- Cecil H. and Ida M. Green Institute of Geophysics and Planetary Physics, Scripps Institution of Oceanography, UC San Diego, La Jolla, CA, United States
It is generally thought that high noise levels in the oceans inhibit the observation of long-period earthquake signals such as Earth’s normal modes on ocean bottom seismometers (OBSs). Here, we document the observation of Earth’s gravest modes at periods longer than 500 s (or frequencies below 2 mHz). We start with our own 2005–2007 Plume-Lithosphere-Undersea-Mantle Experiment (PLUME) near Hawaii that deployed a large number of broadband OBSs for the first time. We collected high-quality normal mode spectra for the great November 15, 2006 Kuril Islands earthquake on multiple OBSs. The random deployment of instruments from different OBS groups allows a direct comparison between different broadband seismometers. For this event, mode
1 Introduction
Ocean bottom seismometers (OBSs) that record seismic signals on the ocean floor after a free-fall deployment are subject to tilt noise from deep-ocean currents, and there is ample evidence that burial can alleviate the noise problem (e.g. Collins et al., 2001). However, burial of an OBS is time consuming and usually requires remotely operated vehicles (e.g. Dziewonski et al., 1991; Montagner et al., 1994; Romanowicz et al., 2006). Burial is therefore cost-prohibitive when conducting campaign-style deployments of multiple OBSs, as opposed to installing a single permanent ocean floor observatory. Instrument tilt affects the horizontal seismometer components particularly strongly. This study therefore concentrates on observations on the less-affected vertical components.
It is generally thought that the noise from ocean infragravity waves inhibits meaningful observations of seismic signal at periods longer than 80 s or so. Yet, some island stations of the Global Seismographic Network (GSN) in the open ocean, including station KIP, Kipapa in Hawaii (operated jointly by the US Geological Survey and the French GEOSCOPE), exhibit perhaps surprisingly high signal levels, even at extremely low frequencies. The question then becomes whether at least some deep-ocean environments far away from long coastlines allow the observation of ultra-low frequency seismic signal as well.
The deployment of broadband ocean bottom seismometers (OBSs) for the Hawaiian Plume Lithosphere Undersea Mantle Experiment (PLUME) in 2005–2007 (Figure 1) provided one of the first opportunities to observe Earth’s normal modes on OBSs to unprecedented signal levels (Laske et al., 2007), to frequencies below 1 mHz. The collocation of temporary and permanent seismic stations on the Hawaiian Islands provides a unique opportunity to assess and quantify the quality of these observations. Subsequent analyses of other OBS deployments in the Pacific ocean involving instruments of the U.S. OBS Instrument Pool (OBSIP) provide additional high-quality normal-mode spectra.
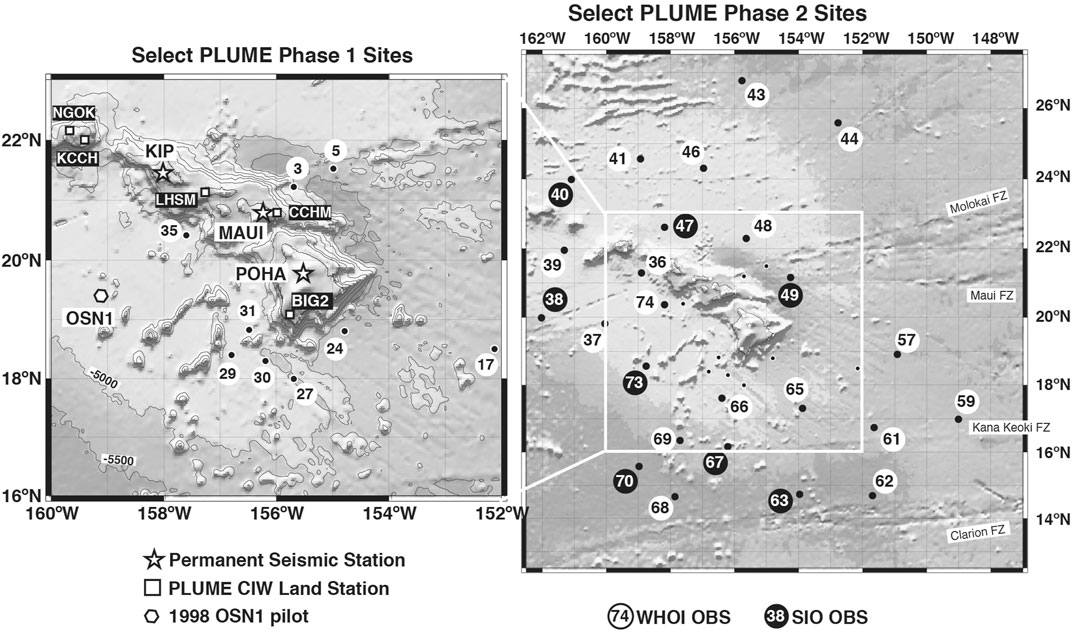
FIGURE 1. Site locations of the two deployment phases of the Hawaiian PLUME project. Phase 1 operated from January 2005 through January 2006 and phase 2 from April 2006 through June 2007. Only the sites discussed in this paper are shown. See Laske et al. (2009) for complete station and deployment history. The OSN1 label marks the location of the 1998 OSN pilot deployment (Dziewonski et al., 1991). Also shown are GSN observatory stations: KIP (Kipapa, Oahu) is jointly operated by GEOSCOPE and the U.S. Geological Survey (USGS). The primary instrument is a Wielandt-Streckeisen STS-1. The primary sensor at POHA (Pohakuloa, Hawaii), operated by the USGS, is a Teledyne-Geotech KS54000 borehole seismometer. MAUI was operated by GEOFON and featured a Wielandt-Streckeisen STS-2. The primary sensor at USGS-run MIDW (Midway Island, 28.22°N, 177.37°W, not shown), is a STS-2.
