- 1School of Geography and water@leeds, University of Leeds, Leeds, United Kingdom
- 2Geography, Staffordshire University, Stoke‐on‐Trent, United Kingdom
- 3School of Humanities, York St John University, York, United Kingdom
Global climate change is evidently manifest in disappearing mountain glaciers and receding and thinning ice sheet margins. Concern about contemporaneous proglacial lake development has spurred an emerging area of research seeking to quantitatively understand lake - glacier interactions. This perspectives article identifies spatio-temporal disparity between the coverage of field data, remote sensing observations and numerical modeling efforts. Throughout, an overview of the physical effects of lakes on glaciers and on ice sheet margins is provided, drawing evidence together from very recent and high-impact studies of both modern glaciology and of the Quaternary record. We identify and discuss six challenges for numerical modeling of lake - glacier interactions, namely that there are meltwater exchanges between glaciers and ice-marginal lakes, lake bathymetry and glacier bed topography are often unknown, lake - glacier interactions affect the longitudinal stress regime of glaciers, lake water temperature affects glacier melting but is very poorly constrained, the interactions persist with considerable spatio-temporal variability and with boundary migration, and data for model parameterization and validation is extremely scarce. Overall, we contend that numerical modeling is a key frontier in the cryospheric sciences to deliver process understanding of lake - glacier interactions.
Introduction
Proglacial lakes are increasing in number and areal extent globally (Carrivick and Tweed, 2013; Shugar et al., 2020). Many recent regional studies have concentrated on the Himalaya (e.g., Komori, 2008; King et al., 2018, 2019; Brun et al., 2019; Falaschi et al., 2019; Maurer et al., 2019; Tsutaki et al., 2019; Zhang et al., 2019; Kumar et al., 2020; Luo et al., 2020) partly due to the importance of glaciers there for water resources and partly due to the threat posed to downstream communities and infrastructure by glacial outburst floods (GLOFS) or “jökulhlaups” (Carrivick and Rushmer, 2006; Carrivick and Tweed, 2016; Dubey and Goyal, 2020; Thompson et al., 2020; Veh et al., 2020). Examples of studies documenting increasing number and area of proglacial lakes from other regions of the world include southern Iceland (Schomacker, 2010; Guðmundsson et al., 2019), western Greenland (Carrivick and Quincey, 2014), central Tibet (Wang et al., 2013), Austria (Buckel et al., 2018) and south America (Wilson et al., 2018; Emmer et al., 2020). The prevalence of proglacial lakes will continue as glacier overdeepenings become ice-free and fill with meltwater (e.g., Magnin et al., 2020; Viani et al., 2020). Proglacial lakes can be ice-marginal (or else situated beyond an ice margin) and impounded by ice, debris or bedrock.
In recent Quaternary studies, ice-marginal lakes that formed immediately following the Last Glacial Maximum (LGM) have been investigated in great detail; across the Northern Hemisphere in Europe and Asia (e.g., Batchelor et al., 2019; Emery et al., 2019; Utting and Atkinson, 2019; Dalton et al., 2020; Herget et al., 2020; Winsemann and Lang, 2020; Turzewski et al., 2020), in Greenland (Carrivick et al., 2018; Adamson et al., 2019), in South America (e.g., Hall et al., 2019; Martin et al., 2019; Thorndycraft et al., 2019; Davies et al., 2020; Mendelová et al., 2020), and the Southern Alps of New Zealand (Sutherland et al., 2019a, b). Some of these lakes have been implicated in destabilizing ice sheets (Colman, 2002) and are thought to have been a control on ice stream onset and dynamics (Stokes and Clark, 2004; Perkins and Brennand, 2015). They have been associated with glacier surges (Clark et al., 1996; Ponce et al., 2019) and with meltwater pulses into the North Atlantic (Clark et al., 1996; Bauer et al., 2004). However, with a few exceptions (e.g., Clarke et al., 2009) these implied effects remain largely untested within numerical models.
Ice-marginal lake development must be understood if mountain glacier and/or ice sheet evolution; dynamics, mass loss, and meltwater contributions and sea level rise are to be accurately quantified. Unfortunately, field data are limited in spatial and temporal coverage. Unraveling cause and effect in remote sensing studies is difficult; is meltwater from diminishing glaciers making the lakes bigger, or are lakes accelerating glacier shrinkage, or is it (most likely) a combination of both and what is the balance between these sets of processes? Furthermore, the vast majority of glacier and ice sheet models to date, whether applied to modern or to Quaternary systems, either completely neglect ice-marginal lake effects, or else have an exceptionally trivial representation of them. This perspective article overviews the physical effects of ice-marginal lakes on glaciers and then discusses the key concerns for future development of numerical models.
Physical Effects of Lakes on Glaciers and Ice Sheet Margins
Ice-marginal lakes interact with glaciers via a series of thermo-mechanical processes (Tsutaki et al., 2011; Carrivick and Tweed, 2013; Tweed and Carrivick, 2015; Truffer and Motyka, 2016). These processes act in addition to climate-driven ablation effects, but can interact with those via several feedbacks. Temporary de-stabilization of an ice-margin, partially decoupling a glacier response from climate forcing (Kirkbride, 1993), can occur via calving, causing draw-down of ice as ice surface slopes are steepened (Figure 1). Furthermore, as a hydraulic connection is established between a subglacial drainage system and lake water it thereby reduces bed friction and reduces longitudinal stresses. Increased glacier flow speeds can be expected, with a reduction in back stress and decreased bed friction. The result is dynamic thinning (e.g., Trüssel et al., 2013; Tsutaki et al., 2019) introducing a positive feedback of decreasing effective pressure, enhanced basal ice motion and further enhanced flow velocity (Sugiyama et al., 2011).
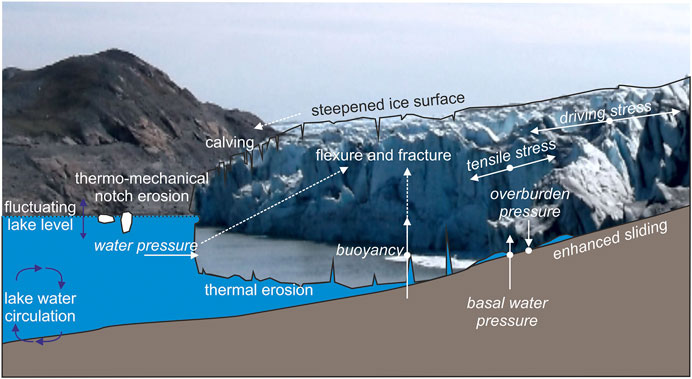
FIGURE 1. Schematic diagram of the most important components of ice-marginal lake - glacier interactions. These interactions can cause accelerated ice motion and mass loss, and glacier behaviour partially decoupled from climate forcing. Linkages between the components are indicated by dashed lines and the orientation of forces is indicated by solid lines. Adapted from Figure 7 of Carrivick and Tweed (2013).
