- 1Department of Ocean Systems, Royal Netherlands Institute for Sea Research (NIOZ), Texel, Netherlands
- 2Department of Marine Biogeosciences, Alfred Wegener Institute, Helmholtz Centre for Polar and Marine Research, Bremerhaven, Germany
- 3MARUM – Center for Marine Environmental Sciences and Faculty of Geosciences, University of Bremen, Bremen, Germany
- 4Department of Earth Sciences, Faculty of Geosciences, Utrecht University, Utrecht, Netherlands
Element incorporation in shell calcite precipitated by foraminifera reflects the chemical and physical properties of the seawater the foraminifera lived in and can therefore be used to reconstruct paleo environmental conditions. One of the most prominent proxies for past seawater temperature is Mg/Ca of foraminiferal calcite. Still, in addition to seawater temperature, also biomineralization processes impact foraminiferal Mg/Ca values. As the impact of biomineralization plays a major role and is not necessarily constant, it is imperative to identify the mechanism by which Mg is incorporated and thereby understand how temperature influences Mg incorporation. Biomineralization is discriminating against Mg to different degrees and hence investigating the fractionation of Mg isotopes at different temperatures and for species with contrasting calcification pathways can be used to better understand the pathway of Mg during biomineralization. Overall, we observe that foraminifera with higher Mg content have δ26Mg values closer to those of seawater. Moreover, controlled temperature culture experiments show that parallel to an increase in Mg/Ca, δ26Mg in the tests of large benthic foraminifer Amphistegina lessonii decreases when sea water temperatures increase. This negative correlation between shell Mg/Ca and δ26Mg suggests a two-step control on the incorporation of Mg during biomineralization. Using a simple model, we can explain both trends as a result of a stable Mg pool, which is only little fractionated with respect to sea water and a temperature dependent Mg pool which shows a higher fractionation with respect to sea water during biomineralization. The stable, not much fractionated pool is relatively large in high Mg foraminifera, whereas for the low Mg foraminifera the transport of Mg over a cell membrane probably results in the observed inverse correlation. Here we present a model using the Mg isotope fractionation we established for A. lessonii to explain the general trends for both high- and low-Mg/Ca foraminifera. A process-based understanding remains crucial a robust interpretation of foraminiferal Mg-isotopes.
Introduction
Past climates and environments can be reconstructed using foraminiferal shell chemistry. The fractionation of many stable isotopes and incorporation of many elements (i.e., proxies) are known to be dependent on seawater chemistry and/or physical parameters. Temperature, for example, influences the foraminiferal calcite’s oxygen stable isotope ratio (δ18Ocalcite; McCrea, 1950; Urey et al., 1951) and salinity affects the Na/Ca of foraminiferal calcite (Wit et al., 2013; Allen et al., 2016; Mezger et al., 2016; Bertlich et al., 2018). With appropriate calibrations, these chemical imprints can be used to reconstruct seawater salinity and temperature and thereby allow inferring regional or global (changes in) climate. The Mg/Ca value of foraminiferal calcite reflects sea water temperature (Nürnberg et al., 1996) and can therefore be applied to fossil foraminifera to reconstruct past bottom water (Lear et al., 2000) and sea surface (Barker et al., 2005) temperatures. The exact mechanisms behind this relation between Mg content and temperature remains elusive. Even for inorganic carbonates, for which the effect of temperature on Mg/Ca has been comprehensively studied by controlled precipitation experiments (e.g., Mucci, 1987), no real consensus has been made so far. Increased precipitation rate at higher temperatures has been proposed to explain an increase in Mg incorporation at higher temperatures (Chilingar, 1962), but this has been also disputed by several studies (Mucci and Morse, 1983; Mucci et al., 1985).
Even though the fundaments behind Mg incorporation as a function of temperature in carbonates are illusive, foraminiferal Mg/Ca remains a popular proxy for temperature reconstructions (Nürnberg et al., 1996; Anand et al., 2003). There are, however, other complications that must be considered when applying this proxy. It has been shown that beside the major impact of temperature, also other variables like salinity and pH can have a minor, but measurable effect on Mg incorporation (Kisakürek et al., 2008; Allen et al., 2016; Geerken et al., 2018; Gray et al., 2018). Furthermore, foraminiferal Mg/Ca in particular is highly species-dependent, leading to species-specific Mg/Ca-temperature calibrations (Toyofuku et al., 2011). What is common for foraminifera, however, is that they incorporate (much) less Mg than is expected from inorganic (i.e., non-biological) precipitation of calcite from seawater (Bentov and Erez, 2006). The biomineralization mechanism responsible for the formation of foraminiferal chambers apparently prevents incorporation of much of the Mg present in seawater either by (active) removal or exclusion of Mg during the calcification process (Erez, 2003; Bentov and Erez, 2006; de Nooijer et al., 2014a). This strong control of foraminifera on Mg uptake or removal results in a test composition 10 to 100-fold lower in Mg/Cacalcite than expected from inorganically precipitated calcite (Zeebe and Sanyal, 2002; Segev and Erez, 2006). To illuminate the pathway of Mg during calcification and the effect of temperature on this process, we can investigate the magnesium isotopic signature of these foraminifers (δ26Mg), since selective removal or uptake of Mg would likely change Mg fractionation.
Previous measurements on foraminiferal δ26Mg include mainly small planktonic and benthic hyaline species as well as porcelaneous species (Chang et al., 2004; Pogge von Strandmann, 2008; Wombacher et al., 2011; Maeda et al., 2019). Their observations suggest that foraminiferal species with low Mg incorporation also have a relatively depleted Mg isotopic composition compared to other (biogenic) carbonates. Porcelaneous species, with a higher Mg content, have a lower magnitude of fractionation, leading to a less depleted Mg isotopic composition which is closer to other organisms, e.g., corals calcite (Pogge von Strandmann, 2008; Saulnier et al., 2012). Estimates of non-biological fractionation vary, but are in the order of −1 to −3.88‰ (Galy et al., 2002; Saulnier et al., 2012; Mavromatis et al., 2013), and could be a function of precipitation rate (Mavromatis et al., 2013). the additional fractionation by hyaline and porcelaneous foraminiferal species is −3.2 to 5.5‰ (summarized in Pogge von Strandmann, 2008). The offset in the magnitude of Mg fractionation between hyaline and porcelaneous foraminifera supports the concept that these groups precipitate their shells using contrasting calcification pathways. The largest fractionation has been found for species with a low Mg content (Wombacher et al., 2011), which may reflect the cellular mechanism that they employ to create a supersaturated fluid with considerably less Mg than that of seawater. This observation supports the hypothesis that occasional transport of Mg ions by transmembrane calcium transport (Nehrke et al., 2013; de Nooijer et al., 2017) is an important source for the Mg that is incorporated into the foraminiferal shell wall of hyaline foraminifera, since cross-membrane ion transport favours lighter isotopes over heavy ones. However, the relation between Mg content and δ26Mg needs to be studied in more detail to provide insights into processes involved in Mg uptake or removal during calcification. For this purpose, especially larger benthic foraminifera are of interest, since this group includes species which have hyaline shells but intermediate to high Mg content. This group has only been studied once before (Maeda et al., 2019) but could provide vital insights into the hyaline calcification pathway.
If the amount and isotopic composition of Mg incorporated both are determined by the same process within the biomineralization mechanism, the δ26Mg will also reflect temperature and hence may be used as a palaeoceanographic temperature proxy. First results show a negative relation between culture temperature and foraminiferal shell δ26Mg (Maeda et al., 2019), which is opposite to the relationship observed in inorganically precipitated calcite (Galy et al., 2002; Li et al., 2012), though earlier studies showed no significant impact of temperature on foraminiferal δ26Mg (Pogge von Strandmann, 2008; Wombacher et al., 2011), or inorganically precipitated calcite (Saulnier et al., 2012). If foraminiferal δ26Mg is independent of temperature but at the same time correlated to the average Mg/Ca of a species, it may constrain Mg/Ca-based temperature reconstructions based on species for which no modern-day calibration is available. This is, for example, a challenge when relying on extinct species (i.e., when reconstructing climates on longer timescales), though variability in sea water Mg isotope composition over geological time scales would have to be taken into account. To investigate the link between temperature and foraminiferal Mg/Ca and δ26Mg, we cultured the large benthic species Amphistegina lessonii at a range of temperatures (18–26°C) and investigated the relationship between δ26Mg and Mg/Ca in a range of benthic foraminiferal species.
