. (2002). The simulated silicification of bacteria – new clues to the modes and timing of bacterial preservation and implications for the search for extraterrestrial microfossils. Astrobiology 2, 1–26. doi: 10.1089/153110702753621312
Tornabene, L. L., Osinski, G. R., McEwan, A. S., Wray, J. J., Craig, M. A., Sapers, H. M., et al. (2013). An impact origin for hydrated silicates on Mars: a synthesis. J. Geophys. Res. Planet. 118, 994–1012. doi: 10.1002/jgre.20082
Vago, J. L., Westall, F., Pasteur Instrument Teams, Pasteur Landing Team, Coates, A. J., Jaumann, R., et al. (2017). Habitability on Early Mars and the Search for Biosignatures with the ExoMars Rover. Astrobiology 17,471–510.
Velbel, M. A. (2012). “Aqueous alteration in martian meteorites: comparing mineral relations in igneous-rock weathering of martian meteorites and in the sedimentary cycle of Mars,” in Sedimentary Geology of Mars, eds J. P. Grotzinger and R. E. Milliken (Tulsa: SEPM special publication), 97–117. doi: 10.2110/pec.12.102.0097
Westall, F., Foucher, F., Bost, N., Bertrand, M., Loizeau, D., Vago, J. L., et al. (2015). Biosignatures on mars: what, where, and how? Implications for the search for martian life. Astrobiology 15, 998–1029. doi: 10.1089/ast.2015.1374
Wiesendanger, R., Grimaudo, V., Moreno, P., Cedeño López, A., Riedo, A., Tulej, M., et al. (2018). Chemical and optical identification of micrometer sized 1.9 Ga old fossils with a miniature LIMS system combined with an optical microscope. Astrobiology 18, 1071–1080. doi: 10.1089/ast.2017.1780
Yen, A. S., Ming, D. W., Vaniman, D. T., Gellert, R., Blake, D. F., Morris, R. V., et al. (2017). Multiple stages of aqueous alteration along fractures in mudstone and sandstone strata in Gale Crater, Mars. Earth Planet. Sci. Lett. 471, 186–198. doi: 10.1016/j.epsl.2017.04.033
Yuen, P., Gao, Y., Griffiths, A., Coates, A., Muller, J.-P., Smith, A., et al. (2013). ExoMars Rover PanCam: autonomous and computational intelligence [application notes]. IEEE Comp. Int. Mag. 8, 52–61. doi: 10.1109/mci.2013.2279561
Keywords: deep biosphere, biosignature, astrobiology, microfossils, Mars
Citation: Ivarsson M, Sallstedt T and Carlsson D-T (2019) Morphological Biosignatures in Volcanic Rocks – Applications for Life Detection on Mars. Front. Earth Sci. 7:91. doi: 10.3389/feart.2019.00091
Received: 14 September 2018; Accepted: 11 April 2019;
Published: 01 May 2019.
Edited by:
Bradley M. Tebo, Oregon Health and Science University, United StatesReviewed by:
Kai Waldemar Finster, Aarhus University, DenmarkNancy Hinman, University of Montana, United States
Copyright © 2019 Ivarsson, Sallstedt and Carlsson. This is an open-access article distributed under the terms of the Creative Commons Attribution License (CC BY). The use, distribution or reproduction in other forums is permitted, provided the original author(s) and the copyright owner(s) are credited and that the original publication in this journal is cited, in accordance with accepted academic practice. No use, distribution or reproduction is permitted which does not comply with these terms.
*Correspondence: Magnus Ivarsson, bWFnbnVzLml2YXJzc29uQG5ybS5zZQ==