Such spectra are typically observed on broadband seismometers that have a corner period of 120 s or longer. But some spectra recorded on a Nanometrics Trillium T-40 (corner period 40 s) for the NoMelt experiment to the far south of Hawaii (Lin et al., 2016) and the ALBACORE experiment off-shore southern California (Reeves et al., 2015) also are of astonishing quality. Earth’s normal modes were never the initial target of any OBS deployment, nor was any other ultra-low-frequency signal. However, given the high costs of an OBS campaign, the fact that data are openly available to future investigators not involved in the campaign, and the fact that seismology is evolving to investigate ever-new signals, this paper makes the case that the investment in a high-quality seismic sensor may be a wise one, even for a free-fall OBS.
In the following sections, we first introduce general instrumentation requirements for observing Earth’s normal modes. We then elaborate on our PLUME deployments near Hawaii and place it in context with the previous OSN pilot experiment (Dziewonski et al., 1991). A detailed comparison between our OBS observations on one hand but also in the context of land installations on the other documents the consistently high quality of ultra-low frequency normal mode spectra, particularly those on Nanometrics Trillium T-240 seismometers. A note of caution hereby addresses the contamination by low-frequency transients during earlier deployments of Scripps Institution of Oceanography (SIO) instruments. We also discuss spectra collected on more recent deployments such as the NoMelt experiment (Lin et al., 2016), the ALBACORE deployment (Reeves et al., 2015) and a short test deployment off-shore southern California (Berger et al., 2016). All of these used SIO instruments but do not have the ‘transients problem’.
2 Requirements to Observe Earth’s Normal Modes: Instrumentation and Installation
2.1 Instrumentation
Of all seismic data, Earth’s free oscillations (normal modes) probably pose the highest demands on seismometry. To be recorded with high fidelity, noise levels in a seismic record have to stay consistently low for several days, sometimes weeks, without interruption or the interference from other seismic events. Normal mode seismometry therefore relies on the records of observatory instruments such as those of the GSN, GEOSCOPE (the French global network) or GEOFON (the German global network). The instruments of these permanent installations are typically located in a sheltered environment such as a vault, an abandoned mine or a borehole. A very-broadband sensor, such as a Wielandt-Streckeisen STS-1 vault seismometer or a Teledyne-Geotech KS54000 borehole seismometer, is needed to record Earth’s normal modes at frequencies below 1 mHz with high fidelity (Figure 2). The broadband Wielandt-Streckeisen STS-2 and the Güralp CMT-3T seismometers that are often used in temporary deployments or regional seismic networks are typically not considered to be in this category. However, the GEOFON and German Regional Seismic Network (GRSN) network operators achieved a remarkable noise reduction on their STS-2 installations by thermally insulating the sensor with a thermal blanket inside an aluminum bell jar (Hanka, 2000). This noise reduction consistently allows the observation of low-frequency normal modes after large earthquakes down to mode
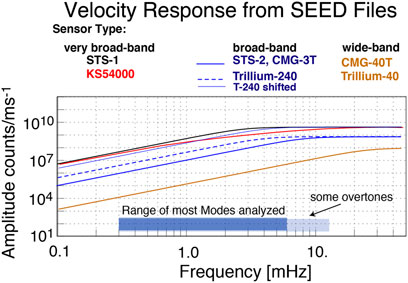
FIGURE 2. Long-period protion of the velocity response of sensors used in broadband seismology. The Wielandt Streckeisen STS-1 vault seismometer (corner period 360 s) and the Teledyne-Geotech KS54000 borehole seismometer are considered very broadband sensors, while the STS-2 and the Güralp CMG-3T (corner period 120 s) are broadband sensors. The Nanometrics Trillium T-240 (corner period 240 s) falls in between. Sensors with a corner period of 40 s (e.g. the Trillium T-40 or the CMG-40T) are wideband sensors (often mislabelled as broadband). For better comparison of the low-frequency roll-off of the Trillium 240 with that of the STS-1, it is also plotted with a thin blue line and shifted to match the STS-1 response at 10 mHz.