Materials and Methods
Foraminiferal Samples
In this study we analysed specimens of Amphistegina lessonii grown under a range of controlled temperature conditions, as well as larger benthic foraminifera collected from an Indo-Pacific reef aquarium. Several species of foraminifera were selected from surface sediment samples of coral debris from the tropical reef aquarium at Royal Burgers’ Zoo in Arnhem, the Netherlands which is known for a large diversity in benthic foraminifera (Ernst et al., 2011). Specimens of A. lessonii, Heterostegina depressa, Sorites orbitolis, (Spiroloculina angluata, Spiroloculina communis, Quinqueloculina pseudoreticulina and Miliolinella labiosa were selected from the sediment to investigate the relation between Mg/Ca and δ26Mg. Due to low numbers, specimens of Spiroloculina angluata and Spiroloculina communis were combined into the group “Spiroloculina spp.”
For the temperature-controlled culture experiment, living specimens of the benthic, symbiont bearing foraminifera Amphistegina lessonii were transferred from sediment to Petri dishes and kept at constant temperature (21°C) and salinity (32) using (0.2 µm) filtered but otherwise unmodified North Atlantic Sea water as a culture medium. Specimens were fed with a solution of freeze-dried Dunaliella salina in sea water every week, and light was provided 12 h per day. These specimens were used as the parental generation for the culture experiment.
Culture Set-Up
Asexual reproductions events resulted in a large number (>30) of genetically identical offspring called a “clone group.” Two days after the reproduction events, when the juveniles had developed two to three chambers, all juveniles from the same clone group were collected and divided into three subgroups. This procedure is required to confidentially exclude genetic variability as a potential cause for any potential differences observed in the outcome of the experiment (de Nooijer et al., 2014b). Each subgroup was transferred into set-ups with one of three temperature settings (18, 21, and 26°C), while all other factors (such as food availability and salinity) are kept constant, identically to the culture conditions of the parental generation. Salinity was measured weekly and was found to be constant at 32, DIC was monitored weekly and was found to be 2,153 ± 138 µmol/L (mean ± SD) across all treatments, temperatures remained within ±0.1°C of the target values and to simulate a natural daylight cycle, light was switched on 12 h per day. Cultures were maintained for 8 wk to allow for sufficient growth, and at the end of the experiment foraminifera had a final diameter of at least 200 µm. Due to the very small proportion of carbonate in the initial two to three chambers of the foraminifera present at onset of the experiment compared to the total amount of carbonate present at the end of the experiment, it can be assumed that the impact of the initial chambers’ composition on the overall shell composition is negligible.
All specimens were then cleaned using an adapted version of the “Barker protocol” (Barker et al., 2003). In short, foraminifera were cleaned using an oxidizing step in which organics were removed with a H2O2 solution (0.5 ml 30% w/v H2O2 in 49.5 ml 0.1 M NH4OH), and consequently, after gentle ultrasonication, rinsed with ultrapure water and dried in a laminar flow cabinet and dissolved in preparation for isotope measurements. For each Mg isotope analysis about 60 µg of material, roughly six specimens per measurement, was dissolved.
Magnesium Isotope Analyses
Magnesium Isotope Analyses at MARUM
Samples from the culture experiment and the aquarium were measured on two different occasions. Samples from the Indo-Pacific aquarium at Burger’s Zoo and the inhouse Mg isotope standard NIOZ-Mg, consisting of 1,000 µg/g Mg in 5% HNO3 were measured in the Isotope Geochemistry Laboratory at MARUM, University of Bremen (Germany).
At MARUM, we followed the method of Wilckens et al. (2019) and adapted it to the sample matrix of foraminifera by using a one-step separation instead of a two-step separation. Approximately 1.5 µg Mg was loaded onto Bio-Rad BIO-spin® columns filled with 1 ml of the Bio-Rad cation exchange resin AG 50W X8 (200–400 mesh). The purification and elution of Mg was done with 0.8 M HCl. The separation is based on published distribution coefficients for the resin AG 50W X8, 200–400 mesh (after Strelow, 1960; Strelow et al., 1965). The reliability of each chemical session was checked with a procedural blank and reference materials. Mg isotope measurements were performed on a ThermoFisher Scientific Neptune Plus MC-ICP-MS equipped with a stable introduction system (SIS) and a high-efficiency x-cone in low and medium resolution as described in (Vogl et al., 2020). The purified samples were dissolved in 2% HNO3 and adjusted to 200 ng/g Mg. The measurements were done in the standard-sample bracketing method using a pure Mg ICP standard (Alfa Aesar Magnesium plasma standard solution; Specpure) as the bracketing standard. The evaluation of single measurement sequences and the conversion of the measured Mg isotope ratios into the DSM-3 scale, the international reference materials DSM-3 and Cambridge-I (Cam-I) were analysed in every sequence.
The uncertainty of the samples is reported as two standard deviations (2 sd). The instrumental precision and internal long-term repeatability for δ26Mg was ± 0.07‰ and for δ25Mg was ± 0.04‰ (2sd, n = 5), obtained by the repeated analysis of the reference material Cambridge-I (Cam-I). δ26Mg values for an internal seawater standard (bottom seawater SuSu Knolls; δ26Mg = −0.82 ± 0.08‰; δ25Mg = 0.43 ± 0.07‰; 2sd, n = 3), JCP-1 (δ26Mg = −1.99 ± 0.04‰; δ25Mg = −1.04 ± 0.08‰; 2sd, n = 4) and Cam-I (δ26Mg = −2.55 ± 0.05‰; δ25Mg = −0.44 ± 0.06‰; 2sd, n = 6) are within analytical uncertainty in agreement with literature values, calculated from published values for seawater by Foster et al. (2010; δ26Mg = −0.82 ± 0.06‰; δ25Mg = −0.43‰; 2 sd, n = 26), for JCP-1 by (Wombacher et al., 2011; δ26Mg = −2.01; δ25Mg = −1.05‰) and for Cam-I by Pogge von Strandmann et al. (2011; −2.62 ± 0.04; 2 sd, n = 43).
Magnesium Isotope Analyses at NIOZ
Samples of Amphistegina lessonii from the temperature-controlled culture experiment were measured at the Royal NIOZ, using triplicates, each consisting of 60 µg foraminiferal carbonate. Mg from the samples was purified by passing them through cation exchange columns with an aspect ratio of ∼14.2, containing 900 µL AG-50W X12 (wet) resin (Bio-Rad) following a miniaturized procedure of Pogge Von Strandmann (2008). Mg isotope ratios were determined using a Thermofinnigan Neptune Plus multicollector ICP-MS equipped with a Scott-double pass cyclonic spraychamber (SIS) equipped with a PFA-ST nebulizer using a 50 µL/min uptake capillary (ESI). For higher sensitivity, a Jet-sample cone was used in combination with a H-skimmer cone. The instrument was tuned daily for optimum signal stability with a typical sensitivity of ∼70 V/ppm (typical measurement solutions contained 100 ng/g Mg dissolved in 2% HNO3 made from 2 times sub-boiling distilled HNO3) and a background lower than 6 mV (24Mg) using Faraday collectors equipped with amplifiers using 1011 Ω resistors. Initial measurements included Ca and Na for monitoring possible matrix interferences prior the Mg isotopic analysis, but this was omitted after several analytical runs where no Ca or Na was detected in the purified samples. North Atlantic 0.2 µm filtered seawater (NASW) was included as a long-term procedure standard and as reference material in every analytical session. Samples and reference materials were measured using sample-standard bracketing against an inhouse ICP Mg (NIOZ-Mg) standard (Alpha Aeser). All solutions were matched within 10% of the Mg concentration. Values were converted to the DSM-3 scale using the NIOZ-Mg house standard, which was found to have a δ25Mg of −0.77‰ (±0.04‰, 2 sd) and δ26Mg of −1.48‰ (±0.06‰, 2 sd) relative to standard DSM-3. This was measured at MARUM (2.3.1). NASW was found to have δ25Mg of −0.44‰ (±0.02‰, 2 sd) and δ26Mg of −0.85‰ (±0.04‰, 2 sd) relative to standard DSM-3, which is very close to values reported by (Pogge von Strandmann, 2008; δ25Mg = −0.44‰ (±0.08‰, 2 sd), δ26Mg = −0.83‰ (±0.09‰, 2 sd)) and (Young and Galy, 2004; δ25Mg = −0.42‰ (±0.08‰, 2 sd), δ26Mg = −0.82‰ (±0.04‰, 2 sd)). Measured isotope ratios were converted to δ25Mg and δ26Mg values using the following equation
where
Comparing these measured and corrected values with δ25Mg and δ26Mg values of other marine carbonates (Figure 1), all δ25Mg and δ26Mg follow the same linear relationship, the terrestrial line, proving the internal consistency of this new data set.