Mass loss at lake-terminating margins of glaciers and ice sheets can be attributed to the effects of “frontal ablation,” which is a term used to include calving and subaqueous and subaerial frontal melt (Cogley et al., 2011). In contrast to marine glacier margins, there have been few attempts to quantify frontal ablation at lake-terminating ice-margins. Several studies have inferred subaqueous melt to be a negligible component of ablation (e.g., Boyce et al., 2007; Trüssel et al., 2013), whereas others have considered it to be a major component of mass loss and a rate-controlling process on calving, particularly where lake temperatures are relatively warm (e.g., Haresign and Warren, 2005; Sugiyama et al., 2016; Minowa et al., 2017) and/or the terminus is slow-flowing with limited crevassing (Kirkbride and Warren, 1997; Röhl, 2006). Subaqueous melt rates are a key control on the propagation of thermo-erosional notches at the waterline, and thus melt-undercut driven calving losses (Diolaiuti et al., 2006; Röhl, 2006). A recent analysis of glacier calving into an ice-marginal lake found associations between calving style and magnitude with presence/absence of lake ice cover and lake water depth (Mallalieu et al., 2020). Where lakes freeze or have a floating terminus, a buttressing effect (Geirsdottir et al., 2008; Tsutaki et al., 2013; Mallalieu et al., 2020) can be imparted on the glacier, rather like that of an ice mélange in a marine setting.
Effects of Sudden Lake Drainages
Ice-marginal lakes can suddenly drain due to failure of a moraine or ice dam. Typical triggers for such failure include mechanical breakage of unconsolidated moraine material or lake water pressure (due to water depth) exceeding ice overburden pressure (Tweed and Russell, 1999). Studies on modern glaciers have shown that when an ice-marginal lake drains, a local portion of the adjacent glacier is near-instantaneously de-buttressed promoting calving (e.g., Sugden, 1985; Mallalieu et al., 2020), localized crevassing, and an abrupt increase in horizontal velocity (e.g., Walder et al., 1996; Furuya and Wahr, 2005; Tsutaki et al., 2013). These effects have been detected with in situ measurements using global positioning systems (GPS) (e.g., Walder et al., 2006; Tsutaki et al., 2013), satellite interferometry (e.g., Furuya and Wahr, 2005), or via processing of multiple oblique field photographs (e.g., Mallalieu et al., 2017, 2020). Vertical displacements of an ice surface have been interpreted to signify passage of energetic and pressurized lake water through/beneath a glacier (e.g., Walder et al., 1996; Anderson et al., 2005; Tsutaki et al., 2013). Lake depth and longitudinal expansion of the lake are key to explaining glacier response to sudden lake drainage events, but are themselves a product of glacier dynamics, thereby complicating process understanding (Kienholz et al., 2020).
Many ice-dammed lakes drain multiple times establishing a filling-draining cycle (Clague and Evans, 2000; Carrivick and Tweed, 2019; Godbout et al., 2019), which further complicates attributions of cause and effect. During lake (re)filling, abrupt changes in glacier surface elevations have been associated with the formation of faults and fractures forced by englacial water, as evidenced by fluctuations in shallow borehole water pressures, and changes in radar internal reflection power (Bigelow et al., 2020).
Whether outburst floods from glacial lakes need to be included in ice sheet evolution models remains unconsidered, despite recognition in the geological record of their widespread influence on subglacial hydrology, especially in association with ice streams, for example, around the periphery of Antarctica (Kirkham et al., 2019; Larter et al., 2019) and in northern Greenland (Keisling et al., 2020).
Discussion of Concerns for Numerical Modeling
The spatio-temporal disparity between modern process-based field data and knowledge gained from the geological record mean that numerical modeling is necessary to deliver process-understanding, specifically on the relative importance of contributions of ice-marginal lakes to overall glacier ablation and dynamics. The space and time scales over which such non-linear feedback processes might be important need unraveling. A quantitative consideration of ice-marginal lake effects should be considered as a key frontier in glaciology. Six major issues are identified from the literature and are discussed in the next sections.
Meltwater Exchanges
As glaciers lose mass, retreat and thin, they release meltwater that can become ponded behind moraine ridges and within other topographic depressions. This is especially the case when a glacier rests on a retrograde slope (Nick et al., 2009), such as a glacial overdeepening, or on the periphery of an ice sheet covering an isostatically-depressed region, such as west Greenland (Morlighem et al., 2017). Until recently, representation of feedback between glacier meltwater generation and ice-marginal lake evolution remained beyond the capability and capacity of mountain glacier or ice sheet models.
Depressions in topography (see Figure 2) act as a sink for many algorithms of water flow routing (Zhu et al., 2006). So-called “flood-fill” algorithms can be used to determine the extent and depth of lakes from topography data. However, computational time can become a limiting factor when applying flood-fill algorithms to large geographical extents and long timescales (for example, in the case of palaeo-ice sheets). Berends and van de Wal’s (2016) model can be completely coupled to glacio-isostatic adjustment (GIA) models and thus the mass of lake water is added to the mass of ice for GIA/sea level calculations, accounting for bedrock deformation and geoid perturbation. These deformation effects both influence the volume of the lake by deepening the basin and through raising the water level. In contrast, Hinck et al. (2020) identify lake basins and the corresponding maximum water level for a given ice sheet and topography reconstruction without including advanced flow routing techniques. They applied their model to reconstructions of North America between the LGM and the present day, successfully reconstructing Lake Agassiz, Lake McConnell and the predecessors of the Great Lakes. Both Berends and van de Wal’s (2016) and Hinck et al. (2020) algorithms negate the need to prescribe lake boundaries. More sophisticated schemes such as “connected-component schemes” (e.g., Bueler and van Pelt, 2015) are likely to be more efficient for larger-scale computations.
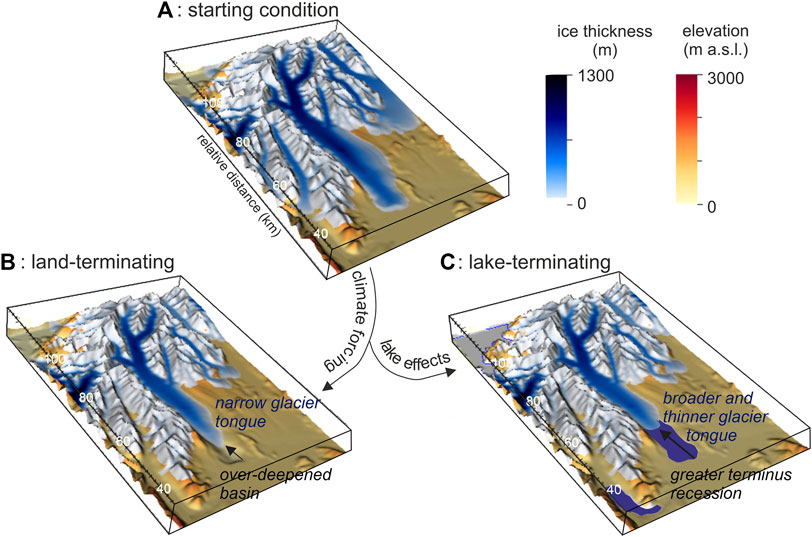
FIGURE 2. Visualization of outputs from BISICLES ice sheet model (Cornford et al., 2013) used by Sutherland et al. (2020) with starting condition of a glacier in equilibrium (A), then forced by climate only (B) and then by the same climate forcing with the addition of ice-marginal lake effects (C). The modeled lake-terminating glacier has a different terminus geometry and retreats further in the same period of time as the land-terminating glacier.
