- 1Marine Sciences Laboratory – Pacific Northwest National Laboratory, Sequim, WA, United States
- 2School of Oceanography, University of Washington, Seattle, WA, United States
- 3Department of Geological Sciences, University of Florida, Gainesville, FL, United States
- 4Centro de Energia Nuclear na Agricultura, Universidade de São Paulo, Piracicaba, Brazil
- 5Department of Ecology and Environmental Science, Umeå University, Umeå, Sweden
When water bodies with unique biogeochemical constituents mix together there is potential for diverse responses by aquatic microbial communities and associated ecosystem functions. Here we evaluate bulk respiration under varying mixtures of turbid Amazon River water and two lowland tributaries—the Tapajós and Xingu rivers—based on O2 drawdown in dark rotating incubation chambers. Experiments containing 5, 17, 33, and 50% tributary water mixed with Amazon River water were performed for the Tapajós and Xingu rivers at three different rotation velocities (0, 0.22, and 0.66 m s−1) during the falling water period. Pseudo first order reaction coefficients (k′), a measure of respiration potential, ranged from −0.15 to −1.10 d−1, corresponding to respiration rates from 1.0 to 8.1 mg O2 L d−1. k′-values consistently increased with the rate of chamber rotation, and also was generally higher in the tributary-mainstem mixtures compared to pure endmembers. For both the Tapajós and Xingu rivers, the 17% mixture of tributary water yielded maximal k′-values, which were up to 2.9 and 2.2 times greater than in the tributary endmembers, respectively. The 50% mixtures, on the other hand, did not result in large increases in k′. We hypothesize that enhanced respiration potential after mixing unique water is driven, in part, by microbial priming effects that have been previously identified on a molecular level for these rivers. The results of this study suggest that there may be an optimal mixture for priming effects to occur in terms of the relative abundance of “priming” and “primed” substrates.
Introduction
Freshwater ecosystems contain a diverse mixture of material and biological organisms linked to both the landscape through which water has previously flowed and processes occurring in situ (Ward et al., 2017). These complex and dynamic biogeochemical conditions allow for rapid processing of organic matter (OM) that results in high rates of CO2 outgassing from inland waters (Raymond et al., 2013; Sawakuchi et al., 2017) and a relatively short residence time of OM (i.e., several years) relative to soils and sediments (Catalán et al., 2016). Since the recognition that large amounts of OM traveling through inland waters could not be entirely accounted for Hedges et al. (1997), Richey et al. (1980), a wide body of research has emerged seeking to understand how the cycling of OM in inland waters influences ecosystem level properties such as CO2 (Cole et al., 2007) and dissolved O2 (DO) (Holtgrieve et al., 2010; Hotchkiss and Hall, 2014), and the role of specific molecular transformations in driving these cycles (Ward et al., 2013; Riedel et al., 2016).
It is becoming increasingly recognized that in order to fully understand the role of inland waters on global biogeochemical cycles we must evaluate processes occurring along the entire continuum—from headwaters to the sea (Vannote et al., 1980; Medeiros et al., 2015; Feng et al., 2016). While comprehensive studies of river continua are becoming more widespread (e.g., Seidel et al., 2016; Duan et al., 2017), there are fairly limited environmental and experimental observations for what happens when unique water bodies converge. For example, mixing zones such as river confluences and river plumes are hypothesized to be hotspots for OM cycling due to processes such as “priming effects,” whereby the decomposition of less reactive OM is stimulated by the presence of highly reactive material such as algal exudates (Bianchi, 2011; Bianchi et al., 2015). There is currently no consensus on how priming effects influence aquatic carbon cycling, with diverse responses observed in a variety of settings (Bengtsson et al., 2018).
The aim of this study is to evaluate how OM cycling varies when algal-rich clearwater tributaries mix with the turbid Amazon River mainstem, containing an abundance of terrestrially derived OM (Seidel et al., 2015). We specifically examine microbial respiration rates, measured as the drawdown of DO in dark incubation chambers, in varying mixtures of tributary and mainstem waters. Experiments were conducted in spinning incubation chambers at various rotational velocities to replicate different mixing rates associated with river flow.