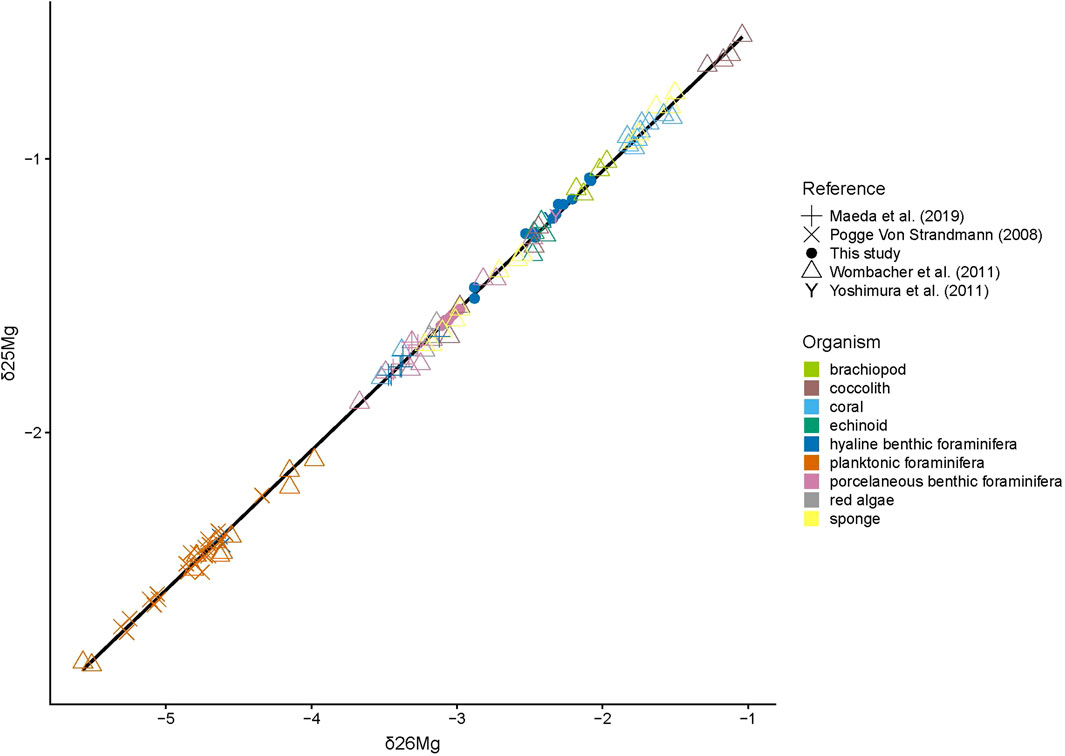
FIGURE 1. The relationship between δ25Mg and δ26Mg values in our dataset combined with published data from other biogenic carbonates (Pogge von Strandmann, 2008; Wombacher et al., 2011; Yoshimura et al., 2011; Maeda et al., 2019) can be described as δ25Mg = 0.510* δ26Mg − 0.024 (p value < 2e−16; R2 = 0.998).
Potential CN interferences, while technically possible due to contamination by carbon from the atmosphere or from the resin used are highly unlikely. Standard, blanks and sample measurements would be affected by atmospheric carbon alike, considering the stability of atmospheric CO2 concentrations on such short time scales as the measurements for this study. If carbon would have originated from the resin, this would likely be accompanied by additional problems such as polymers clogging up the sampling system. Such issues have not been observed during the measurements, and we thus assume no significant interferences due to the resin. Nitrate from the acid used represents a potential source of N, though since all solutions were diluted from a single batch of acid, this would also lead to a constant impact across all measurements. The peak-stripping procedure performed removes this potential CN interference, since these would introduce constant contributions to all mass 26 measurements.
All statistical analysis was performed using RStudio Version 1.1.453.
Results
δ25Mg and δ26Mg Values and Derived Mg/Ca From the Temperature Experiment
The culture medium was found to have δ25Mg and δ26Mg values of −0.44‰ and −0.81‰ (relative to DSM-3), respectively, which corresponds to open ocean seawater (Young and Galy, 2004; Ra and Kitagawa, 2007; Pogge von Strandmann, 2008; Foster et al., 2010). Values for δ25Mg and δ26Mg measured in the cultured foraminifera range from −1.29 to −1.15‰ and −2.48 to −2.21‰, respectively, (Table 3). The δ26Mg values show a strong, negative correlation with temperature in A. lessonii (δ26Mg = −0.03 (±0.003 se) * T − 1.66 (±0.07 se); p-value < 0.01, adjusted R2 = 0.94; Figure 2).
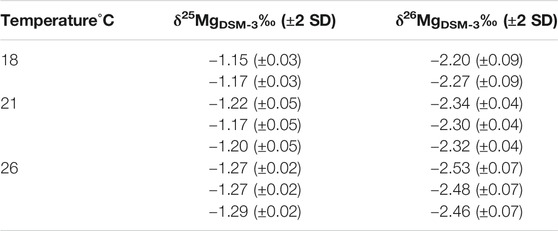
TABLE 3. Mean δ25Mg and δ26Mg values (±2 standard deviations; SD) relative to DSM-3 measured in Amphistegina lessonii cultured at different temperatures.
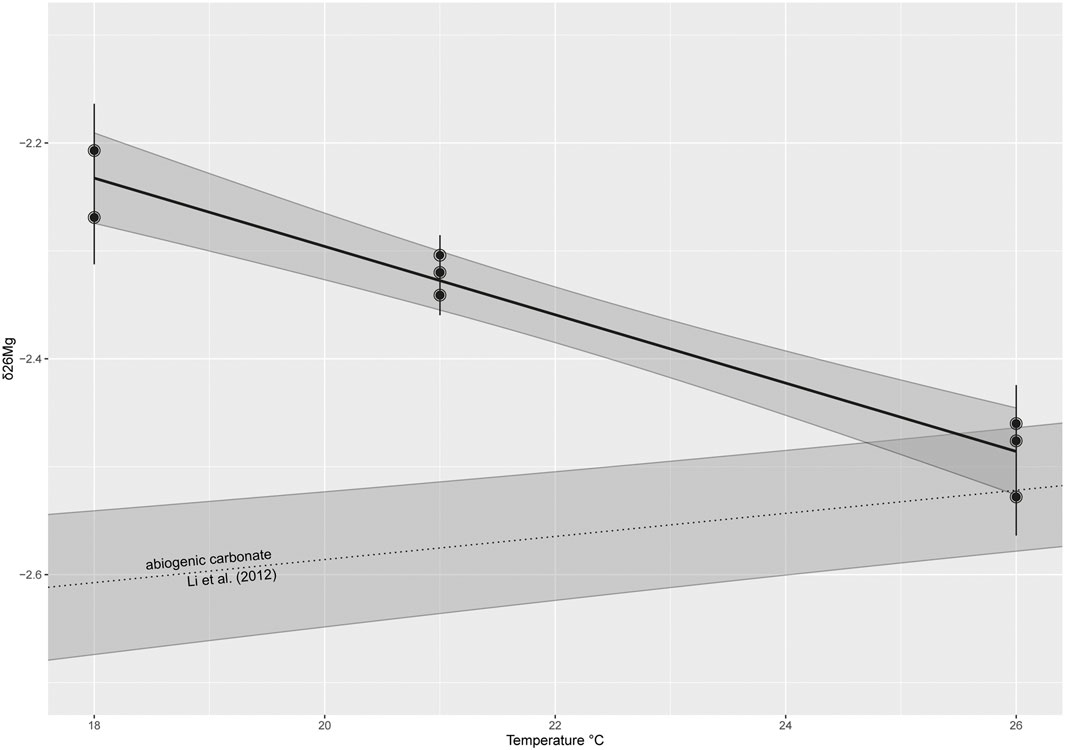
FIGURE 2. δ26Mg values (±SD) measured in foraminiferal calcite of cultured Amphistegina lessonii shows a strong, negative correlation with temperature. Since temperature was a controlled variable, errorbars are within the width of the symbol. The grey area indicates the 95% confidence interval. The relationship observed can be described as δ26Mg = −0.03 * T − 1.66 (p-value < 0.001, adjusted R2 = 0.93). This relationship is opposite to the one observed in some abiogenic carbonates (Li et al., 2012).
The Mg/Ca values of the foraminifera was calculated using a species-specific temperature calibration (van Dijk et al., 2019b), resulting in Mg/Ca ratios of 13.9, 19.0, and 27.4 mmol/mol for the specimens stemming from the 18, 21, and 26°C treatment, respectively.
Mg Isotopic Composition of Foraminifera From the Indo-Pacific Aquarium
The Mg-isotopic fractionation between species derived from the coral debris collected from the aquarium (kept at 26.0 ± 0.5°C during the last 20 yr; Ernst et al., 2011) varies greatly between species, though the variability within the hyaline foraminifera appears to be larger than within the analysed species of porcelaneous foraminifera (Table 4). Mean δ26MgDSM-3±2 SD of the foraminiferal samples varies between −2.09 ± 0.09‰ and −2.88 ± 0.03‰ for the investigated hyaline species (Amphistegina lessonii and Heterostegina depressa) and ranges from −3.08 (±0.07) to −3.00 (±0.04) for the porcelaneous species (Sorites orbitolis, Spiroculina spp., Quinqueloculina pseudoreticulina, Miliolinella labiosa).