Ocean islands are usually thought of as being noisy sites for the GSN. In the microseism band between 15 and 5 s, noise levels can easily be 10–20 dB higher than at stations in the interiors of continents. On the other hand, and somewhat curiously, several Pacific island sites are some of the world’s quietest to record vertical ground motion in the free oscillation band at periods longer than 200 s (frequencies below 5 mHz). This includes station KIP (Kipapa, on Oahu; Hawaii) but also GEOSCOPE stationPPT (Pamatai, Papete; Tahiti). Supplementary Figure S1 shows some examples of noise curves published in the Federation of Digital Seismic Network (FDSN) station book. Station KIP compares favorably to remote installations in the interiors of continents even for the horizontal components. In this paper, we concentrate our discussion on vertical components, for two reasons. The vertical components are usually the quieter components in general. Secondly, the normal mode spectra of vertical components are easier to assess because of the general absence of toroidal modes as they have only horizontal motion, and so spectral peaks are farther apart on the vertical component than on the horizontal components that record both spheroidal and toroidal modes.
2.2 Benchmark Earthquake–The 1998 Balleny Island Earthquake at Ocean Seismic Network1
On the ocean floor, long-period noise levels are reported to be considerably higher than on land as a consequence of exposure to wind-generated infragravity waves (Webb, 1998). It has therefore been expected that free oscillations can only be observed on buried sensors. Even then, burial does not guarantee success. For example, the buried and cabled ocean bottom station H2O, halfway between Hawaii and the coast of Oregon was operational between 1999 and 2003 and was witness to several large earthquakes. But we did not obtain any convincing and consistent normal mode spectrum. The exact sensor type was a subject of long debate but may not have been a broadband sensor after all.
Just before H2O went online, the Ocean Seismic Network (OSN) initiative deployed a KS54000 very-broadband borehole sensor at ODP site 843B south of Oahu, Hawaii, about 250 m below the seafloor (Dziewonski et al., 1991; Vernon et al., 1998). During this roughly 4-months long pilot deployment, OSN1, the great
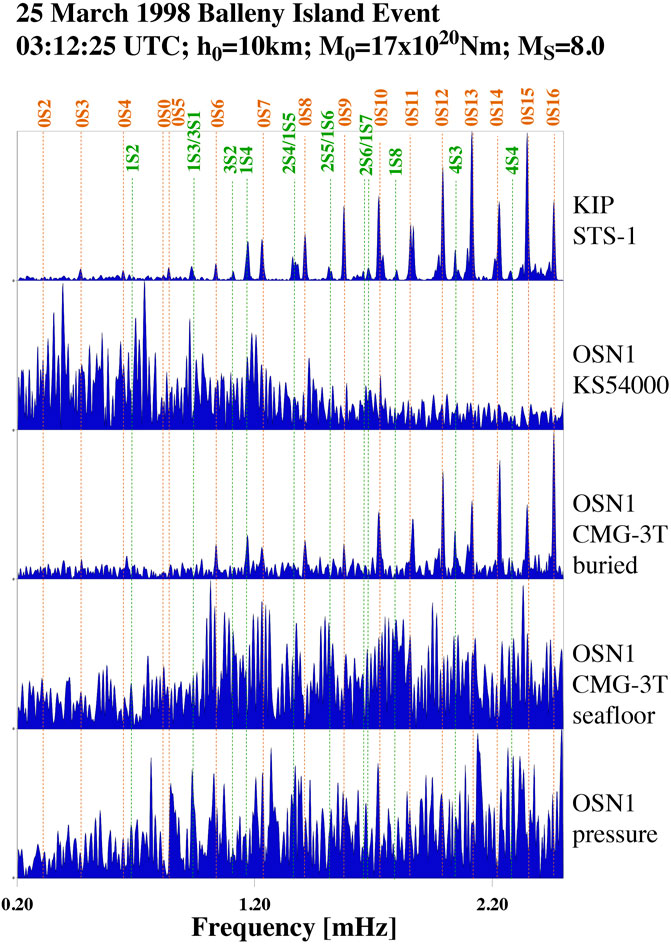
FIGURE 3. Raw vertical-component spectra for the March 25, 1998 Balleny Island earthquake of 50-hour long segments, starting 1.5 h after the source time. Hanning tapers are applied. Shown are spectra for GSN station KIP and the ocean bottom sensors deployed at OSN1(Figure 1). Comments below the station name denote the seismic sensor. At OSN1, pressure was recorded on a Cox-Webb differential pressure gauge (DPG Cox et al., 1984). In the frequency range shown here, corrections for respective instrument responses (Figure 2) change the relative shape of the spectra only marginally, but modes and background noise at frequencies below 1 mHz would be enhanced. The spectra are self-normalized for optimal display. Orange lines mark fundamental mode frequencies for model PREM(Dziewonski and Anderson, 1981), while green lines mark overtones.