Ice-marginal lakes are commonly hydraulically connected to a glacier and to each other through subglacial drainage systems. Stubblefield et al. (2019) developed and experimentally analyzed a numerical model of the evolution of glacial lakes connected by subglacial channels to analyze filling-drainage cycles of those lakes as the meltwater supply varies. They highlighted that the dynamic and two-way coupling between lake dynamics and glacier behavior affected bed friction, glacier thermal regime and hence ice motion in addition to mechanical buttressing.
Lake Bathymetry and Glacier Bed Topography
The modeling outlined in Meltwater Exchanges strongly depends on topographic data resolution and on lake bathymetry. Indeed, lake bathymetry and glacier bed topography are essential datasets for all numerical models wishing to simulate glacier behavior and lake—glacier interactions. Unfortunately, acquiring lake bathymetry data and bed topography data is very difficult and field data often simply does not exist. The Ice Thickness Models Intercomparison Experiment (ItMix) (Farinotti et al., 2019) has provided a consensus model estimate of ice thickness for all mountain glaciers globally, from which bed topography can be derived. The margins of ice sheets and mountain glaciers are especially poorly constrained because airborne survey instruments struggle to generate reliable data where an ice surface is significantly broken up, the ice substrate is near or at the melting point, and scattering and signal absorption are high due to wet and often debris-rich ice (e.g., Morlighem et al., 2013).
Longitudinal Stress Regime
Ice-marginal lakes fundamentally alter the longitudinal stress and flow regime of a glacier and that effect can propagate kilometres up-ice from the terminus. Field data analysis and remote-sensing of glacier surface velocity by Tsutaki et al. (2019) and by Liu et al. (2020) have both showed that the presence of an ice-marginal lake alters glacier thickness. They both attributed the effects of the lake to be a dynamic thinning mechanism. Tsutaki et al. (2019) additionally conducted some numerical simulations to support their dynamic thinning interpretation but their model had a static lake water level. However, the positive feedback between glacier mass loss, as manifest in ice-margin recession and surface lowering, and meltwater feeding an enlarging lake means that lake levels fluctuate; often by many tens of meters (e.g., Carrivick et al., 2017; Armstrong and Anderson, 2020). Dynamic water levels are technically difficult to achieve and computationally expensive to consider. Similar effects of ice-marginal lakes affecting longitudinal flow extending many kilometres up-ice have been interpreted recently for large lobate outlet glaciers in southern Iceland (e.g., Storrar et al., 2017; Dell et al., 2019; Baurley et al., 2020) and numerically modeled for an ice sheet margin in west Greenland (Price et al., 2008).
Lake Water Temperature
Ice-marginal lake water temperature dramatically affects subaqueous melt rate, but unfortunately is very rarely quantified. There is an unspoken assumption that it remains close to zero due to a predominant influence of subglacial meltwater inputs, but in actuality it can vary considerably between seasons, both in magnitude (to over 10°C) and also in stratification pattern (Haresign and Warren, 2005; Röhl, 2006; Sugiyama et al., 2016; Carrivick et al., 2017; Minowa et al., 2017; Mallalieu et al., 2020; Watson et al., 2020). On a small (meters) scale, thermo-mechanical notch development will be affected by lake water temperature (e.g., Diolaiuti et al., 2006; Röhl, 2006). On a meso-scale, lake temperature affects subglacial hydrology (Carrivick et al., 2017; Stubblefield et al., 2019). On a macro scale (hundreds to thousands of meters) lake water temperature will affect the shape of the floating underside of a glacier and potentially also affect the shape of the entire terminus tongue (Sugiyama et al., 2019; Sutherland et al., 2020; Figure 2). Subglacial hydrology is affected by lake water temperature at all scales. Lakes can affect local surface energy balance via an albedo effect, as well as a thermal heat capacity effect.
Lake water temperature and lake albedo can affect local air movements, and lake water circulation currents can be driven by valley winds. This modification of a local surface energy balance by lakes, and the potential for wind to enhance thermal undercutting and calving, is probably proportional to lake size (Sakai et al., 2009; Sugiyama et al., 2016), but remains unquantified. Very large lakes can act as moisture sources, enhancing precipitation on parts of ice sheets.
Spatio-Temporal Variability and Boundary Migration
Representing the impact of short-lived (decadal) accelerations and decelerations of a glacier in response to ice-marginal lake effects (Sutherland et al., 2020) is difficult within a longer (millennial) glacier evolution model, and not least because those effects might not simply average out. Being able to perform long simulations over large spatial extents must contend with lake size being highly sensitive to the size and position of lake overspill channels, which are on a scale of 10–100 m.
There is a spatio-temporal disconnect between observations made in the field (days to weeks), those from remote-sensing (inter-annual) and numerical modeling concerns (decades to centuries to millennia). This disconnect is a problem because boundary conditions and parameters needed by models vary spatio-temporally. Lake bathymetry, glacier bed topography, lake level and lake water temperature vary in space as well as through time. Multiple ice-marginal lakes will likely have differing elevations, which is quite different to conditions at a marine boundary and makes it difficult to adapt a marine ice sheet model for use with ice-marginal lakes (Sutherland et al., 2020). Furthermore, individual lakes will have levels that vary with filling and draining. Convective circulation due to thermal warming of distal water could enhance subaqueous melting. Thermal stratification will likely be an effective contribution to glacier melt seasonally (Sugiyama et al., 2016). The differences between water temperatures at an ice-marginal lake boundary and at a marine boundary are yet to be fully elucidated. A lake—glacier boundary is not a simple interface; it moves in time with lake expansion and glacier retreat, for example. It is complex in that lake water can be hydraulically-connected to a subglacial drainage network.