TABLE 4. Mean δ25Mg and δ26Mg values (±2 standard deviations; SD) relative to DSM-3 of a variety of benthic foraminiferal species collected from coral debris of the Indo-Pacific aquarium of Burgers’ Zoo, Netherlands.
Discussion
δ26Mg in Marine Calcifiers
A wide range in values in magnesium isotope ratios has been observed for marine calcifiers. Earlier studies on the relationship between temperature and δ26Mg values of biogenic and abiogenic calcium carbonate showed highly variable Mg isotope ratios and trends (Figure 3). For sponges, a slight positive correlation with temperature is observed, though different species show different response to both absolute temperature as well as changes in sea water temperature. Previously published δ26Mg values for planktonic foraminifera range from −4 to −5.5‰ (Chang et al., 2004; Pogge von Strandmann, 2008; Wombacher et al., 2011), showing a slight negative relationship with temperature, which is not present when considering individual species. Although the isotopic composition of Mg in sea water is assumed to be rather uniform based on the residence time of Mg in seawater which is several orders of magnitude higher than ocean mixing time (Foster et al., 2010; Lécuyer, 2016), different biotic and abiotic processes result in highly variable isotope fractionation. Variability could be caused by kinetic effects or differences in precipitation rates (Immenhauser et al., 2010; Mavromatis et al., 2013) or by microscopic fluid inclusions that have been observed in inorganic calcite growth experiments (Saulnier et al., 2012). While fluid inclusions so far have not been observed in biogenic calcite, precipitation rates among marine calcifiers vary (e.g., Ullmann, 2016) and are known to impact the isotopic composition of calcium carbonates (DePaolo, 2011). Well established estimates of carbonate precipitation rates would therefore be a valuable addition to take into account and could allow inter-taxa comparisons as well as deepen our understanding of Mg isotope incorporation, such data is unfortunately very difficult to obtain. The largest differences in fractionation are most likely due to biogenic processes though considering the large differences observed between organisms even under similar temperatures. Such a biogenic contribution to the overall Mg isotope fractionation is apparently variable amongst organisms and between species as δ26Mg can vary up to 4‰. For example, δ26Mg values for coccolithophores are reported as high as −1‰ (relative to DSM-3), whereas planktonic foraminifera have values as low as −5.5‰ (Chang et al., 2004; Pogge von Strandmann, 2008; Wombacher et al., 2011).
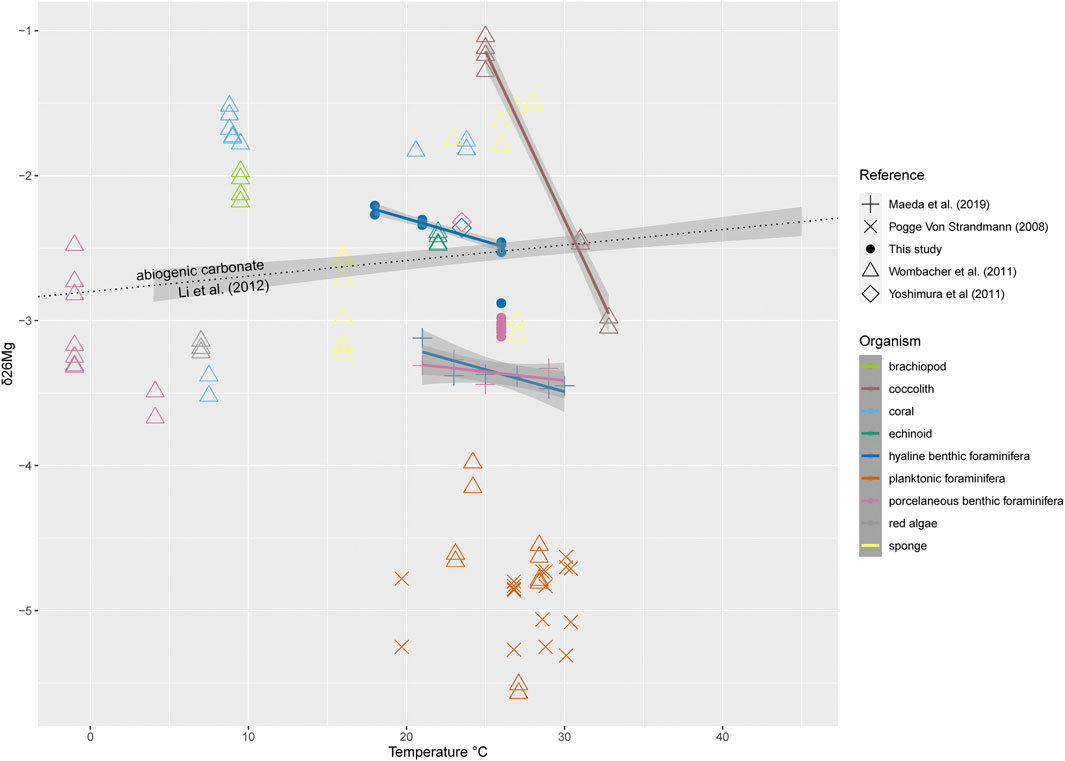
FIGURE 3. δ26Mg values versus temperature for different organisms from literature data (Maeda et al., 2019; Pogge von Strandmann, 2008; Wombacher et al., 2011; Yoshimura et al., 2011) and this study, showing different responses between groups of organisms. In addition to species specific relationships in benthic foraminifera (see Figure 1), a statistically significant relationship (p-values < 0.05) can be observed for coccoliths (δ26Mg = −0.23*T+ 4.66, R2 = 0.98), and sponges (δ26Mg = 0.07*T − 4.0, R2 = 0.34), while other groups such as corals show no clear trend and the δ26Mg values in abiogenic carbonates rise with temperature (Li et al., 2012). Error bars for Amphistegina lessonii are shown in Figure 2.
Abiogenic carbonate shows δ26Mg values between −2.8 and −2.4‰ with a slight positive correlation between temperature and both Mg incorporation and δ26Mg (Li et al., 2012): i.e., more Mg is incorporated at higher temperature, and the Mg is at the same time less depleted with respect to heavy isotopes. In contrast a strong negative relationship of δ26Mg with temperature has been observed for coccoliths (Wombacher et al., 2011). Since all coccolith samples were collected from the field, it was suggested by Wombacher et al. (2011) that the observed trend may, however, have been indirect. Here we observe a similar trend for intermediate Mg/Ca foraminifera from controlled growth experiments, suggesting that temperature somehow affects Mg isotopes in some marine calcifiers. Since biomineralization processes vary largely between taxa, conclusions drawn from foraminifera presented here cannot be transferred to other organisms without caution though.
δ26Mg in Foraminiferal Calcite and the Effect of Temperature
Results from previous studies combined with ours show that foraminiferal δ26Mg values range between −5.5 and −1‰ (DSM-3; Chang et al., 2004; Maeda et al., 2019; Pogge von Strandmann, 2008; Wombacher et al., 2011; Yoshimura et al., 2011; Young and Galy, 2004). To investigate the relation between δ26Mg and Mg content, we plotted data from our study as well as previous studies, of which both these parameters were known or calculated through well-established calibrations, in Figure 4. On a species level, the δ26Mg values decrease with increasing Mg content, although the range in fractionation itself increases at lower Mg/Ca values. This suggests that for species with high Mg/Ca calcite shells, precipitation resembles more closely inorganic precipitation from an open sea water reservoir, whereas at low Mg/Ca values (i.e., when organisms discriminate against Mg-incorporation) fractionation can be either close to inorganic values (open system) or there may be strong offsets from sea water towards more negative values. This implies that the observed range at low Mg/Ca reflects mainly the biological control and to a lesser extend the inorganic fractionation effects, viz. for example the large range in δ26Mg for planktonic foraminifera at relatively uniform Mg/Ca values (Figure 4; Wombacher et al., 2011; Yoshimura et al., 2011).