To keep the data processing consistent between deployments and between earthquakes, we perform minimal data editing which may include the interactive removal of single-point spikes or similar. We apply bandpass convolution filters with a 40-dB lowpass roll-off between 40 and 50 mHz and a gently decaying highpass with a roll-off of 20 dB between 0 and 0.5 mHz to suppress tidal signals. A Hanning taper is applied before computing the spectra. But we do not correct for the instrument response, nor do we perform any noise-optimization measures such as the correction for tilt and/or pressure (Webb and Crawford, 1999; Crawford and Webb, 2000; Bell et al., 2015).
At stations on land, normal modes can be observed very clearly down to mode
At OSN1, the spectrum for the KS54000 fails to show Earth’s normal modes. Unfortunately, long-period convection of fluids in the borehole caused long-period noise (Vernon et al., 1998) to levels too high for standard normal-mode observations that involve time series lengths of several days. For extremely short windows (e.g. 20 h), some isolated modes such as
Events of the size of the Balleny Island earthquake occur perhaps every other year. The last previous event with a similar moment was the February 17, 1996 Irian Jaya earthquake (
3 The Hawaiian Plume-Lithosphere-Undersea-Mantle Experiment Project
In 2005, we launched a large broadband OBS network to investigate the fine-scale seismic structure of the crust and mantle beneath Hawaii (Figure 1). The PLUME OBS network included 73 sites that were occupied in two phases by 74 instruments (Laske et al., 2009). These instruments were provided by the two OBSIP institutional operators at the time: SIO and Woods Hole Oceanographic Institution (WHOI). The Carnegie Institution of Washington (CIW) operated ten temporary land stations for the entire duration of the field campaign. An inner OBS network of 35 sites recorded continuously from January 2005 through January 2006 (phase 1). An outer OBS network of 38 sites recorded from April 2006 through June 2007 (phase 2). During both deployment phases, the WHOI instruments were equipped with Güralp CMG-3T sensors. PLUME was the first experiment to deploy these OBSs in large numbers. The SIO instruments featured a Nanometrics Trillium T-40 wideband sensor during phase 1 and a Trillium T-240 broadband sensor during phase 2. All instruments included a Cox-Webb DPG (Cox et al., 1984). Several instruments were lost, some instruments did not record useful seismic signal, and some sites were excluded for other reasons (e.g., some phase 1 SIO OBSs recorded only on horizontal components). Figure 1 shows only the sites discussed in this paper.
During the two PLUME OBS deployments, 11 great earthquakes with scalar seismic moments
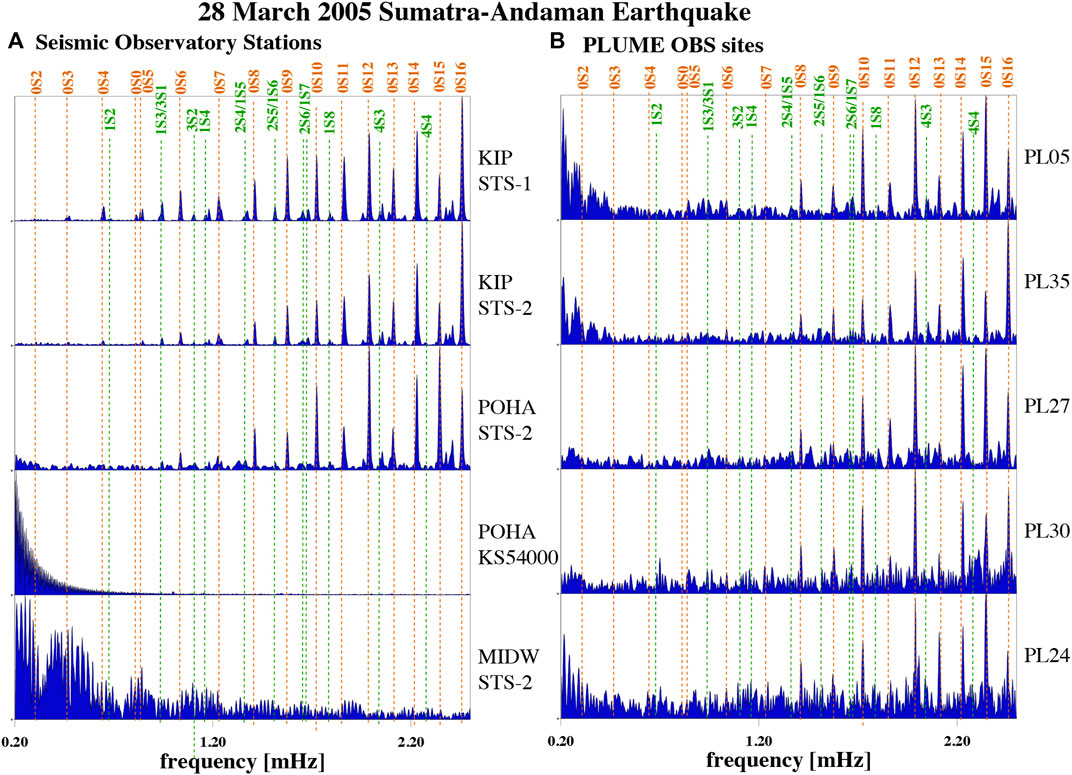
FIGURE 4. Normalized raw vertical-component spectra for the March 28, 2005 Sumatra-Andaman earthquake of 50-hour long segments, starting 1.5 h after the event time. (A) Spectra at stations of permanent observatory stations KIP, POHA and MIDW. The sensor type is listed below the stations name. Stations KIP and POHA featured two sensors, where the secondary sensor (location code 10) was a STS-2 broadband sensor. (B) Spectra at five OBS sites with the best signal-to-noise ratio. The sensor was a Güralp CMG-3T sensor. See Supplementary Table S1 in the supplement for earthquake parameters.