Materials and Methods
Experiments were performed in the lower Amazon River during the rising water period (February 21–28, 2016). Sampling sites included Óbidos, the furthest downstream gauging station in the Amazon River mainstem without observable tidal effects; Almeirim, which is a mainstem site roughly halfway between Óbidos and the Amazon River mouth; and two clearwater tributaries, the Tapajós and Xingu rivers (Figure 1). River discharge obtained from the Agencia Nacional de Aìguas (ANA) was 119,107 m3 s−1 at Óbidos on the day of sampling, compared to < 1,000 to m3 s−1 and 3,185 m3 s−1 for the Tapajós and Xingu rivers, respectively. Geochemical conditions including water temperature, conductivity, pH, fine suspended sediments (FSS), dissolved organic carbon (DOC), and total dissolved nitrogen (TDN) were measured in the middle of each channel at the surface and 50% depth (Table 1) according to methods described by Ward et al. (2015).
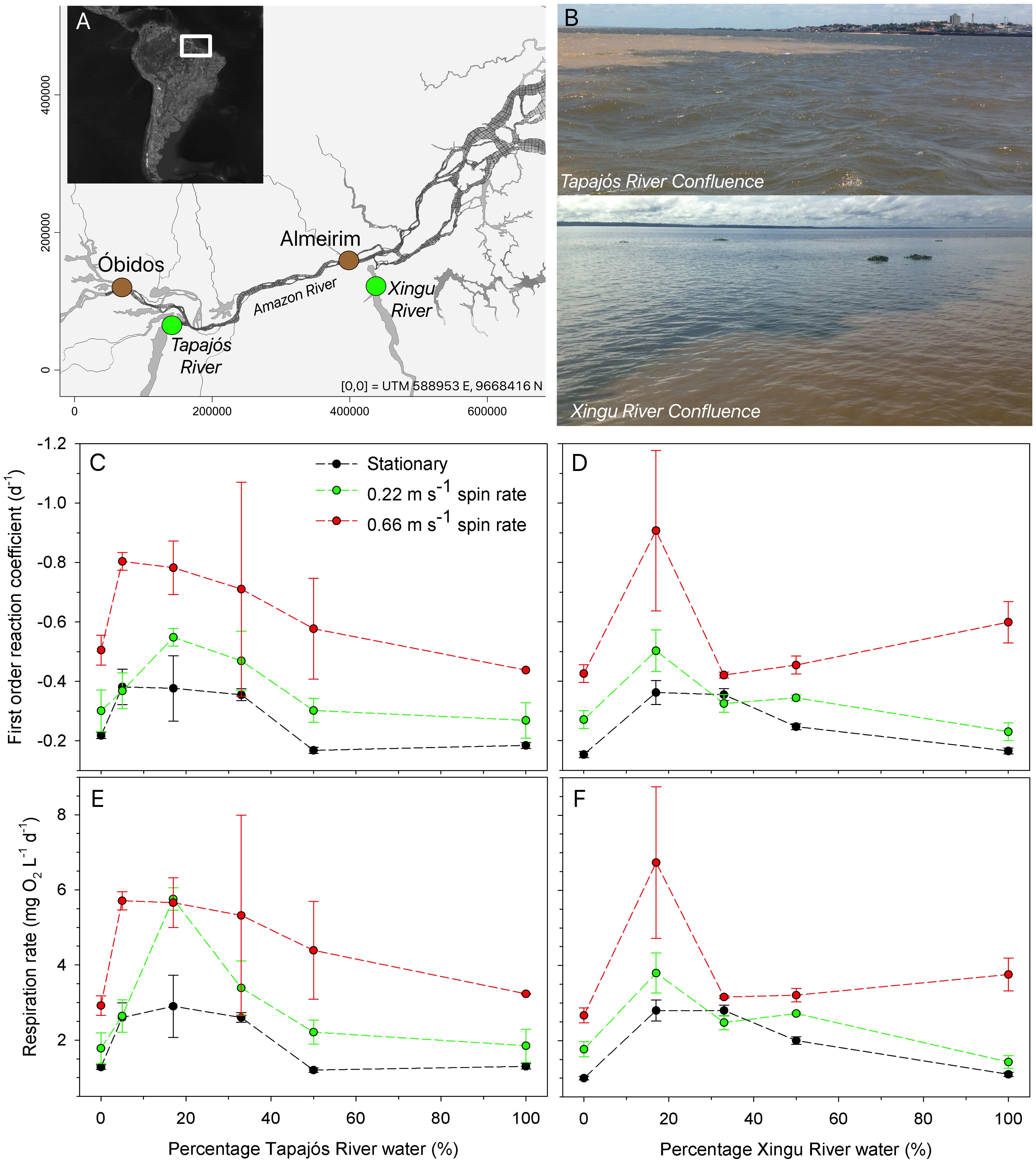
Figure 1. (A) Incubation experiments were performed using water collected from the Amazon River mainstem at Óbidos and Almeirim and in two clearwater tributaries—the Tapajós and Xingu rivers. (B) Water collected in the Amazon River mainstem was also mixed with water from the Tapajós (top) and Xingu rivers (bottom) on the days pictured. Pseudo first order reaction coefficients (k′) were measured for these different mixtures at three rotational velocities for the (C) Tapajós and (D) Xingu rivers and used to calculate respiration rates (E,F). Error bars represent ± 1 standard deviation calculated by propagating uncertainty in our calculations and variability between replicates (n = 2 for rotating chambers and n = 1 for stationary chamber).