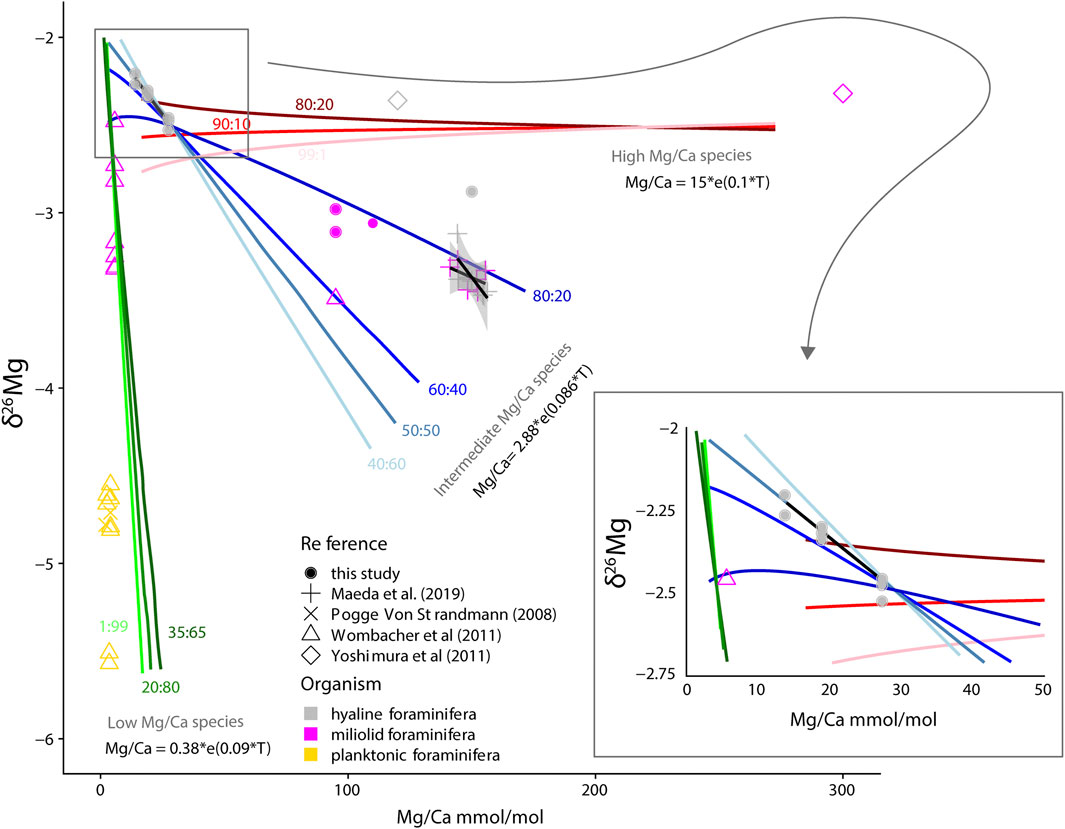
FIGURE 4. Modelled Mg/Ca versus δ26Mg calculated over a range of temperatures using the species-specific temperature calibrations for a number of (hypothetical) species (red, blue and green lines). Lines indicate isotopic signatures as a consequence of different relative amounts of low versus high Mg/Ca bands (high:low). Fitted calibration lines (black), including uncertainty intervals are added for A. lessonii (this study) as well as A. kudakajimensis and C. gaudichaudii (Maeda et al., 2019). Also included is published data (Pogge von Strandmann, 2008; Wombacher et al., 2011; Yoshimura et al., 2011; Maeda et al., 2019) for comparison with the model output. When information about Mg/Ca was not available from the same study as the δ26Mg values, Mg/Ca was estimated using accompanying temperature information and published calibrations (Nürnberg et al., 1996; Anand et al., 2003; Raja et al., 2007; Sadekov et al., 2014; de Nooijer et al., 2017). Mg/Ca of specimens from the controlled culture experiment are based on the Mg/Ca-temperature calibration of van Dijk et al. (2019b).
We show a distinct negative relation (slope −0.03‰/°C) between foraminiferal δ26Mg and Mg/Ca for the hyaline species A. lessonii (Figure 2). The overall trend is about three times more sensitive as observed for porcelaneous Amphisorus kudakajimensis (slope −0.01‰/°C; Maeda et al., 2019). Such a difference most likely reflects a difference in the biomineralization between these species, which is also evident in the contrasting Mg/Ca of these species: A. kudakajimensis typically has Mg/Ca ratios of ∼150 mmol/mol, whereas A. lessonii has an order of magnitude lower Mg/Ca ratio, typically of 20–30 mmol/mol (e.g., van Dijk et al., 2019a; Geerken et al., 2019). The sensitivity of the isotopic composition of incorporated Mg to temperature also varies between these species: A. lessonii being less sensitive (slopes of the calibration regression being 1.69 mmol/mol/°C in A. lessonii (van Dijk et al., 2019b) compared to 2.74 mmol/mol/°C in A. kudakajimensis (Maeda et al., 2017). Overall, the isotopic sensitivity seems to increase with organismal fractionation against Mg during biomineralization.
The higher temperature sensitivity of both Mg/Ca and Mg isotopes in A. lessonii suggests that the incorporation and fractionation of Mg is intrinsically coupled, which is supported by the observation that most isotopically depleted values are associated with species most depleted in Mg (Figure 4). The process-based explanation for the observed relation between Mg incorporated and the isotopic fractionation will also have to relate to the banded within-wall Mg distribution, which occurs in alternating low- and high-concentration bands in many benthic and planktonic species (Erez, 2003; Sadekov et al., 2005; Bentov and Erez, 2006; Steinhardt et al., 2015; Fehrenbacher et al., 2017). Although the exact mechanism responsible for Mg-transport during calcification remains to be fully determined, it is generally thought that both precipitation from seawater and from a Mg-depleted fluid are involved. In many reports, the higher Element/Ca ratio (El/Ca) of elements such as Mg, Na or K are found in the first layers precipitated and the lower El/Ca bands are precipitated later (e.g., van Dijk et al., 2019a; Geerken et al., 2019). There are some exceptions with reports on highest El/Ca at the end of chamber wall formation (Jonkers et al., 2016) or alternations of high- and low-El/Ca bands in the spherical chamber of Orbulina universa (Spero et al., 2015). Here we use these observations and recent concepts in foraminiferal biomineralization models to explore the coupled Mg/Ca and Mg isotope behaviour with a simple two-endmember-mixing model resembling foraminiferal chamber formation.
Foraminiferal Mg Incorporation During Biomineralization
Calcifying perforate foraminifera form an organic, protective envelope around their shell that shields the site of calcification from the direct environment and allows for chemical manipulation, such as increasing the pH of the calcifying fluid (Erez, 2003; Jørgensen et al., 1985; de Nooijer et al., 2009). The Mg isotope signal of the first calcium carbonate formed during biomineralization likely represents that of inorganic calcium carbonate formed from the sea water trapped by the protective envelope (Figure 5). As the newly formed carbonate layer grows, more Ca2+ ions are transported from the sea water to the site of calcification through selective calcium ion channels in the protective envelope. While these channels mainly transport calcium ions, they likely bring a small amount of Mg2+ ions into the site of calcification. While these transmembrane transport channels have not yet been identified in foraminifera, they have long been suspected (Nehrke et al., 2013; Marchitto et al., 2018) and Ca-ATPases with a strong Ca-selectivity over Mg2+ are known from other organisms (Drake et al., 1996; Xiang et al., 2007). It is also likely that as this transport happens, these channels also discriminate against heavier Mg isotopes, which leads to an increasingly depleted δ26Mg signal being recorded in the calcium carbonate. Channel activity and/or selectivity could vary as a function of temperature. Bulk shell δ26Mg represents a mixed signal, consisting of the initial layer of carbonate, formed from the sea water Mg ions at the start of calcification, and the later leaked-in Mg ions transported through the discriminating ion channels. The bulk shell δ26Mg therefore depends on the isotopic composition of the sea water (determining the first endmember), the fractionation caused by the ion channels (determining the second endmember) as well as the ratio of initial to derived carbonate formed during calcification, which determines mixing of the endmembers. Both endmembers differ in the process that supplies the Mg2+ to the calcifying fluid, but once the Mg has arrived in the calcifying fluid, precipitation of both endmembers will be impacted by the same processes that also affect inorganic calcite precipitation such as Rayleigh fractionation. As modelled results show, the effect of Rayleigh fractionation on the bulk shell δ26Mg signal would cause a slightly, but significantly positive correlation with temperature (Pogge von Strandmann, 2008), which is the opposite of the trend observed here. It can therefore be concluded that the biogenic effect caused by the transmembrane transport is large enough to overcome possible effects caused by Rayleigh fractionation and still be measurable. Rayleigh fractionation therefore only plays a minor role in foraminiferal δ26Mg signals, which appear to be largely controlled by transmembrane transport, similarly to foraminiferal Mg/Ca.