Calving is assumed to be the dominant effect of a water boundary at an ice-margin. That assumption might be valid for marine-terminating glaciers but remains untested for lake-terminating glaciers. Calving models are increasing in number and complexity and they are becoming more and more explicit in their process representation but, because they are orientated toward marine boundaries, they tend to be driven by short-term (intra-seasonal) observations. However, some of the water-depth calving laws are derived from data at lake margins (e.g., Benn et al., 2007). The few inter-annual, decadal to millennial scale glacier evolution models are either of a statistical nature; with a stochastic representation of calving, or else they are of a highly-parameterized numerical modeling type. The two most important factors controlling rates and mechanisms of calving at ice-marginal lake boundaries are water depth relative to ice thickness for buoyancy-driven calving (e.g., Boyce et al., 2007; Trüssel et al., 2013) and lake water temperature for melt-undercutting (e.g., Haresign and Warren 2005; Diolaiuti et al., 2006; Röhl, 2006). Buoyancy is different in freshwater compared to marine settings due to water density differences and possibly also ice density differences. A third calving mechanism, which is commonly considered in marine ice sheet models, is hydrofracturing whereby water pressure within surface crevasses causing propagation of that crevasse to the glacier bed, but this calving model has yet to be understood for lake-terminating glaciers.
The few studies that have sought to understand glacier or ice sheet evolution with representation of ice-marginal lake effects have adapted marine ice sheet models (e.g., Figure 2). Such an adaptation is not a trivial task, either conceptually for the reasons listed above, but also from mathematical, numerical and computational perspectives. Some of the earlier examples of this sort of work were on the evolution of the Laurentide Ice Sheet and included lakes as a calving term for land-based margins (Tarasov and Peltier, 2004, 2006).
Data Available to Validate Models
The previous five sub-sections all concern boundary conditions or data that might be used to parameterize numerical models. Model validation must come from independent quantification of the evolution of lake - glacier boundaries in space and time. For example, a study wishing to numerically model the evolution of the Laurentide Ice Sheet would need geochronological data with good spatial coverage to adequately constrain not only glacier ice margin positions (e.g., Dalton et al., 2020) but also ice-marginal lake shoreline transgressions. Inventories of ice-marginal lake occurrence and areal size are improving in quality and coverage all the time (see Introduction to this article) but looking forwards, there are exciting prospects offered by using cloud computing, such as Google Earth Engine, for efficient automatic identification and geometric delineation of ice-marginal lakes from multiple satellite images and over wide geographical areas.
Summary, Conclusions and Future Prospects
This article has emphasized that there is a pressing need to include lake—glacier interactions in numerical models of mountain glacier and ice sheet evolution. It has highlighted that there are a several challenges to overcome for those modeling efforts to be meaningful. The problem of including lake - glacier interactions can be summarized as follows i) determining which processes have significant effects and ii) determining physically-plausible parameter ranges. When moving upscale from single lake-glaciers to whole ice sheets, then also iii) identifying which processes to ignore or simplify, and iv) developing a model framework that can account for and track individual lake size and elevation. This Perspectives paper provides the foundation for future studies aiming to address these problems more thoroughly. Concerted efforts continue by researchers around the World who are applying ice sheet models to ice-marginal lake problems, most especially to reconstructions of the Laurentide Ice Sheet for interest in ice sheet (in)stability and collapse, ice stream development and meltwater pulses. Understanding the last Quaternary (de)glaciation will inform our understanding of the present and future evolution of the Greenland and Antarctic ice sheets. Indeed, we challenge future Greenland and Antarctic ice sheet models to consider lake—glacier interactions.
Data Availability Statement
The original contributions presented in the study are included in the article, further inquiries can be directed to the corresponding author.
Author Contributions
All authors listed have made a substantial, direct, and intellectual contribution to the work and approved it for publication.
Funding
JLS was supported by a NERC PhD studentship NE/L002574/1. JM was supported by a Graduate Teaching Assistantship from the School of Geography, University of Leeds.
Conflict of Interest
The authors declare that the research was conducted in the absence of any commercial or financial relationships that could be construed as a potential conflict of interest.
Acknowledgments
We thank the editor and reviewers for their very helpful suggestions that have improved this manuscript.
References
Adamson, K., Lane, T., Carney, M., Bishop, T., and Delaney, C. (2019). High‐resolution proglacial lake records of pre‐Little Ice Age glacier advance, northeast Greenland. Boreas 48 (3), 535–550. doi:10.1111/bor.12361
Anderson, R. S., Walder, J. S., Anderson, S. P., Trabant, D. C., and Fountain, A. G. (2005). The dynamic response of Kennicott Glacier, Alaska, USA, to the Hidden Creek lake outburst flood. Ann. Glaciol. 40, 237–242. doi:10.3189/172756405781813438
Armstrong, W. H., and Anderson, R. S. (2020). Ice-marginal lake hydrology and the seasonal dynamical evolution of Kennicott Glacier, Alaska. J. Glaciol. 66, 1–15. doi:10.1017/jog.2020.41