Table 1. Initial conductivity (cond), water temperature, pH, fine suspended sediments (FSS), dissolved organic carbon (DOC), and total dissolved nitrogen (TDN) at each sampling site.
Incubation experiments were performed in custom chambers with a volume of 2.85 L, which were wrapped in reflective tape to maintain darkness. DO drawdown was monitored inside the chamber at a 30 s interval for 2–24 h using a YSI Exo 2 sonde with an optical DO probe inserted into the chamber prior to filling. To test the effects of sample disturbance on respiration, the chambers were affixed to a Marconi spin plate and rotated at the minimum and maximum available speeds—20 and 60 rpm, which is equivalent to a rotational velocity of 0.22 and 0.66 m s−1, respectively. A total of five chambers were used per experiment—two per rotational velocity and one that was held stationary on the floor of the ship. A detailed description and photos of the experimental chambers can be found in Ward et al. (2018).
To test how respiration varies when two rivers mix along their confluence, we performed experiments first using water collected from each pair of river endmembers independently—Óbidos and the Tapajós River and Almeirim and the Xingu River. In this case surface waters were collected using a Rule bilge pump with the chambers allowed to overflow ∼3 times their volume, and incubations typically lasted ∼24 h. We then performed experiments after mixing water from each endmember (in the case of the Tapajós experiment we used water collected just upstream of the city of Santarém rather than Óbidos for the sake of logistics). Experiments were performed with mixtures of 17, 33, and 50% tributary water by volume for both the Tapajós and Xingu rivers. For the Tapajós River we performed an additional experiment with 5% tributary water (95% Amazon River water). To make the mixtures we collected numerous 20 L carboys and poured these into large coolers, attempting to limit aeration while pouring. The chambers were then submerged into the cooler to fill and shaken to dislodge any bubbles. These experiments were run for 2–6 h so that more mixtures could be tested in the same day. DO drawdown was linear in all experiments regardless of incubation time.
To quantify differences in respiration in the mixtures compared to the endmembers without interference from different initial DO concentrations (Table 2) we assumed that DO consumption followed pseudo first order reaction kinetics according to Dai et al. (2006):
where [O2] is the DO concentration, t is incubation time, k is the integrated reaction coefficient for all DO consuming reactions, [M] is the concentration of bioavailable substrates, and k′ is the pseudo first order reaction coefficient assuming a constant concentration of bioavailable substrates. k′ was calculated based on the following equation and reported in d−1 units:
where A is a random constant. k′ was calculated in Microsoft Excel by performing a linear regression on natural log transformed DO data, where k′ is the slope. The linear regression fit (R2) was used to determine uncertainty, which was propagated with variability between duplicate chambers and reported as ± 1 SD. Respiration rates (mg O2 L−1 d−1) were then calculated based on initial DO concentrations according to Eq. 1. k′-values are reported for the 0.66 m s−1, 0.22 m s−1, and stationary chambers as k3x, k1x, and kStat, respectively.
Differences between k′ values and initial geochemical conditions were evaluated using unpaired t-tests for statistical significance and marginal significance with a 95 and 90% confidence interval, respectively. The correlation between k′ and initial DO was tested based on linear regression.