In our model, for simplicity, we assume that species primarily differ in their average Mg/Ca as a consequence of the ratio of seawater- and ion-pump derived Mg. Species with calcite Mg/Ca values of >150 mmol/mol likely precipitate from a solution with a seawater-like Mg concentration (e.g., miliolids or high-Mg/Ca larger benthic foraminifers). Species with lowest Mg/Ca values (i.e., ∼1–5 mmol/mol), make most of their carbonate with ion-pump derived Ca and hence also (depleted) Mg. Using the here measured effect of temperature on the Mg/Ca-δ26Mg relationship for A. lessonii, and assuming a 50/50 ratio of Mg in low and high-Mg carbonate bands (Geerken et al., 2019) allows deconvolving the isotope values of both endmembers as function of temperature. Using EPMA analyses, Geerken et al. (2019) established that roughly 50% of the Mg is part of the high Mg-carbonate and the remaining 50% is part of the low Mg carbonate in A. lessonii. Moreover, average Mg/Ca for A. lessonii can be described as (van Dijk et al., 2019b; Geerken et al., 2019):
Where
Where
With the δ26Mg/temperature-dependency of inorganically precipitated calcite (Li et al., 2012), the δ26Mg of the high-Mg/Ca band can hence be approximated:
And the δ26Mglow band is simply:
The δ26Mg of the calculated low-Mg/Ca band then can be used to calculate the possible Mg/Ca- δ26Mg for other species with a different Mg/Ca-T calibration and/or species with a considerably lower or higher Mg/Ca. First, applying different relative contributions of the low and high Mg/Ca bands clearly show that this mixing model explains the values observed for A. lessonii, and also can be extended to A. kudakajimensis appeared and C. gaudichaudii using a relative contribution of 80% of high Mg carbonate. This higher relative contribution is in line with the higher overall Mg/Ca values for these species.
Figure 4 shows a number of possible, modelled Mg/Ca-δ26Mg relationships for a range of temperatures for species with fundamentally different Mg-incorporation. The other Mg/Ca-T calibrations are here assumed to resemble those of planktonic species (i.e., Mg/Ca = 0.38*e(0.09*T), Anand et al., 2003) and those of high-Mg/Ca species (i.e., Mg/Ca = 15*e(0.1*T), cf. Wit et al., 2012). This essentially renders the Mg/Ca-δ26Mg relationship a function of p (i.e., the proportion of Mg in the high-Mg/Ca band).
The two end-member model presented here, which incorporates the earlier established inorganic fractionation and the biological process which either pumps in Ca and/or removes Mg from the SOC, can not only explain the temperature dependance we observed for A. lessonii, but also the differences between the different species. When all these organisms or foraminifera would use the same ion pump mechanism one would expect a single correlation between Mg/Ca and δ26Mg. This is clearly not what is observed here. Although the fact that at high Mg/Ca values δ26Mg consistently plots close to the inorganic values, whereas at low Mg/Ca values a large range in δ26Mg is observed implies a major biological imprint, using two different phases only suffices to explain all data measured until now for foraminifera. Clearly this does not imply that these two processes are the only ones involved in Mg incoporation and hence fractionation. The offsets between species in our model with a variable fractionation during ion transport for the low Mg/Ca phase does highlight the importance of ion transport during foraminiferal calcification. From a proxy-perspective, the δ26Mg adds to the mechanistic understanding of the relation between Mg/Ca, temperature and biomineralization.
Conclusion
Here we tested the effect of temperature on δ26Mg in the large benthic, symbiont bearing foraminifera Amphistegina lessonii. Results show a significant negative relationship with higher temperatures leading to a more negative isotopic signal in the foraminiferal calcite. This phenomenon can be explained by a combination of two pathways that lead to the incorporation of Mg into the carbonate. Initially formed carbonate comprises Mg2+ ions stemming from the sea water trapped by the protective envelope surrounding the foraminifera during calcification. This isotopic signal gets modified by new Mg2+ ions reaching the site of calcification through Ca2+ ion channels that discriminate against heavier isotopes and thus cause fractionation. The former process explains the Mg isotopes in the high-Mg band commonly found in Rotaliid foraminifera and follows the inorganic δ26Mg-temperature relationship. The second process adds a less depleted phase to the shell wall. Inter-species differences in both average δ26Mg and Mg/Ca can thus be explained by differences in relative contribution of these two phases.
Data Availability Statement
The original contributions presented in the study are included in the article/Supplementary Material, further inquiries can be directed to the corresponding author.
Author Contributions
The study was designed by LD, ID, LN, and GR. The temperature experiment was prepared and performed by LD and BZ, the geochemical analyses at NIOZ were done by BW and BZ. Geochemical analyses at MARUM were performed by FW, the species identification and sample preparation were done by ID. The model was developed by GR with input from LN and LD, the manuscript was drafted by LD with contributions from all authors.
Funding
This work was carried out under the program of the Netherlands Earth System Science Centre (NESSC), financially supported by the Ministry of Education, Culture and Science (OCW) gravitational grant 024.002.001. ID acknowledges funding from the Alexander von Humboldt-Foundation.
Conflict of Interest
The authors declare that the research was conducted in the absence of any commercial or financial relationships that could be construed as a potential conflict of interest.
Acknowledgments
We thank Max Janse (Royal Burgers’ Zoo Arnhem, the Netherlands) for providing the coral debris and living foraminifera. We are grateful for Jordi Beunk’s (Utrecht University) vital support during the temperature experiment and thank Siham de Goeyse (NIOZ) for her assistance in hand selecting suitable specimens for the experiment’s parental generation. We thank Karel Bakker for performing weekly DIC and salinity measurements. We would also like to thank Prof Simone Kasemann (MARUM) and Laurent Devriendt (NIOZ) for helpful comments and discussions during this project.
References
Allen, K. A., Hönisch, B., Eggins, S. M., Haynes, L. L., Rosenthal, Y., and Yu, J. (2016). Trace Element Proxies for Surface Ocean Conditions: A Synthesis of Culture Calibrations with Planktic Foraminifera. Geochimica et Cosmochimica Acta 193, 197–221. doi:10.1016/j.gca.2016.08.015
Anand, P., Elderfield, H., and Conte, M. H. (2003). Calibration of Mg/Ca Thermometry in Planktonic Foraminifera from a Sediment Trap Time Series. Paleoceanography 18 (2), 1050. doi:10.1029/2002PA000846
Barker, S., Cacho, I., Benway, H., and Tachikawa, K. (2005). Planktonic Foraminiferal Mg/Ca as a Proxy for Past Oceanic Temperatures: A Methodological Overview and Data Compilation for the Last Glacial Maximum. Quat. Sci. Rev. 24 (7-9), 821–834. doi:10.1016/j.quascirev.2004.07.016
Barker, S., Greaves, M., and Elderfield, H. (2003). A Study of Cleaning Procedures Used for Foraminiferal Mg/Ca Paleothermometry. Geochem. Geophys. Geosyst. 4 (9), 8407. doi:10.1029/2003GC000559
Bentov, S., and Erez, J. (2006). Impact of Biomineralization Processes on the Mg Content of Foraminiferal Shells: A Biological Perspective. Geochem. Geophys. Geosyst. 7 (1), Q01P08. doi:10.1029/2005GC001015
Bertlich, J., Nürnberg, D., Hathorne, E. C., de Nooijer, L. J., Mezger, E. M., Kienast, M., et al. (2018). Salinity Control on Na Incorporation into Calcite Tests of the Planktonic Foraminifera Trilobatus Sacculifer - Evidence from Culture Experiments and Surface Sediments. Biogeosciences 15 (20), 5991–6018. doi:10.5194/bg-15-5991-2018
Chang, V. T.-C., Williams, R. J. P., Makishima, A., Belshawl, N. S., and O’Nions, R. K. (2004). Mg and Ca Isotope Fractionation during CaCO3 Biomineralisation. Biochem. Biophysical Res. Commun. 323 (1), 79–85. doi:10.1016/j.bbrc.2004.08.053
Chilingar, G. V. (1962). Dependence on Temperature of Ca/Mg Ratio of Skeletal Structures of Organisms and Direct Chemical Precipitates Out of Sea Water. Bull. South. Calif. Acad. Sci. 61, 45–61.