Batchelor, C. L., Margold, M., Krapp, M., Murton, D. K., Dalton, A. S., Gibbard, P. L., et al. (2019). The configuration of Northern Hemisphere ice sheets through the Quaternary. Nat. Commun. 10 (1), 1–10. doi:10.1038/s41467-019-11601-2
Bauer, E., Ganopolski, A., and Montoya, M. (2004). Simulation of the cold climate event 8200 years ago by meltwater outburst from Lake Agassiz. Paleoceanography 19 (3), PA3014. doi:10.1029/2004pa001030
Baurley, N. R., Robson, B., and Hart, J. K. (2020). Long‐term impact of the proglacial lake Jökulsárlón on the flow velocity and stability of Breiðamerkurjökull glacier, Iceland. Earth Surf. Process Landf. 45, 2647–2663. doi:10.1002/esp.4920
Benn, D. I., Warren, C. R., and Mottram, R. H. (2007). Calving processes and the dynamics of calving glaciers. Earth Sci. Rev. 82 (3–4), 143–179. doi:10.1016/j.earscirev.2007.02.002
Berends, C. J., and Van De Wal, R. S. W. (2016). A computationally efficient depression-filling algorithm for digital elevation models, applied to proglacial lake drainage. Geosci. Model Dev. 9 (12), 4451–4460. doi:10.5194/gmd-9-4451-2016
Bigelow, D. G., Flowers, G. E., Schoof, C. G., Mingo, L. D., Young, E. M., and Connal, B. G. (2020). The role of englacial hydrology in the filling and drainage of an ice‐dammed lake, Kaskawulsh Glacier, Yukon, Canada. J. Geophys. Res. Earth Surf. 125 (2), e2019JF005110. doi:10.1029/2019jf005110
Boyce, E. S., Motyka, R. J., and Truffer, M. (2007). Flotation and retreat of a lake-calving terminus, Mendenhall Glacier, southeast Alaska, USA. J. Glaciol. 53 (181), 211–224. doi:10.3189/172756507782202928
Brun, F., Wagnon, P., Berthier, E., Jomelli, V., Maharjan, S. B., Shrestha, F., et al. (2019). Heterogeneous influence of glacier morphology on the mass balance variability in High Mountain Asia. J. Geophys. Res. Earth Surf. 124 (6), 1331–1345. doi:10.1029/2018jf004838
Buckel, J., Otto, J. C., Prasicek, G., and Keuschnig, M. (2018). Glacial lakes in Austria - distribution and formation since the Little Ice Age. Glob. Planet. Chang. 164, 39–51. doi:10.1016/j.gloplacha.2018.03.003
Bueler, E., and van Pelt, W. (2015). Mass-conserving subglacial hydrology in the Parallel Ice Sheet model version 0.6. Geosci. Model Dev. 8, 1613–1635. doi:10.5194/gmd-8-1613-2015
Carrivick, J. L., and Quincey, D. J. (2014). Progressive increase in number and volume of ice-marginal lakes on the western margin of the Greenland Ice Sheet. Glob. Planet. Chang. 116, 156–163. doi:10.1016/j.gloplacha.2014.02.009
Carrivick, J. L., and Rushmer, E. L. (2006). Understanding high-magnitude outburst floods. Geol. Today. 22 (2), 60–65. doi:10.1111/j.1365-2451.2006.00554.x
Carrivick, J. L., and Tweed, F. S. (2016). A global assessment of the societal impacts of glacier outburst floods. Glob. Planet. Chang. 144, 1–16. doi:10.1016/j.gloplacha.2016.07.001
Carrivick, J. L., and Tweed, F. S. (2019). A review of glacier outburst floods in Iceland and Greenland with a megafloods perspective. Earth Sci. Rev. 196, 102876. doi:10.1016/j.earscirev.2019.102876
Carrivick, J. L., Tweed, F. S., Ng, F., Quincey, D. J., Mallalieu, J., Ingeman-Nielsen, T., et al. (2017). Ice-dammed lake drainage evolution at Russell glacier, west Greenland. Front. Earth Sci. 5, 100. doi:10.3389/feart.2017.00100
Carrivick, J. L., and Tweed, F. S. (2013). Proglacial lakes: character, behaviour and geological importance. Quat. Sci. Rev. 78, 34–52. doi:10.1016/j.quascirev.2013.07.028
Carrivick, J. L., Yde, J. C., Knudsen, N. T., and Kronborg, C. (2018). Ice-dammed lake and ice-margin evolution during the Holocene in the Kangerlussuaq area of west Greenland. Arctic Antarct. Alpine Res. 50 (1), S100005. doi:10.1080/15230430.2017.1420854
Clague, J. J., and Evans, S. G. (2000). A review of catastrophic drainage of moraine-dammed lakes in British Columbia. Quat. Sci. Rev. 19 (17–18), 1763–1783. doi:10.1016/s0277-3791(00)00090-1
Clark, P. U., Alley, R. B., Keigwin, L. D., Licciardi, J. M., Johnsen, S. J., and Wang, H. (1996). Origin of the first global meltwater pulse following the last glacial maximum. Paleoceanography 11 (5), 563–577. doi:10.1029/96pa01419
Clarke, G. K. C., Bush, A. B. G., and Bush, J. W. M. (2009). Freshwater discharge, sediment transport, and modeled climate impacts of the final drainage o glacial Lake Agassiz. J. Clim. 22 (8), 2161–2180. doi:10.1175/2008jcli2439.1
Cogley, J. G., Hock, R., Rasmussen, L. A., Arendt, A. A., Bauder, A., Braithwaite, R. J., et al. (2011). Glossary of glacier mass balance and related terms. IHP-VII technical Documents in hydrology No. 86, IACS contribution No. 2, UNESCO-IHP, Paris.
Colman, S. M. (2002). Paleoclimate: a fresh look at glacial floods. Science 296 (5571), 1251–1252. doi:10.1126/science.1073377
Cornford, S. L., Martin, D. F., Graves, D. T., Ranken, D. F., Le Brocq, A. M., Gladstone, R. M., et al. (2013). Adaptive mesh, finite volume modeling of marine ice sheets. J. Comput. Phys. 232 (1), 529–549. doi:10.1016/j.jcp.2012.08.037
Dalton, A. S., Margold, M., Stokes, C. R., Tarasov, L., Dyke, A. S., Adams, R. S., et al. (2020). An updated radiocarbon-based ice margin chronology for the last deglaciation of the North American Ice Sheet Complex. Quat. Sci. Rev. 234, 106223. doi:10.1016/j.quascirev.2020.106223
Davies, B. J., Darvill, C. M., Lovell, H., Bendle, J. M., Dowdeswell, J. A., Fabel, D., et al. (2020). The evolution of the Patagonian Ice Sheet from 35 ka to the present day (PATICE). Earth Sci. Rev. 204, 103152. doi:10.1016/j.earscirev.2020.103152
Dell, R., Carr, R., Phillips, E., and Russell, A. J. (2019). Response of glacier flow and structure to proglacial lake development and climate at Fjallsjökull, south-east Iceland. J. Glaciol. 65 (250), 321–336. doi:10.1017/jog.2019.18
Diolaiuti, G., Citterio, M., Carnielli, T., D'agata, C., Kirkbride, M., and Smiraglia, C. (2006). Rates, processes and morphology of freshwater calving at Miage Glacier (Italian Alps). Hydrol. Process. 20 (10), 2233–2244. doi:10.1002/hyp.6198