During the phase 2 deployment, four of the recorded events were very large, with scalar seismic moments greater than
3.1 The March 28, 2005 Sumatra Earthquake
The March 28, 2005 Sumatra earthquake was an aftershock of the devastating, tsunami-genic December 26, 2004 Sumatra-Andaman earthquake. With a scalar seismic moment
The quality of spectra recorded on the PLUME OBSs varies greatly, but mode
3.2 The June 13, 2005 Tarapaca, Northern Chile Earthquake
Events as large as the March 28, 2005 Sumatra event occur perhaps less than once a decade. High-quality spectra for this event therefore should be expected, and a favorable comparison with the 1998 Balleny Island may not be representative of the high quality of spectra collected for the PLUME project. We therefore also inspect smaller events. Figure 5 shows the spectra of the
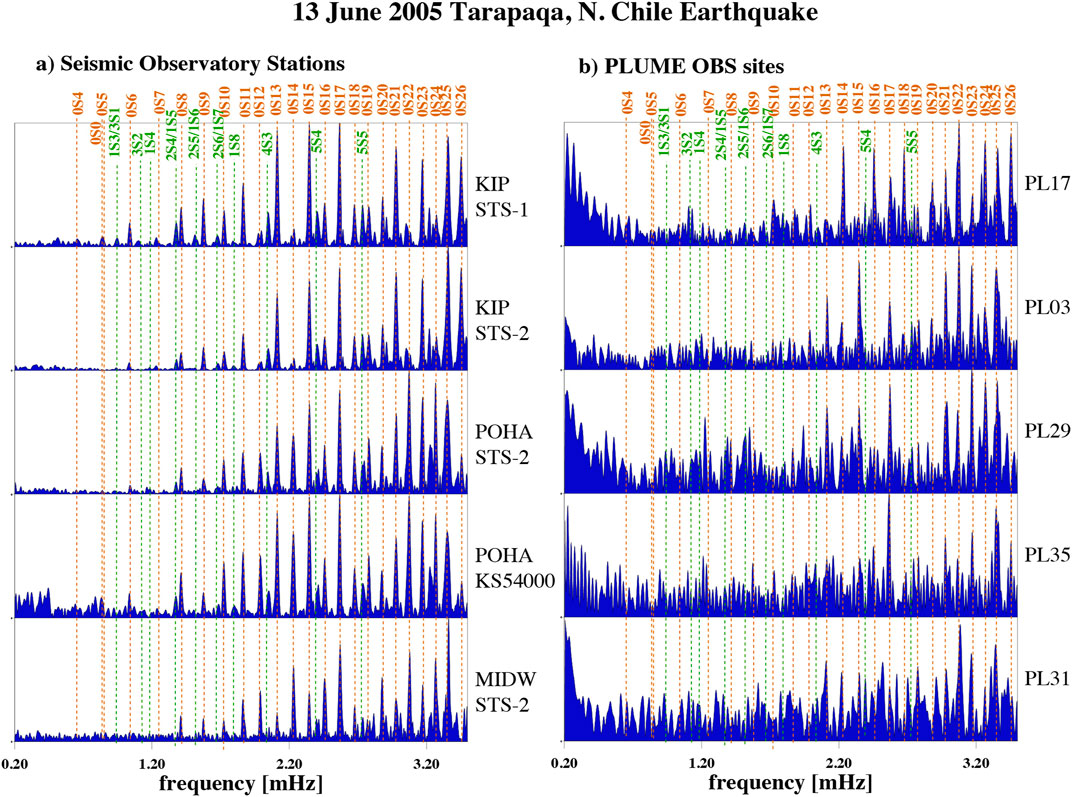
FIGURE 5. Normalized raw vertical-component spectra for the June 13, 2005 Tarapaqa, Northern Chile earthquake of 25-hour long segments, starting 1.5 h after the event time. (A) Spectra at stations of the GSN; (B) Spectra at five OBS sites with the best signal-to-noise ratio. See Supplementary Table S1 in the supplement for earthquake parameters. This event was more than three times smaller than the 1998 Balleny Island earthquake (see Figure 3). Yet, we are able to observe normal modes on unburied OBSs.