Results
Initial Conditions
Conductivity, pH, and FSS were significantly higher (p < 0.05) in the Amazon River mainstem (Óbidos and Almeirim) compared to the Xingu and Tapajós rivers, whereas no significant difference was observed for water temperature, DOC, and TDN (p > 0.10; Table 1). Prior to incubating, average DO concentrations in the experimental chambers were lowest at Óbidos (5.9 ± 0.1 mg L−1) and highest in the Tapajós River (7.1 ± 0.2 mg L−1; Table 2). Each site had significantly different initial DO concentrations from one another (p < 0.05) with the exception of Almeirim (6.5 ± 0.3 mg L−1) versus the Xingu River (6.3 ± 0.2 mg L−1; p > 0.05). The Xingu-Amazon mixture experiments all had elevated initial DO concentrations compared to the pure river endmembers (p < 0.05) likely due to aeration. For the Tapajós-Amazon mixture experiments, initial DO was significantly higher in all of the mixed samples compared to Óbidos (p < 0.05), but only significantly higher than the Tapajós River for the 50% mixture (p < 0.05) and not the other mixtures (p > 0.10).
First-Order Reaction Coefficients and Respiration Rates
Across all incubations k3x, k1x, and kStat varied from −0.40 to −1.10 (mean = −0.59) d−1, −0.21 to −0.85 (mean = −0.37) d−1, and −0.15 to −0.38 (mean = −0.27) d−1, respectively (Figure 1). k3x was significantly higher (i.e., more negative) than k1x and, likewise, k1x was significantly higher than kStat (p < 0.05). When multiplied by initial DO concentrations, these k′-values correspond to respiration rates of 2.6 to 8.1 (mean = 4.2) mg O2 L−1 d−1, 1.3 to 6.1 (mean = 2.0) mg O2 L−1 d−1, and 1.0 to 2.9 (mean = 2.0) mg O2 L−1 d−1 for chambers rotated at 0.66, 0.22, and 0 m s−1, respectively.
Óbidos had a 1.1 times greater k3x-value than the Tapajós River (p < 0.05) and the Xingu River had a 1.2 times greater average k1x-value than Óbidos (p < 0.10). There were no other significant differences between the endmember k′-values. For all four of the different Tapajós-Amazon mixtures combined, k3x and k1x were 1.6 and 1.8 times greater than for the pure Tapajós River water, respectively, and 1.5 and 1.6 times greater than at Óbidos (p < 0.05). kStat was also 1.5 and 1.6 times greater in the Tapajós-Amazon mixtures compared to Tapajós River and Óbidos endmembers, respectively, but the lack of duplicates resulted in statistical insignificance (p > 0.10). For all three Xingu-Amazon mixtures combined, k1x was 1.7 and 1.4 times higher than at Almeirim and in the Xingu River, respectively (p < 0.05), whereas the other rotational velocities were not statistically different than the endmembers (p > 0.10).
For both the Tapajós (p < 0.05) and Xingu (p < 0.10) rivers, the 17% mixture of tributary water yielded maximal k1x-values, which were 2.9 and 2.2 times greater than k1x in the tributary endmembers, respectively. For the Tapajós-Amazon experiments, k3x was highest in the 5% mixture with a k3x 1.8 times greater than pure Tapajós River water (p < 0.05). k′-values were not statistically different between the pure endmembers and the 50 and 33% Tapajós-Amazon mixtures for all rotational velocities (p > 0.10). When considering all incubations, there was no correlation between initial DO concentrations and k3x, k1x, or kStat (R2 = 0.07, 0.15, and 0.18, respectively). Calculated respiration rates followed the same trend as k′-values (Figure 1), and the difference between endmembers and mixtures was generally greater considering the mixtures had elevated DO concentrations compared to the endmembers, as previously described.