Dubey, S., and Goyal, M. K. (2020). Glacial lake outburst flood hazard, downstream impact, and risk over the Indian Himalayas. Water Resour. Res. 56 (4), e2019WR026533. doi:10.1029/2019wr026533
Emery, A. R., Hodgson, D. M., Barlow, N., Carrivick, J. L., Cotterill, C., and Phillips, E. (2019). Left high and dry: deglaciation of Dogger Bank, North Sea, recorded in proglacial lake evolution. Front. Earth Sci. 7, 234. doi:10.3389/feart.2019.00234
Emmer, A., Harrison, S., Mergili, M., Allen, S., Frey, H., and Huggel, C. (2020). 70 years of lake evolution and glacial lake outburst floods in the Cordillera Blanca (Peru) and implications for the future. Geomorphology 365, 107178. doi:10.1016/j.geomorph.2020.107178
Falaschi, D., Lenzano, M. G., Villalba, R., Bolch, T., Rivera, A., and Lo Vecchio, A. (2019). Six decades (1958–2018) of geodetic glacier mass balance in Monte san Lorenzo, Patagonian Andes. Front. Earth Sci. 7 (236), 1–20. doi:10.3389/feart.2019.00326
Farinotti, D., Huss, M., Fürst, J. J., Landmann, J., Machguth, H., Maussion, F., et al. (2019). A consensus estimate for the ice thickness distribution of all glaciers on Earth. Nat. Geosci. 12 (3), 168–173. doi:10.1038/s41561-019-0300-3
Furuya, M., and Wahr, J. M. (2005). Water level changes at an ice-dammed lake in west Greenland inferred from InSAR data. Geophys. Res. Lett. 32 (14), L14501. doi:10.1029/2005gl023458
Geirsdottir, A., Miller, G. H., Wattrus, N. J., Bjornsson, H., and Thors, K. (2008). Stabilization of glaciers terminating in closed water bodies: evidence and broader implications. Geophys. Res. Lett. 35, PA3014. doi:10.1029/2008gl034432
Godbout, P.-M., Roy, M., and Veillette, J. J. (2019). High-resolution varve sequences record one major late-glacial ice readvance and two drainage events in the eastern Lake Agassiz-Ojibway basin. Quat. Sci. Rev. 223, 105942. doi:10.1016/j.quascirev.2019.105942
Guðmundsson, S., Björnsson, H., Pálsson, F., Magnússon, E., Sæmundsson, Þ., and Jóhannesson, T. (2019). Terminus lakes on the south side of Vatnajökull ice cap, SE-Iceland. Jokull 69, 1–34. doi:10.33799/jokull2019.69.001
Hall, B. L., Lowell, T. V., Bromley, G. R. M., Denton, G. H., and Putnam, A. E. (2019). Holocene glacier fluctuations on the northern flank of Cordillera Darwin, southernmost South America. Quat. Sci. Rev. 222, 105904. doi:10.1016/j.quascirev.2019.105904
Haresign, E., and Warren, C. R. (2005). Melt rates at calving termini: a study at Glaciar León, Chilean Patagonia. Geol. Soc. Spec. 242 (1), 99–109. doi:10.1144/gsl.sp.2005.242.01.09
Herget, J., Agatova, A. R., Carling, P. A., and Nepop, R. K. (2020). Altai megafloods-the temporal context. Earth Sci. Rev. 200, 102995. doi:10.1016/j.earscirev.2019.102995
Hinck, S., Gowan, E. J., and Lohmann, G. (2020). LakeCC: a tool for efficiently identifying lake basins with application to palaeogeographic reconstructions of North America. J. Quat. Sci. 35 (3), 422–432. doi:10.1002/jqs.3182
Keisling, B. A., Nielsen, L. T., Hvidberg, C. S., Nuterman, R., and DeConto, R. M. (2020). Pliocene-Pleistocene megafloods as a mechanism for Greenlandic megacanyon formation. Geology 48 (7), 737–741. doi:10.1130/g47253.1
Kienholz, C., Pierce, J., Hood, E., Amundson, J., Wolken, G., Jacobs, A., et al. (2020). Deglacierization of a marginal basin and implications for outburst floods, Mendenhall Glacier, Alaska. Front. Earth Sci. 8, 137. doi:10.3389/feart.2020.00137
King, O., Bhattacharya, A., Bhambri, R., and Bolch, T. (2019). Glacial lakes exacerbate Himalayan glacier mass loss. Sci. Rep. 9 (1), 1–9. doi:10.1038/s41598-019-53733-x
King, O., Dehecq, A., Quincey, D., and Carrivick, J. (2018). Contrasting geometric and dynamic evolution of lake and land-terminating glaciers in the central Himalaya. Glob. Planet. Chang. 167, 46–60. doi:10.1016/j.gloplacha.2018.05.006
Kirkbride, M. P. (1993). The temporal significance of transitions from melting to calving termini at glaciers in the central Southern Alps of New Zealand. Holocene 3 (3), 232–240. doi:10.1177/095968369300300305
Kirkbride, M. P., and Warren, C. R. (1997). Calving processes at a grounded ice cliff. Ann. Glaciol. 24, 116–121. doi:10.3189/s0260305500012039
Kirkham, J. D., Hogan, K. A., Larter, R. D., Arnold, N. S., Nitsche, F. O., Golledge, N. R., et al. (2019). Past water flow beneath pine Island and Thwaites glaciers, west Antarctica. Cryosphere 13, 1959–1981. doi:10.5194/tc-13-1959-2019
Komori, J. (2008). Recent expansions of glacial lakes in the Bhutan Himalayas. Quat. Int. 184 (1), 177–186. doi:10.1016/j.quaint.2007.09.012
Kumar, R., Bahuguna, I. M., Ali, S. N., and Singh, R. (2020). Lake inventory and evolution of glacial lakes in the Nubra-Shyok basin of Karakoram Range. Earth Syst. Environ. 4 (1), 57–70. doi:10.1007/s41748-019-00129-6
Larter, R. D., Hogan, K. A., Hillenbrand, C.-D., Smith, J. A., Batchelor, C. L., Cartigny, M., et al. (2019). Subglacial hydrological control on flow of an Antarctic Peninsula palaeo-ice stream. Cryosphere 13 (6), 1583–1596. doi:10.5194/tc-13-1583-2019
Liu, Q., Mayer, C., Wang, X., Nie, Y., Wu, K., Wei, J., et al. (2020). Interannual flow dynamics driven by frontal retreat of a lake-terminating glacier in the Chinese Central Himalaya. Earth Planet. Sci. Lett. 546, 116450. doi:10.1016/j.epsl.2020.116450
Luo, W., Zhang, G., Chen, W., and Xu, F. (2020). Response of glacial lakes to glacier and climate changes in the western Nyainqentanglha range. Sci. Total Environ., 735, 139607. doi:10.1016/j.scitotenv.2020.139607
Magnin, F., Haeberli, W., Linsbauer, A., Deline, P., and Ravanel, L. (2020). Estimating glacier-bed overdeepenings as possible sites of future lakes in the de-glaciating Mont Blanc massif (Western European Alps). Geomorphology 350, 106913. doi:10.1016/j.geomorph.2019.106913
Mallalieu, J., Carrivick, J. L., Quincey, D. J., and Smith, M. W. (2020). Calving seasonality associated with melt‐undercutting and lake ice cover. Geophys. Res. Lett. 47, e2019GL086561. doi:10.1029/2019gl086561