de Nooijer, L. J., Hathorne, E. C., Reichart, G. J., Langer, G., and Bijma, J. (2014b). Variability in Calcitic Mg/Ca and Sr/Ca Ratios in Clones of the Benthic Foraminifer Ammonia Tepida. Mar. Micropaleontol. 107, 32–43. doi:10.1016/j.marmicro.2014.02.002
de Nooijer, L. J., Spero, H. J., Erez, J., Bijma, J., and Reichart, G. J. (2014a). Biomineralization in Perforate Foraminifera. Earth-Science Rev. 135, 48–58. doi:10.1016/j.earscirev.2014.03.013
de Nooijer, L. J., Toyofuku, T., and Kitazato, H. (2009). Foraminifera Promote Calcification by Elevating Their Intracellular pH. Proc. Natl. Acad. Sci. 106, 15374–15378. doi:10.1073/pnas.0904306106
de Nooijer, L. J., van Dijk, I., Toyofuku, T., and Reichart, G. J. (2017). The Impacts of Seawater Mg/Ca and Temperature on Element Incorporation in Benthic Foraminiferal Calcite. Geochem. Geophys. Geosyst. 18 (10), 3617–3630. doi:10.1002/2017GC007183
DePaolo, D. J. (2011). Surface Kinetic Model for Isotopic and Trace Element Fractionation during Precipitation of Calcite from Aqueous Solutions. Geochimica et Cosmochimica Acta 75 (4), 1039–1056. doi:10.1016/j.gca.2010.11.020
Drake, S. K., Lee, K. L., and Falke, J. J. (1996). Tuning the Equilibrium Ion Affinity and Selectivity of the EF-Hand Calcium Binding Motif: Substitutions at the Gateway Position†. Biochemistry 35, 6697–6705. doi:10.1021/bi952430l
Erez, J. (2003). The Source of Ions for Biomineralization in Foraminifera and Their Implications for Paleoceanographic Proxies. Rev. Mineralogy Geochem. 54 (1), 115–149. doi:10.2113/0540115
Ernst, S., Janse, M., Renema, W., Kouwenhoven, T., Goudeau, M.-L., and Reichart, G.-J. (2011). Benthic Foraminifera in a Large Indo-Pacific Coral Reef Aquarium. J. Foraminiferal Res. 41 (2), 101–113. doi:10.2113/gsjfr.41.2.101
Fehrenbacher, J. S., Russell, A. D., Davis, C. V., Gagnon, A. C., Spero, H. J., Cliff, J. B., et al. (2017). Link between Light-Triggered Mg-Banding and Chamber Formation in the Planktic Foraminifera Neogloboquadrina Dutertrei. Nat. Commun. 8 (May), 1–10. doi:10.1038/ncomms15441
Foster, G. L., Pogge von Strandmann, P. A. E., and Rae, J. W. B. (2010). Boron and Magnesium Isotopic Composition of Seawater. Geochem. Geophys. Geosyst. 11 (8), Q08015. doi:10.1029/2010GC003201
Galy, A., Bar-Matthews, M., Halicz, L., and O’Nions, R. K. (2002). Mg Isotopic Composition of Carbonate: Insight from Speleothem Formation. Earth Planet. Sci. Lett. 201 (1), 105–115. doi:10.1016/S0012-821X(02)00675-1
Geerken, E., de Nooijer, L. J., Roepert, A., Polerecky, L., King, H. E., and Reichart, G. J. (2019). Element Banding and Organic Linings within Chamber walls of Two Benthic Foraminifera. Sci. Rep. 9 (1), 3598. doi:10.1038/s41598-019-40298-y
Geerken, E., de Nooijer, L. J., van Dijk, I., and Reichart, G.-J. (2018). Impact of Salinity on Element Incorporation in Two Benthic Foraminiferal Species with Contrasting Magnesium Contents. Biogeosciences 15 (7), 2205–2218. doi:10.5194/bg-15-2205-2018
Gray, W. R., Weldeab, S., Lea, D. W., Rosenthal, Y., Gruber, N., Donner, B., et al. (2018). The Effects of Temperature, Salinity, and the Carbonate System on Mg/Ca in Globigerinoides Ruber (white): A Global Sediment Trap Calibration. Earth Planet. Sci. Lett. 482, 607–620. doi:10.1016/j.epsl.2017.11.026
Immenhauser, A., Buhl, D., Richter, D., Niedermayr, A., Riechelmann, D., Dietzel, M., et al. (2010). Magnesium-isotope Fractionation During Low-Mg Calcite Precipitation in a Limestone Cave - Field Study and Experiments. Geochimica et Cosmochimica Acta 74 (15), 4346–4364. doi:10.1016/j.gca.2010.05.006
Jonkers, L., Buse, B., Brummer, G.-J. A., and Hall, I. R. (2016). Chamber Formation Leads to Mg/Ca Banding in the Planktonic Foraminifer Neogloboquadrina Pachyderma. Earth Planet. Sci. Lett. 451, 177–184. doi:10.1016/j.epsl.2016.07.030
Jørgensen, B. B., Erez, J., Revsbech, P., and Cohen, Y. (1985). Symbiotic Photosynthesis in a Planktonic Foraminiferan, Globigerinoides Sacculifer (Brady), Studied with Microelectrodes1. Limnol. Oceanogr. 30 (6), 1253–1267. doi:10.4319/lo.1985.30.6.1253
Kisakürek, B., Eisenhauer, A., Böhm, F., Garbe-Schönberg, D., and Erez, J. (2008). Controls on Shell Mg/Ca and Sr/Ca in Cultured Planktonic Foraminiferan, Globigerinoides Ruber (white). Earth Planet. Sci. Lett. 273 (3–4), 260–269. doi:10.1016/j.epsl.2008.06.026
Lear, C. H., Elderfield, H., and Wilson, P. A. (2000). Cenozoic Deep-Sea Temperatures and Global Ice Volumes from Mg/Ca in Benthic Foraminiferal Calcite. Science (80) 287 (5451), 269–272. doi:10.1126/science.287.5451.269
Lécuyer, C. (2016). Seawater Residence Times of Some Elements of Geochemical Interest and the Salinity of the Oceans. Bull. de la Société Géologique de France 187 (6), 245–260. doi:10.2113/gssgfbull.187.6.245
Li, W., Chakraborty, S., Beard, B. L., Romanek, C. S., and Johnson, C. M. (2012). Magnesium Isotope Fractionation during Precipitation of Inorganic Calcite under Laboratory Conditions. Earth Planet. Sci. Lett. 333-334, 304–316. doi:10.1016/j.epsl.2012.04.010
Maeda, A., Fujita, K., Horikawa, K., Suzuki, A., Yoshimura, T., Tamenori, Y., et al. (2017). Evaluation of Oxygen Isotope and Mg/Ca Ratios in High-Magnesium Calcite from Benthic Foraminifera as a Proxy for Water Temperature. J. Geophys. Res. Biogeosci. 122 (1), 185–199. doi:10.1002/2016JG003587
Maeda, A., Yoshimura, T., Araoka, D., Suzuki, A., Tamenori, Y., Fujita, K., et al. (2019). Magnesium Isotopic Composition of Tests of Large Benthic Foraminifers: Implications for Biomineralization. Geochem. Geophys. Geosyst. 20 (8), 4046–4058. doi:10.1029/2019GC008314
Marchitto, T. M., Bryan, S. P., Doss, W., McCulloch, M. T., and Montagna, P. (2018). A Simple Biomineralization Model to Explain Li, Mg, and Sr Incorporation into Aragonitic Foraminifera and Corals. Earth Planet. Sci. Lett. 481, 20–29. doi:10.1016/j.epsl.2017.10.022
Mavromatis, V., Gautier, Q., Bosc, O., and Schott, J. (2013). Kinetics of Mg Partition and Mg Stable Isotope Fractionation during its Incorporation in Calcite. Geochimica et Cosmochimica Acta 114, 188–203. doi:10.1016/j.gca.2013.03.024
McCrea, J. M. (1950). On the Isotopic Chemistry of Carbonates and a Paleotemperature Scale. J. Chem. Phys. 18 (6), 849–857. doi:10.1063/1.1747785
Mezger, E. M., Nooijer, L. J., Boer, W., Brummer, G. J. A., and Reichart, G. J. (2016). Salinity Controls on Na Incorporation in Red Sea Planktonic Foraminifera. Paleoceanography 31 (12), 1562–1582. doi:10.1002/2016PA003052
Mucci, A. (1987). Influence of Temperature on the Composition of Magnesian Calcite Overgrowths Precipitated from Seawater. Geochim. Cosmochim. Acta 51, 1977–1984. doi:10.1016/0016-7037(87)90186-4
Mucci, A., Morse, J. W., and Kaminsky, M. S. (1985). Auger Spectroscopy Analysis of Magnesian Calcite Overgrowths Precipitated from Seawater and Solutions of Similar Composition. Am. J. Sci. 285 (4), 289–305. doi:10.2475/ajs.285.4.289
Mucci, A., and Morse, J. W. (1983). The Incorporation of Mg2+ and Sr2+ into Calcite Overgrowths: Influences of Growth Rate and Solution Composition. Geochimica et Cosmochimica Acta 47 (2), 217–233. doi:10.1016/0016-7037(83)90135-7
Nehrke, G., Keul, N., Langer, G., de Nooijer, L. J., Bijma, J., and Meibom, A. (2013). A New Model for Biomineralization and Trace-Element Signatures of Foraminifera Tests. Biogeosciences 10 (10), 6759–6767. doi:10.5194/bg-10-6759-2013