Discussion
Calculating pseudo first order reaction coefficients is a useful approach for comparing aquatic respiration potential between sites, along gradients, and under different experimental regimes without interference from factors such as aeration when sampling but has not been widely used in river systems. For example, k′-values of −0.29 to −0.72 (mean = 0.51) d−1 have been reported for the upper Pearl River estuary (Dai et al., 2006; Zhai unpublished data), which is similar to our observed range of endmember k3x-values of −0.40 to −0.65 (mean = −0.49) d−1, and lower than our mixture experiment k3x-values of −0.42 to −1.10 (mean = −0.67) d−1. In the case of both studies, experiments were performed in aerobic conditions, however, it is well known that the specific proton and electron donors involved with respiration in soils, sediments, and water bodies shift as DO levels drop below levels suitable for aerobic respiration (e.g., ∼0.3 mg L−1), altering pathways and rates of microbial respiration (del Giorgio and Williams, 2005).
Considering DO was not a limiting factor in our experiments, we hypothesize that the amplified respiration rates we observed when waters were mixed were related to a variety of factors linked to the unique geochemical constituents and microbial communities present in the two bodies of water that drive processes such as priming effects. TDN and DOC levels were not significantly different between sites, thus, nutrient or substrate limitation is not a likely driver for the amplified k′-values. The relatively small range of conductivity and pH between sites likely also did not exert strong differences in respiration when mixed. Our results show that regardless of what water was used, respiration rates are directly linked to the velocity at which samples are rotated, similar to findings by Ward et al. (2018). It was hypothesized that this linkage between velocity and aquatic metabolism occurs as a result of interactions between suspended particles, dissolved constituents, and free-living and particle-bound microbes considering that the presence of particles have been shown to play an important role in microbial activity and priming effects, or lack thereof Catalán et al. (2015).
Metatranscriptomic data from the lower Amazon River has shown that particle bound microbes are more metabolically active than free-living counterparts (Satinsky et al., 2015, 2017) even though the microbial assemblage is fairly similar between these two communities in the lower Amazon River (Doherty et al., 2017) in contrast to some other river (Crump et al., 1999), lake (Parveen et al., 2011), and marine settings (Kellogg and Deming, 2009). While microbial communities are very similar along the lower Amazon River mainstem, dominated by Actinobacteria and Betaproteobacteria, the Tapajós River contains a higher abundance of Cyanobacteria (∼12%) compared to the Amazon River (∼5%) (Doherty et al., 2017). Thus, mixing tributary and Amazon River waters likely yielded a more diverse microbial community. An experimental study showed that the presence of different organic substrates can cause shifts in marine microbial community composition and functional gene abundance; for example, when both algal- and sediment-derived OM were added together, 23 functional genes associated with aromatic carbon decomposition were found that were not present when these two substrates were added independently (Ward et al., 2019).
Considering that microbial community composition plays a central role in shaping the reactivity of OM in both terrestrial and aquatic environments (Schmidt et al., 2011), it is likely that amplified respiration rates in our mixed incubations were in part due to microbial diversification. The abundance of particular substrates have also been shown to have a strong positive correlation to the rate of their breakdown in the lower Amazon River, and isotopic tracer studies have shown that vascular plant-derived OM can be amplified by 1.5–6.0 times when algal OM is added to Amazon River water, which was attributed to priming effects (Ward et al., 2016). Therefore, we hypothesize that as tributaries mix, microbial activity is both amplified and diversified, resulting in enhanced respiration rates such as those observed here. The fact that k′-values slightly decreased relative to the endmembers in several cases suggests that there may be an optimal relative abundance for “priming” and “primed” substrates, or perhaps even negative priming effects under certain conditions (Gontikaki et al., 2013). For example, the Xingu River only had a significant increase in k′ with the 17% mixture. This could be related to the fact that water collected at Almeirim that was mixed with Xingu River water already represented a mixture of the Tapajós and Amazon rivers. Another potential mechanism for increased respiration potential upon mixing is desorption of nitrogen-rich molecules as Amazon River suspended sediments are diluted, providing both nutrients and reactive substrates that could also drive priming effects. For example, it has been shown that basic amino acids selectively sorb to fine sediments in the Amazon River (Aufdenkampe et al., 2001).
This study provides a brief examination of what happens as unique rivers mix, suggesting that such environments may be overlooked hotspots for carbon transformations. As human activities continue to alter the climate, landscape, and physical structure of inland water bodies, the footprint and impact of these “aquatic critical zones” are likely to shift in unknown ways (Bianchi and Morrison, 2018), necessitating a robust baseline understanding of present day conditions.