Mallalieu, J., Carrivick, J. L., Quincey, D. J., Smith, M. W., and James, W. H. M. (2017). An integrated structure-from-Motion and time-lapse technique for quantifying ice-margin dynamics. J. Glaciol. 63 (242), 937–949. doi:10.1017/jog.2017.48
Martin, J. R. V., Davies, B. J., and Thorndycraft, V. R. (2019). Glacier dynamics during a phase of Late Quaternary warming in Patagonia reconstructed from sediment-landform associations. Geomorphology 337, 111–133. doi:10.1016/j.geomorph.2019.03.007
Maurer, J. M., Schaefer, J. M., Rupper, S., and Corley, A. (2019). Acceleration of ice loss across the Himalayas over the past 40 years. Sci. Adv. 5 (6), eaav7266. doi:10.1126/sciadv.aav7266
Mendelová, M., Hein, A. S., Rodés, Á., Smedley, R. K., and Xu, S. (2020). Glacier expansion in central Patagonia during the Antarctic cold Reversal followed by retreat and stabilisation during the Younger Dryas. Quat. Sci. Rev. 227, 106047. doi:10.1016/j.quascirev.2019.106047
Minowa, M., Sugiyama, S., Sakakibara, D., and Skvarca, P. (2017). Seasonal variations in ice-front position controlled by frontal ablation at glaciar Perito Moreno, the southern Patagonia Icefield. Front. Earth Sci. 5, 1. doi:10.3389/feart.2017.00001
Morlighem, M., Rignot, E., Mouginot, J., Wu, X., Seroussi, H., Larour, E., et al. (2013). High-resolution bed topography mapping of Russell Glacier, Greenland, inferred from Operation IceBridge data. J. Glaciol. 59 (218), 1015–1023. doi:10.3189/2013jog12j235
Morlighem, M., Williams, C. N., Rignot, E., An, L., Arndt, J. E., Bamber, J. L., et al. (2017). BedMachine v3: complete bed topography and ocean bathymetry mapping of Greenland from multibeam echo sounding combined with mass conservation. Geophys. Res. Lett. 44 (21), 11–051. doi:10.1002/2017gl074954
Nick, F. M., Vieli, A., Howat, I. M., and Joughin, I. (2009). Large-scale changes in Greenland outlet glacier dynamics triggered at the terminus. Nat. Geosci. 2 (2), 110–114. doi:10.1038/ngeo394
Perkins, A. J., and Brennand, T. A. (2015). Refining the pattern and style of Cordilleran Ice Sheet retreat: palaeogeography, evolution and implications of lateglacial ice-dammed lake systems on the southern Fraser Plateau, British Columbia, Canada. Boreas 44, 319–342. doi:10.1111/bor.12100
Ponce, J. F., González Guillot, M., Díaz Balocchi, L., and Martínez, O. (2019). Geomorphological evidences of paleosurge activity in lake Viedma Lobe, Patagonia, Argentina. Geomorphology 327, 511–522. doi:10.1016/j.geomorph.2018.11.023
Price, S. F., Payne, A. J., Catania, G. A., and Neumann, T. A. (2008). Seasonal acceleration of inland ice via longitudinal coupling to marginal ice. J. Glaciol. 54 (185), 213–219. doi:10.3189/002214308784886117
Röhl, K. (2006). Thermo-erosional notch development at fresh-water-calving Tasman Glacier, New Zealand. J. Glaciol. 52 (177), 203–213. doi:10.3189/172756506781828773
Sakai, A., Nishimura, K., Kadota, T., and Takeuchi, N. (2009). Onset of calving at supraglacial lakes on debris-covered glaciers of the Nepal Himalaya. J. Glaciol. 55 (193), 909–917. doi:10.3189/002214309790152555
Schomacker, A. (2010). Expansion of ice-marginal lakes at the Vatnajökull ice cap, Iceland, from 1999 to 2009. Geomorphology 119 (3–4), 232–236. doi:10.1016/j.geomorph.2010.03.022
Shugar, D. H., Burr, A., Haritashya, U. K., Kargel, J. S., Watson, C. S., Kennedy, M. C., et al. (2020). Rapid worldwide growth of glacial lakes since 1990. Nat. Clim. Chang. 10, 939–945. doi:10.1038/s41558-020-0855-4
Stokes, C. R., and Clark, C. D. (2004). Evolution of late glacial ice-marginal lakes on the northwestern Canadian Shield and their influence on the location of the Dubawnt Lake palaeo-ice stream. Palaeogeogr. Palaeoclimatol. Palaeoecol. 215 (1–2), 155–171. doi:10.1016/s0031-0182(04)00467-5
Storrar, R. D., Jones, A. H., and Evans, D. J. A. (2017). Small-scale topographically-controlled glacier flow switching in an expanding proglacial lake at Breiðamerkurjökull, SE Iceland. J. Glaciol. 63 (240), 745–750. doi:10.1017/jog.2017.22
Stubblefield, A. G., Creyts, T. T., Kingslake, J., and Spiegelman, M. (2019). Modeling oscillations in connected glacial lakes. J. Glaciol. 65, 745–758. doi:10.1017/jog.2019.46
Sugden, D. E., Clapperton, C. M., and Knight, P. G. (1985). A jökulhlaup near Søndre Strømfjord, west Greenland, and some effects on the ice-sheet margin. J. Glaciol. 31 (109), 366–368. doi:10.1017/s0022143000006729
Sugiyama, S., Minowa, M., Sakakibara, D., Skvarca, P., Sawagaki, T., Ohashi, Y., et al. (2016). Thermal structure of proglacial lakes in Patagonia. J. Geophys. Res. Earth Surf. 121 (12), 2270–2286. doi:10.1002/2016jf004084
Sugiyama, S., Minowa, M., and Schaefer, M. (2019). Underwater ice terrace observed at the front of Glaciar Grey, a freshwater calving glacier in Patagonia. Geophys. Res. Lett. 46, 2602–2609. doi:10.1029/2018gl081441
Sugiyama, S., Skvarca, P., Naito, N., Enomoto, H., Tsutaki, S., Tone, K., et al. (2011). Ice speed of a calving glacier modulated by small fluctuations in basal water pressure. Nat. Geosci. 4 (9), 597–600. doi:10.1038/ngeo1218
Sutherland, J. L., Carrivick, J. L., Evans, D. J. A., Shulmeister, J., and Quincey, D. J. (2019b). The Tekapo Glacier, New Zealand, during the Last Glacial Maximum: an active temperate glacier influenced by intermittent surge activity. Geomorphology 343, 183–210. doi:10.1016/j.geomorph.2019.07.008
Sutherland, J. L., Carrivick, J. L., Gandy, N., Shulmeister, J., Quincey, D. J., and Cornford, S. L. (2020). Proglacial lakes control glacier geometry and behaviour during recession. Geophys. Res. Lett. 47, e2020GL088865. doi:10.1029/2020GL088865
Sutherland, J. L., Carrivick, J. L., Shulmeister, J., Quincey, D. J., and James, W. H. M. (2019a). Ice-contact proglacial lakes associated with the last glacial maximum across the southern Alps, New Zealand. Quat. Sci. Rev. 213, 67–92. doi:10.1016/j.quascirev.2019.03.035