Nürnberg, D., Bijma, J., and Hemleben, C. (1996). Assessing the Reliability of Magnesium in Foraminiferal Calcite as a Proxy for Water Mass Temperatures. Geochimica et Cosmochimica Acta 60 (5), 803–814. doi:10.1016/0016-7037(95)00446-7
Pogge von Strandmann, P. A. E., Elliott, T., Marschall, H. R., Coath, C., Lai, Y.-J., Jeffcoate, A. B., et al. (2011). Variations of Li and Mg Isotope Ratios in Bulk Chondrites and Mantle Xenoliths. Geochimica et Cosmochimica Acta 75 (18), 5247–5268. doi:10.1016/j.gca.2011.06.026
Pogge von Strandmann, P. A. E. (2008). Precise Magnesium Isotope Measurements in Core Top Planktic and Benthic Foraminifera. Geochem. Geophys. Geosyst. 9 (12), Q12015. doi:10.1029/2008GC002209
Ra, K., and Kitagawa, H. (2007). Magnesium Isotope Analysis of Different Chlorophyll Forms in Marine Phytoplankton Using Multi-Collector ICP-MS. J. Anal. Spectrom. 22 (7), 817–821. doi:10.1039/b701213f
Raja, R., Saraswati, P. K., and Iwao, K. (2007). A Field-Based Study on Variation in Mg/Ca and Sr/Ca in Larger Benthic Foraminifera. Geochem. Geophys. Geosyst. 8 (10), Q10012. doi:10.1029/2006GC001478
Sadekov, A. Y., Bush, F., Kerr, J., Ganeshram, R., and Elderfield, H. (2014). Mg/Ca Composition of Benthic Foraminifera Miliolacea as a New Tool of Paleoceanography. Paleoceanography 29 (10), 990–1001. doi:10.1002/2014PA002654
Sadekov, A. Y., Eggins, S. M., and De Deckker, P. (2005). Characterization of Mg/Ca Distributions in Planktonic Foraminifera Species by Electron Microprobe Mapping. Geochem. Geophys. Geosyst. 6 (12), Q12P06. doi:10.1029/2005GC000973
Saulnier, S., Rollion-Bard, C., Vigier, N., and Chaussidon, M. (2012). Mg Isotope Fractionation during Calcite Precipitation: An Experimental Study. Geochimica et Cosmochimica Acta 91, 75–91. doi:10.1016/j.gca.2012.05.024
Segev, E., and Erez, J. (2006). Effect of Mg/Ca Ratio in Seawater on Shell Composition in Shallow Benthic Foraminifera. Geochem. Geophys. Geosyst. 7 (2), Q02P09. doi:10.1029/2005GC000969
Spero, H. J., Eggins, S. M., Russell, A. D., Vetter, L., Kilburn, M. R., and Hönisch, B. (2015). Timing and Mechanism for Intratest Mg/Ca Variability in a Living Planktic Foraminifer. Earth Planet. Sci. Lett. 409, 32–42. doi:10.1016/j.epsl.2014.10.030
Steinhardt, J., de Nooijer, L. J., Brummer, G.--J.--A., and Reichart, G.-J. (2015). Profiling Planktonic Foraminiferal Crust Formation. Geochemistry, Geophys. Geosystems, 16(7):2409–2430. doi:10.1002/2015GC005752.Received
Strelow, F. W. E. (1960). An Ion Exchange Selectivity Scale of Cations Based on Equilibrium Distribution Coefficients. Anal. Chem. 32 (9), 1185–1188. doi:10.1021/ac60165a042
Strelow, F. W. E., Rethemeyer, R., and Bothma, C. J. C. (1965). Ion Exchange Selectivity Scales for Cations in Nitric Acid and Sulfuric Acid Media with a Sulfonated Polystyrene Resin. Anal. Chem. 37 (1), 106–111. doi:10.1021/ac60220a027
Toyofuku, T., Suzuki, M., Suga, H., Sakai, S., Suzuki, A., Ishikawa, T., et al. (2011). Mg/Ca and δ18O in the Brackish Shallow-Water Benthic Foraminifer Ammonia 'beccarii'. Mar. Micropaleontol. 78 (3–4), 113–120. doi:10.1016/j.marmicro.2010.11.003
Ullmann, C. V. (2016). The Effect of Precipitation Rate on Mg/Ca and Sr/Ca Ratios in Biogenic Calcite as Observed in a Belemnite Rostrum. Biogeosciences Discuss. (8), 1–14. doi:10.5194/bg-2016-340
Urey, H. C., Lowenstam, H. A., Epstein, S., and McKinney, C. R. (1951). Measurement of Paleotemperatures and Temperatures and the Southeastern United States, Bull. Geol. Soc. Am. 62. 399–416. doi:10.1130/0016-7606(1951)62[399:MOPATO]2.0.CO;2
van Dijk, I., Barras, C., de Nooijer, L. J., Mouret, A., Geerken, E., Oron, S., et al. (2019b). Coupled Ca and Inorganic Carbon Uptake Suggested by Magnesium and Sulfur Incorporation in Foraminiferal Calcite. Biogeosciences 16 (10), 2115–2130. doi:10.5194/bg-16-2115-2019
van Dijk, I., Mouret, A., Cotte, M., Le Houedec, S., Oron, S., Reichart, G.-J., et al. (2019a). Chemical Heterogeneity of Mg, Mn, Na, S, and Sr in Benthic Foraminiferal Calcite. Front. Earth Sci. 7 (11), 1–23. doi:10.3389/feart.2019.00281
Vogl, J., Rosner, M., Kasemann, S. A., Kraft, R., Meixner, A., Noordmann, J., et al. (2020). Intercalibration of Mg Isotope Delta Scales and Realisation of SI Traceability for Mg Isotope Amount Ratios and Isotope Delta Values. Geostand Geoanal Res. 44 (3), 439–457. doi:10.1111/ggr.12327
Wilckens, F. K., Reeves, E. P., Bach, W., Seewald, J. S., and Kasemann, S. A. (2019). Application of B, Mg, Li, and Sr Isotopes in Acid‐Sulfate Vent Fluids and Volcanic Rocks as Tracers for Fluid‐Rock Interaction in Back‐Arc Hydrothermal Systems. Geochem. Geophys. Geosyst. 20 (12), 5849–5866. doi:10.1029/2019GC008694
Wit, J. C., De Nooijer, L. J., Barras, C., Jorissen, F. J., and Reichart, G. J. (2012). A Reappraisal of the Vital Effect in Cultured Benthic Foraminifer Bulimina Marginata on Mg/Ca Values: Assessing Temperature Uncertainty Relationships. Biogeosciences 9 (9), 3693–3704. doi:10.5194/bg-9-3693-2012
Wit, J. C., de Nooijer, L. J., Wolthers, M., and Reichart, G. J. (2013). A Novel Salinity Proxy Based on Na Incorporation into Foraminiferal Calcite. Biogeosciences 10 (10), 6375–6387. doi:10.5194/bg-10-6375-2013
Wombacher, F., Eisenhauer, A., Böhm, F., Gussone, N., Regenberg, M., Dullo, W.-C., et al. (2011). Magnesium Stable Isotope Fractionation in Marine Biogenic Calcite and Aragonite. Geochimica et Cosmochimica Acta 75 (19), 5797–5818. doi:10.1016/j.gca.2011.07.017
Xiang, F., Cukier, R. I., and Bu, Y. (2007). Ca2+ Selectivity of the Sarcoplasmic Reticulum Ca2+-ATPase at the Enzyme-Water Interface and in the Ca2+ Entrance Channel. J. Phys. Chem. B 111 (42), 12282–12293. doi:10.1021/jp073883q
Yoshimura, T., Tanimizu, M., Inoue, M., Suzuki, A., Iwasaki, N., and Kawahata, H. (2011). Mg Isotope Fractionation in Biogenic Carbonates of Deep-Sea Coral, Benthic Foraminifera, and Hermatypic Coral. Anal. Bioanal. Chem. 401 (9), 2755–2769. doi:10.1007/s00216-011-5264-0
Young, E. D., and Galy, A. (2004). The Isotope Geochemistry and Cosmochemistry of Magnesium. Rev. Mineralogy Geochem. 55, 197–230. doi:10.2138/gsrmg.55.1.197
Keywords: foraminifera, biomineralization, magnesium isotopes, Mg/Ca, paleothermometer
Citation: Dämmer LK, van Dijk I, de Nooijer L, van der Wagt B, Wilckens FK, Zoetemelk B and Reichart G-J (2021) Temperature Impact on Magnesium Isotope Fractionation in Cultured Foraminifera. Front. Earth Sci. 9:642256. doi: 10.3389/feart.2021.642256
Received: 15 December 2020; Accepted: 19 May 2021;
Published: 01 June 2021.
Edited by:
Guillaume Paris, Centre de recherches pétrographiques et géochimiques (CRPG), FranceReviewed by:
Nathalie Vigier, Laboratoire d’océanographie de Villefranche (LOV), FranceClaire Rollion-Bard, Institut de Physique du Globe de Paris (IPGP), France
Copyright © 2021 Dämmer, van Dijk, de Nooijer, van der Wagt, Wilckens, Zoetemelk and Reichart. This is an open-access article distributed under the terms of the Creative Commons Attribution License (CC BY). The use, distribution or reproduction in other forums is permitted, provided the original author(s) and the copyright owner(s) are credited and that the original publication in this journal is cited, in accordance with accepted academic practice. No use, distribution or reproduction is permitted which does not comply with these terms.
*Correspondence: Linda K. Dämmer, TGluZGEuRGFlbW1lckBuaW96Lm5s