Author Contributions
RK conceived the idea for continuous O2 monitoring in a closed chamber, originally designed for use in the ocean. NW adapted the design to replicate rapidly mixed river waters and prepared the manuscript. NW and TB designed the experimental design of mixing waters. NW, HS, and JR performed the experiments in the field and oversaw project logistics. All authors discussed the results and commented on the manuscript.
Conflict of Interest Statement
The authors declare that the research was conducted in the absence of any commercial or financial relationships that could be construed as a potential conflict of interest.
Acknowledgments
Funding was provided by NSF DEB Grant #1256724, FAPESP Grant #08/58089-9, and the University of Florida Jon L. and Beverly A. Thompson Endowment. Field logistics were assisted by Alan Cunha, Daimio Brito, Rodrigo da Silva, Alex Krusche, and Troy Beldini. Research was performed aboard the Barco de Pesquisa “Mirage.” Other assistance in the field and lab was provided by Vania Neu, Diani Less, William Gagne-Maynard, Joel E. M. Diniz, Aline Valerio, Milton Kampel, and Victor Guedes.
References
Aufdenkampe, A. K., Hedges, J. I., Richey, J. E., Krusche, A. V., and Llerena, C. A. (2001). Sorptive fractionation of dissolved organic nitrogen and amino acids onto fine sediments within the Amazon Basin. Limnol. Oceanogr. 46, 1921–1935. doi: 10.4319/lo.2001.46.8.1921
Bengtsson, M. M., Attermeyer, K., and Catalán, N. (2018). Interactive effects on organic matter processing from soils to the ocean: are priming effects relevant in aquatic ecosystems? Hydrobiologia 822, 1–17. doi: 10.1007/s10750-018-3672-2
Bianchi, T. S. (2011). The role of terrestrially derived organic carbon in the coastal ocean: a changing paradigm and the priming effect. Proc. Natl. Acad. Sci. U.S.A. 108, 19473–19481. doi: 10.1073/pnas.1017982108
Bianchi, T. S., and Morrison, E. (2018). Human Activities Create Corridors of Change in Aquatic Zones. Washington, DC: EOS, 99.
Bianchi, T. S., Thornton, D. C. O., Yvon-lewis, S. A., King, G. M., Eglinton, T. I., Shields, M. R., et al. (2015). Positive priming of terrestrially derived dissolved organic matter in a freshwater microcosm system. Geophys. Res. Lett. 42, 5460–5467. doi: 10.1002/2015GL064765
Catalán, N., Kellerman, A. M., Peter, H., Carmona, F., and Tranvik, L. J. (2015). Absence of a priming effect on dissolved organic carbon degradation in lake water. Limnol. Oceanogr. 60, 159–168. doi: 10.1002/lno.10016
Catalán, N., Marcé, R., Kothawala, D. N., and Tranvik, L. J. (2016). Organic carbon decomposition rates controlled by water retention time across inland waters. Nat. Geosci. 9, 501–504. doi: 10.1038/ngeo2720
Cole, J. J., Prairie, Y. T., Caraco, N. F., McDowell, W. H., Tranvik, L. J., Striegl, R. G., et al. (2007). Plumbing the global carbon cycle: integrating inland waters into the terrestrial carbon budget. Ecosystems 10, 172–185. doi: 10.1007/s10021-006-9013-8
Crump, B. C., Armbrust, E. V., and Baross, J. A. (1999). Phylogenetic analysis of particle-attached and free-living bacterial communities in the Columbia River, its estuary, and the adjacent coastal ocean. Appl. Environ. Microbiol. 65, 3192–3204.
Dai, M., Guo, X., Zhai, W., Yuan, L., Wang, B., Wang, L., et al. (2006). Oxygen depletion in the upper reach of the Pearl River estuary during a winter drought. Mar. Chem. 102, 159–169. doi: 10.1016/j.marchem.2005.09.020
del Giorgio, P., and Williams, P. M. (eds) (2005). Respiration in Aquatic Ecosystems. Oxford, MA: Oxford University Press.