3.3 The November 15, 2006 Kuril Islands Earthquake
During PLUME phase 2, we captured four very large earthquakes with scalar seismic moment
With a scalar seismic moment
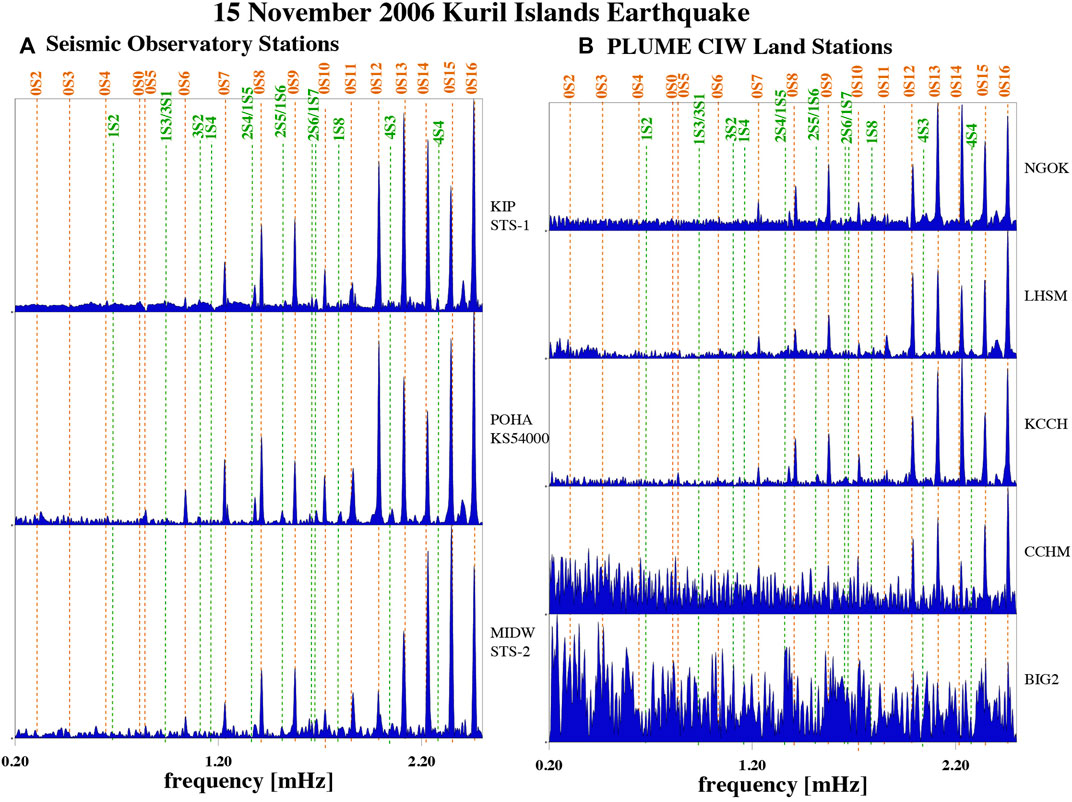
FIGURE 6. Normalized raw vertical-component spectra for the November 15, 2006 Kuril Islands earthquake of 50-hour long segments, starting 1.5 h after the event time. (A) Spectra at stations of the GSN. See Supplementary Table S1 in the supplement for earthquake parameters. (B) Spectra at the five CIW land stations still operating at the time. All CIW stations were equipped with a Wielandt-Streckeisen STS-2 seismometer.
Except for station KCCH, the noise level at the PLUME land stations is considerably higher though robust observations for mode
Taking these spectra as benchmark, we also grade the OBS spectra (Supplementary Table S2). Spectra at some of the WHOI OBSs compare quite favorably (Figure 7). At stations PL44 and PL69 (grade “A” though PL69 experienced minor data dropouts), mode
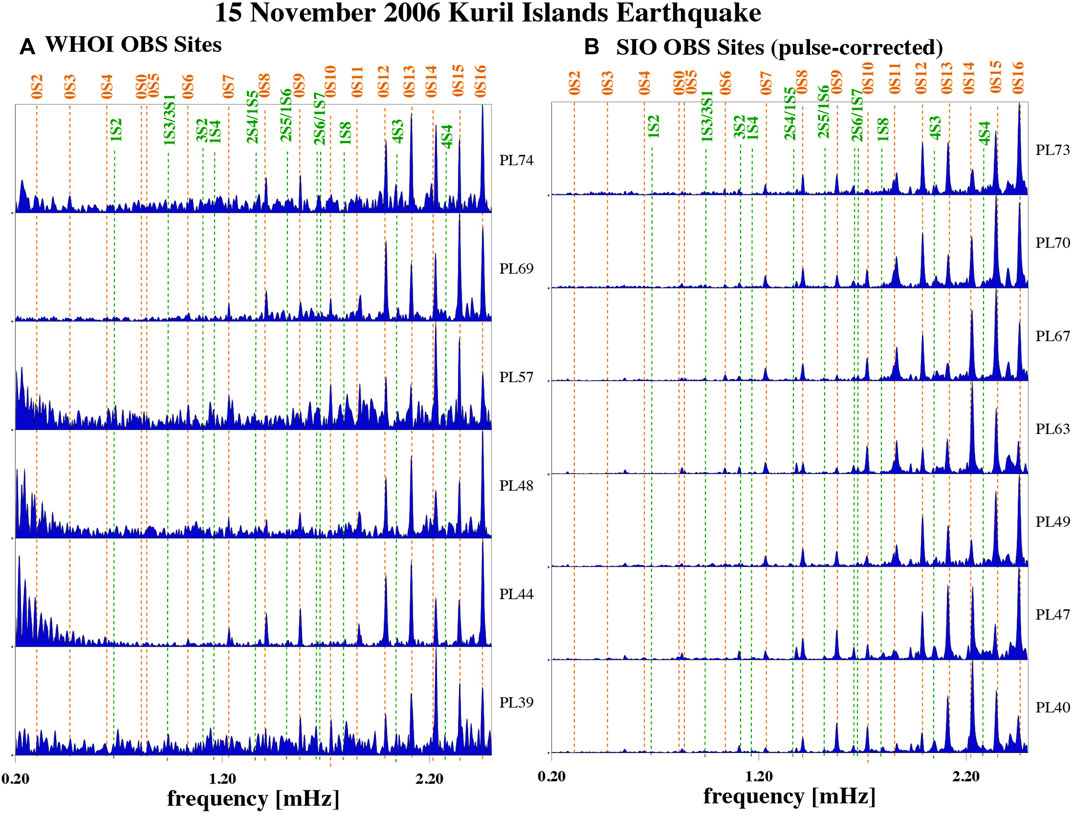
FIGURE 7. Normalized raw vertical-component spectra for the November 15, 2006 Kuril Islands earthquake of 50-hour long segments, starting 1.5 h after the event time. (A) Spectra at the six best WHOI OBS sites. The recording sensor was a CMG-3T. (B) Spectra at seven of the eight SIO OBS sites. The 8th instrument failed earlier and did not record seismic data at the time. The recording sensor was a T-240. For event details see Figure 6. The SIO spectra have been corrected for instrument-generated harmonic signals (see text for detail).