Tarasov, L., and Peltier, W. R. (2006). A calibrated deglacial drainage chronology for the North American continent: evidence of an Arctic trigger for the Younger Dryas. Quat. Sci. Rev. 25 (7–8), 659–688. doi:10.1016/j.quascirev.2005.12.006
Tarasov, L., and Peltier, W. R. (2004). A geophysically constrained large ensemble analysis of the deglacial history of the North American ice-sheet complex. Quat. Sci. Rev. 23 (3–4), 359–388. doi:10.1016/j.quascirev.2003.08.004
Thompson, I., Shrestha, M., Chhetri, N., and Agusdinata, D. B. (2020). An institutional analysis of glacial floods and disaster risk management in the Nepal Himalaya. Int. J. Disaster Risk Reduct. 47, 101567. doi:10.1016/j.ijdrr.2020.101567
Thorndycraft, V. R., Bendle, J. M., Benito, G., Davies, B. J., Sancho, C., Palmer, A. P., et al. (2019). Glacial lake evolution and Atlantic-Pacific drainage reversals during deglaciation of the Patagonian Ice Sheet. Quat. Sci. Rev. 203, 102–127. doi:10.1016/j.quascirev.2018.10.036
Trüssel, B. L., Motyka, R. J., Truffer, M., and Larsen, C. F. (2013). Rapid thinning of lake-calving Yakutat glacier and the collapse of the Yakutat Icefield, southeast Alaska, USA. J. Glaciol. 59 (213), 149–161. doi:10.3189/2013j0g12j081
Truffer, M., and Motyka, R. J. (2016). Where glaciers meet water: subaqueous melt and its relevance to glaciers in various settings. Rev. Geophys. 54 (1), 220–239. doi:10.1002/2015rg000494
Tsutaki, S., Fujita, K., Nuimura, T., Sakai, A., Sugiyama, S., Komori, J., et al. (2019). Contrasting thinning patterns between lake- and land-terminating glaciers in the Bhutanese Himalaya. Cryosphere 13 (10), 2733–2750. doi:10.5194/tc-13-2733-2019
Tsutaki, S., Nishimura, D., Yoshizawa, T., and Sugiyama, S. (2011). Changes in glacier dynamics under the influence of proglacial lake formation in Rhonegletscher, Switzerland. Ann. Glaciol. 52 (58), 31–36. doi:10.3189/172756411797252194
Tsutaki, S., Sugiyama, S., Nishimura, D., and Funk, M. (2013). Acceleration and flotation of a glacier terminus during formation of a proglacial lake in Rhonegletscher, Switzerland. J. Glaciol. 59 (215), 559–570. doi:10.3189/2013jog12j107
Turzewski, M. D., Huntington, K. W., Licht, A., and Lang, K. A. (2020). Provenance and erosional impact of Quaternary megafloods through the Yarlung-Tsangpo Gorge from zircon U-Pb geochronology of flood deposits, eastern Himalaya. Earth Planet. Sci. Lett. 535, 116113. doi:10.1016/j.epsl.2020.116113
Tweed, F. S., and Carrivick, J. L. (2015). Deglaciation and proglacial lakes. Geol. Today. 31 (3), 96–102. doi:10.1111/gto.12094
Tweed, F. S., and Russell, A. J. (1999). Controls on the formation and sudden drainage of glacier-impounded lakes: implications for jökulhlaup characteristics. Prog. Phys. Geogr. Earth Environ. 23, 79–110. doi:10.1177/030913339902300104
Utting, D. J., and Atkinson, N. (2019). Proglacial lakes and the retreat pattern of the southwest Laurentide ice sheet across Alberta, Canada. Quat. Sci. Rev. 225, 106034. doi:10.1016/j.quascirev.2019.106034
Veh, G., Korup, O., and Walz, A. (2020). Hazard from Himalayan glacier lake outburst floods. Proc. Natl. Acad. Sci. U. S. A. 117 (2), 907–912. doi:10.1073/pnas.1914898117
Viani, C., Machguth, H., Huggel, C., Godio, A., Franco, D., Perotti, L., et al. (2020). Potential future lakes from continued glacier shrinkage in the Aosta valley region (western Alps, Italy). Geomorphology 355, 107068. doi:10.1016/j.geomorph.2020.107068
Walder, J. S., and Costa, J. E. (1996). Outburst floods from glacier‐dammed lakes: the effect of mode of lake drainage on flood magnitude. Earth Surf. Process. Landf.. 21 (8), 701–723. doi:10.1002/(SICI)1096-9837(199608)21:8<701::AID-ESP615>3.0.CO;2-2
Walder, J. S., Trabant, D. C., Cunico, M., Fountain, A. G., Anderson, S. P., Anderson, R. S., et al. (2006). Local response of a glacier to annual filling and drainage of an ice-marginal lake. J. Glaciol. 52 (178), 440–450. doi:10.3189/172756506781828610
Wang, X., Siegert, F., Zhou, A.-g., and Franke, J. (2013). Glacier and glacial lake changes and their relationship in the context of climate change, Central Tibetan Plateau 1972–2010. Glob. Planet. Chang. 111, 246–257. doi:10.1016/j.gloplacha.2013.09.011
Watson, C. S., Kargel, J. S., Shugar, D. H., Haritashya, U. K., Schiassi, E., and Furfaro, R. (2020). Mass loss from calving in Himalayan proglacial lakes. Front. Earth Sci. 7, 342. doi:10.3389/feart.2019.00342
Wilson, R., Glasser, N. F., Reynolds, J. M., Harrison, S., Anacona, P. I., Schaefer, M., et al. (2018). Glacial lakes of the central and patagonian andes. Glob. Planet. Chang. 162, 275–291. doi:10.1016/j.gloplacha.2018.01.004
Winsemann, J., and Lang, J. (2020). “Flooding northern Germany: impacts and magnitudes of Middle Pleistocene glacial lake-outburst floods,” in Palaeohydrology. Editors J. Herget and A. Fontana (Cham, Switzerland: Springer), 29–47
Keywords: 33 proglacial lake, ice sheet, frontal ablation, calving, glacier
Citation: Carrivick JL, Tweed FS, Sutherland JL and Mallalieu J (2020) Toward Numerical Modeling of Interactions Between Ice-Marginal Proglacial Lakes and Glaciers. Front. Earth Sci. 8:577068. doi: 10.3389/feart.2020.577068
Received: 28 June 2020; Accepted: 05 October 2020;
Published: 29 October 2020.
Edited by:
Shin Sugiyama, Hokkaido University, JapanReviewed by:
Daniel Martin, Lawrence Berkeley National Laboratory, United StatesChris D. Clark, The University of Sheffield, United Kingdom
Copyright © 2020 Carrivick, Tweed, Sutherland and Mallalieu. This is an open-access article distributed under the terms of the Creative Commons Attribution License (CC BY). The use, distribution or reproduction in other forums is permitted, provided the original author(s) and the copyright owner(s) are credited and that the original publication in this journal is cited, in accordance with accepted academic practice. No use, distribution or reproduction is permitted which does not comply with these terms.
*Correspondence: Jonathan L. Carrivick, ai5sLmNhcnJpdmlja0BsZWVkcy5hYy51aw==