Doherty, M., Yager, P. L., Moran, M. A., Coles, V. J., Fortunato, C. S., Krusche, A. V., et al. (2017). Bacterial biogeography across the Amazon River-ocean continuum. Front. Microbiol. 8:882. doi: 10.3389/fmicb.2017.00882
Duan, S., He, Y., Kaushal, S. S., Bianchi, T. S., Ward, N. D., and Guo, L. (2017). Impact of wetland decline on decreasing dissolved organic carbon concentrations along the Mississippi River continuum. Front. Mar. Sci. 3:280. doi: 10.3389/FMARS.2016.00280
Feng, X., Feakins, S. J., Liu, Z., Ponton, C., Wang, R. Z., Karkabi, E., et al. (2016). Source to sink: evolution of lignin composition in the Madre de Dios River system with connection to the Amazon basin and offshore. J. Geophys. Res. Biogeosci. 121, 1316–1338. doi: 10.1002/2016jg003323
Gontikaki, E., Thornton, B., Huvenne, V. A. I., and Witte, U. (2013). Negative priming effect on organic matter mineralisation in NE Atlantic slope sediments. PLoS One 8:e0067722. doi: 10.1371/journal.pone.0067722
Hedges, J. I., Keil, R. G., and Benner, R. (1997). What happens to terrestrial organic matter in the ocean? Org. Geochem. 27, 195–212. doi: 10.1016/s0146-6380(97)00066-1
Holtgrieve, G. W., Schindler, D. E., Branch, T. A., and Teresa, Z. A. (2010). Simultaneous quantification of aquatic ecosystem metabolism and reaeration using a Bayesian statistical model of oxygen dynamics. Limnol. Oceanogr. 55, 1047–1063. doi: 10.4319/lo.2010.55.3.1047
Hotchkiss, E. R., and Hall, R. O. Jr. (2014). High rates of daytime respiration in three streams: use of δ18OO2 and O2 to model diel ecosystem metabolism. Limnol. Oceanogr. 59, 798–810. doi: 10.4319/lo.2014.59.3.0798
Kellogg, C. T., and Deming, J. W. (2009). Comparison of free-living, suspended particle, and aggregate-associated bacterial and archaeal communities in the Laptev Sea. Aquat. Microb. Ecol. 57, 1–18. doi: 10.3354/ame01317
Medeiros, P. M., Seidel, M., Ward, N. D., Carpenter, E. J., Gomes, H. R., Niggemann, J., et al. (2015). Fate of the Amazon river dissolved organic matter in the tropical Atlantic Ocean. Glob. Biogeochem. Cycles 29, 677–690. doi: 10.1002/2015GB005115
Parveen, B., Reveilliez, J. P., Mary, I., Ravet, V., Bronner, G., Mangot, J. F., et al. (2011). Diversity and dynamics of free-living and particle-associated Betaproteobacteria and Actinobacteria in relation to phytoplankton and zooplankton communities. FEMS Microbiol. Ecol. 77, 461–476. doi: 10.1111/j.1574-6941.2011.01130.x
Raymond, P. A., Hartmann, J., Lauerwald, R., Sobek, S., McDonald, C., Hoover, M., et al. (2013). Global carbon dioxide emissions from inland waters. Nature 503, 355–359. doi: 10.1038/nature12760
Richey, J. E., Brock, J. T., Naiman, R. J., Wissmar, R. C., and Stallard, R. F. (1980). Organic carbon: oxidation and transport in the Amazon River. Science 207, 1348–1351. doi: 10.1126/science.207.4437.1348
Riedel, T., Zark, M., Vähätalo, A. V., Niggemann, J., Spencer, R. G. M., Hernes, P. J., et al. (2016). Molecular signatures of biogeochemical transformations in dissolved organic matter from ten world rivers. Front. Earth Sci. 4:85. doi: 10.3389/feart.2016.00085
Satinsky, B. M., Fortunato, C. S., Doherty, M., Smith, C. B., Sharma, S., Ward, N. D., et al. (2015). Metagenomic and metatranscriptomic inventories of the lower Amazon River, May 2011. Microbiome 3:39. doi: 10.1186/s40168-015-0099-0
Satinsky, B. M., Smith, C. B., Sharma, S., Ward, N. D., Krusche, A. V., Richey, J. E., et al. (2017). Patterns of bacterial and archaeal gene expression through the lower Amazon River. Front. Mar. Sci. 4:253. doi: 10.3389/fmars.2017.00253