The process of assigning grades is somewhat subjective so we conduct a more quantitative assessment where we measure the peak amplitudes of the five fundamental modes
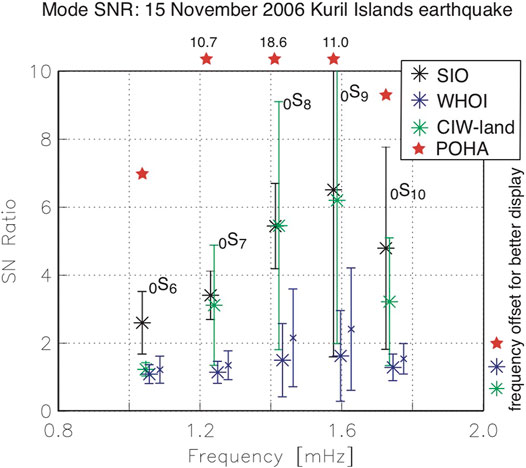
FIGURE 8. Average of signal-to-noise ratios for different instrument types and five low-frequency normal modes. Averages were determined over 7 SIO, 5 CIW-land and 17 WHOI instruments. The results are slightly offset in frequency between instrument types for better display. For reference, red stars mark the SNR measured at GSN station POHA. Blue ‘x’ mark a reanalysis of the seven best WHOI instruments.
Drawing from longtime experience with analyzing GSN data, we are aware that Güralp CMG-3T spectra can be of high quality. But the variance in the quality of CMG-3T spectra is much greater than in those for Nanometrics Trillium T-240 sensors that consistently deliver high SNR spectra. Another factor that distinguishes the two types of OBSs is the installation of the sensor: the WHOI sensor ball in which the seismometer is housed is dropped directly into the seafloor mud, while the SIO sensor ball stands on a small tripod whose footprint reaches just beyond that of the sensor ball. It has been argued that such an installation increases turbulences and may induce high-frequency noise but in-situ test deployments have so far been inconclusive (Jeff Babcock, 2016, personal communication). The tripod may well provide a more stable coupling to the ground though at this point this is highly speculative.