Sawakuchi, H. O., Neu, V., Ward, N. D., Barros, M. C., Valerio, A. M., Gagne-Maynard, W., et al. (2017). Carbon dioxide emissions along the lower Amazon River. Front. Mar. Sci. 4:76. doi: 10.3389/fmars.2017.00076
Schmidt, M. W. I., Torn, M. S., Abiven, S., Dittmar, T., Guggenberger, G., Janssens, I. A., et al. (2011). Persistence of soil organic matter as an ecosystem property. Nature 478, 49–56. doi: 10.1038/nature10386
Seidel, M., Dittmar, T., Ward, N. D., Krusche, A. V., Richey, J. E., Yager, P. L., et al. (2016). Seasonal and spatial variability of dissolved organic matter composition in the lower Amazon River. Biogeochemistry 131, 281–302. doi: 10.1007/s10533-016-0279-4
Seidel, M., Yager, P. L., Ward, N. D., Carpenter, E. J., Gomes, H. R., Krusche, A. V., et al. (2015). Molecular-level changes of dissolved organic matter along the Amazon River-to-ocean continuum. Mar. Chem. 177, 218–231. doi: 10.1016/j.marchem.2015.06.019
Vannote, R. L., Minshall, G. W., Cummins, K. W., Sedell, J. R., and Cushing, C. E. (1980). The river continuum concept. Can. J. Fish. Aquat. Sci. 37, 130–137.
Ward, N. D., Bianchi, T. S., Medeiros, P. M., Seidel, M., Richey, J. E., Keil, R. G., et al. (2017). Where carbon goes when water flows: carbon cycling across the aquatic continuum. Front. Mar. Sci. 4:7. doi: 10.3389/fmars.2017.00007
Ward, N. D., Bianchi, T. S., Sawakuchi, H. O., Gagne-maynard, W., Cunha, A. C., Brito, D. C., et al. (2016). The reactivity of plant-derived organic matter and the potential importance of priming effects along the lower Amazon River. J. Geophys. Res. Biogeosci. 121, 1522–1539. doi: 10.1002/2016JG003342
Ward, N. D., Keil, R. G., Medeiros, P. M., Brito, D. C., Cunha, A. C., Dittmar, T., et al. (2013). Degradation of terrestrially derived macromolecules in the Amazon River. Nat. Geosci. 6, 530–533. doi: 10.1038/ngeo1817
Ward, N. D., Krusche, A. V., Sawakuchi, H. O., Brito, D. C., Cunha, A. C., Moura, J. M. S., et al. (2015). The compositional evolution of dissolved and particulate organic matter along the lower Amazon River-Óbidos to the ocean. Mar. Chem. 177, 244–256. doi: 10.1016/j.marchem.2015.06.013
Ward, N. D., Morrison, E., Liu, Y., Rivas-Ubach, A., Osborne, T. Z., Ogram, A., et al. (2019). Marine microbial responses related to wetland carbon mobilization in the coastal zone. Limnol. Oceanogr. Lett. 4, 25–33. doi: 10.1002/lol2.10101
Keywords: respiration, aquatic, priming, carbon, mixing
Citation: Ward ND, Sawakuchi HO, Richey JE, Keil RG and Bianchi TS (2019) Enhanced Aquatic Respiration Associated With Mixing of Clearwater Tributary and Turbid Amazon River Waters. Front. Earth Sci. 7:101. doi: 10.3389/feart.2019.00101
Received: 16 January 2019; Accepted: 23 April 2019;
Published: 08 May 2019.
Edited by:
Toshi Nagata, The University of Tokyo, JapanReviewed by:
Wei-dong Zhai, Shandong University, ChinaJohn Christopher Lehrter, University of South Alabama, United States
Rainer M. W. Amon, Texas A&M University, United States
Copyright © 2019 Ward, Sawakuchi, Richey, Keil and Bianchi. This is an open-access article distributed under the terms of the Creative Commons Attribution License (CC BY). The use, distribution or reproduction in other forums is permitted, provided the original author(s) and the copyright owner(s) are credited and that the original publication in this journal is cited, in accordance with accepted academic practice. No use, distribution or reproduction is permitted which does not comply with these terms.
*Correspondence: Nicholas D. Ward, bmljaG9sYXMud2FyZEBwbm5sLmdvdg==