Unfortunately, the SIO spectra are fraught with instrument-generated low-frequency harmonic noise that remains visible even after a correction for it (see also Ringler et al., 2021). The transients were generated by the gimbal electronics in the sensor ball that awaken to check whether the seismometer needs to be relevelled. This process is a low-frequency process and is not visible in the raw data. But in lowpass filtered data, long-duration transients (up to 20 min long) become visible that have been described as ‘glitches’ (Deen et al., 2017). In the early deployments of SIO OBSs, the relevel checks were performed nearly every hour (every 3,620.56 s to be more precise) which causes the harmonics in the spectrum. Why the relevel check occurs every 3,620.56 s and not every 3,600 s is unclear. The lowest-frequency harmonic related to these checks is at 0.2762 mHz (hence the derived repeat time just mentioned). Unfortunately, higher harmonics overlap in frequency with some of Earth’s normal modes, including
The transients are different in shape between different instruments (Figure 9) but are rather reproducible on the same instrument, at least within a five-day period. The temporal distance between transients also is the same between instruments. Our somewhat ad-hoc approach to remove the transients is as follows: we determine the average ‘transient’ starting 10 h into a 110-hour long time series after the earthquake. A step in the data preprocessing is a downsampling from an original sampling rate of 31.25 Hz to a sampling interval of 9.92 s. Hence our repeat transient segment is 365 samples long (3,620.8 s). We then subtract a reconstructed time series consisting of repeats of the 365-sample transient from the entire time series. Spectra before and after the correction are juxtaposed in Supplementary Figure S2. The choice to start the averaging 10 h into the time series is the results of visually optimizing the removal of the offending harmonics at ultra-low frequencies. Starting later provides a smoother average transient in the time domain and removes more of the higher harmonics beyond 5 mHz. But this leaves more harmonics at frequencies below 2 mHz. An earlier start brings no improvement. This optimal start time of 10 h after the source time may depend on the earthquake analyzed. A mismatch between the 365-sample repeat (as constrained by the 9.92 s sampling interval) and the inferred 3,620.56 s repeat from the lowest-frequency harmonic produces a one-sample (12 s) mismatch 50 h into the time series. However, a correction in a 0.992 s downsampled time series yields no noticeably improvement. Since the correction for the transients still leaves considerable signal in the spectra, we would rather not include such spectra in a normal-mode analysis, for affected modes. After providing feedback to the SIO OBS operator, the schedule for the relevel checks was changed to occur less frequently, most recently to once a week (Martin Rapa, personal communication). The examples shown in the rest of this paper are from a time after this change in protocol occurred.
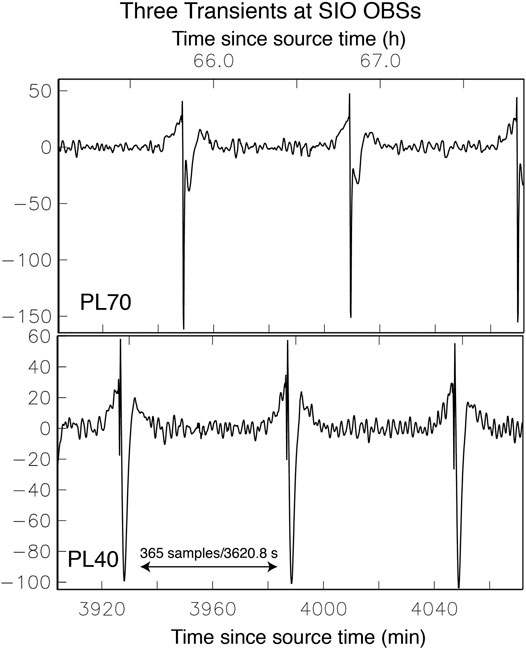
FIGURE 9. A sequence of three low-frequency transients in the bandpass filtered time series of the vertical component of SIO OBSs PL40 and PL70 many hours after the November 15, 2006 Kuril Islands earthquake. Spectral analysis indicates that the distance between pulses is 3,620.56 s, which is about 365 samples (a sample is 9.92 s, so 365 samples would be 3,620.8 s; see text for details).
4 Other Ocean Bottom Seismometer Instrument Pool Deployments in the Pacific Ocean
4.1 NoMelt
The NoMelt experiment (Lin et al., 2016; Russell et al., 2019) combined passive broadband and active-source seismic refraction/reflection surveys with the deployment of marine magnetotelluric instruments to explore 70-million year old oceanic lithosphere about 1700 km southeast of Hawaii (Figure 10). The broadband passive deployment recorded data between December 2011 and January 2013 and encompassed 20 broadband sensors as well as two additional T-40 wideband sensors. During the deployment time, six earthquakes occurred with a scalar seismic moment
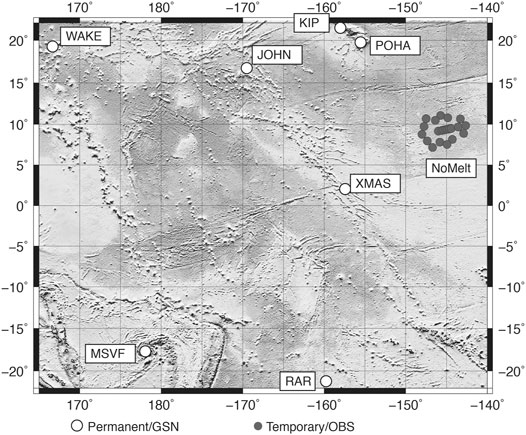
FIGURE 10. Site locations of the broadband NoMelt OBS network (Dec 2011–Jan 2013). Also shown are sites of permanent observatory stations of the GSN: KIP (Kipapa, Oahu; primary sensor: STS-1), POHA (Pohakuloa, Hawaii; KS54000), JOHN (Johnston Island; KS54000), WAKE (Wake Island; KS54000), XMAS (Kiritimati Island, Kiribati; STS-2); RAR (Rarotonga, Cook Islands (KS54000); MSVF (Monasavu, Fiji; KS54000).
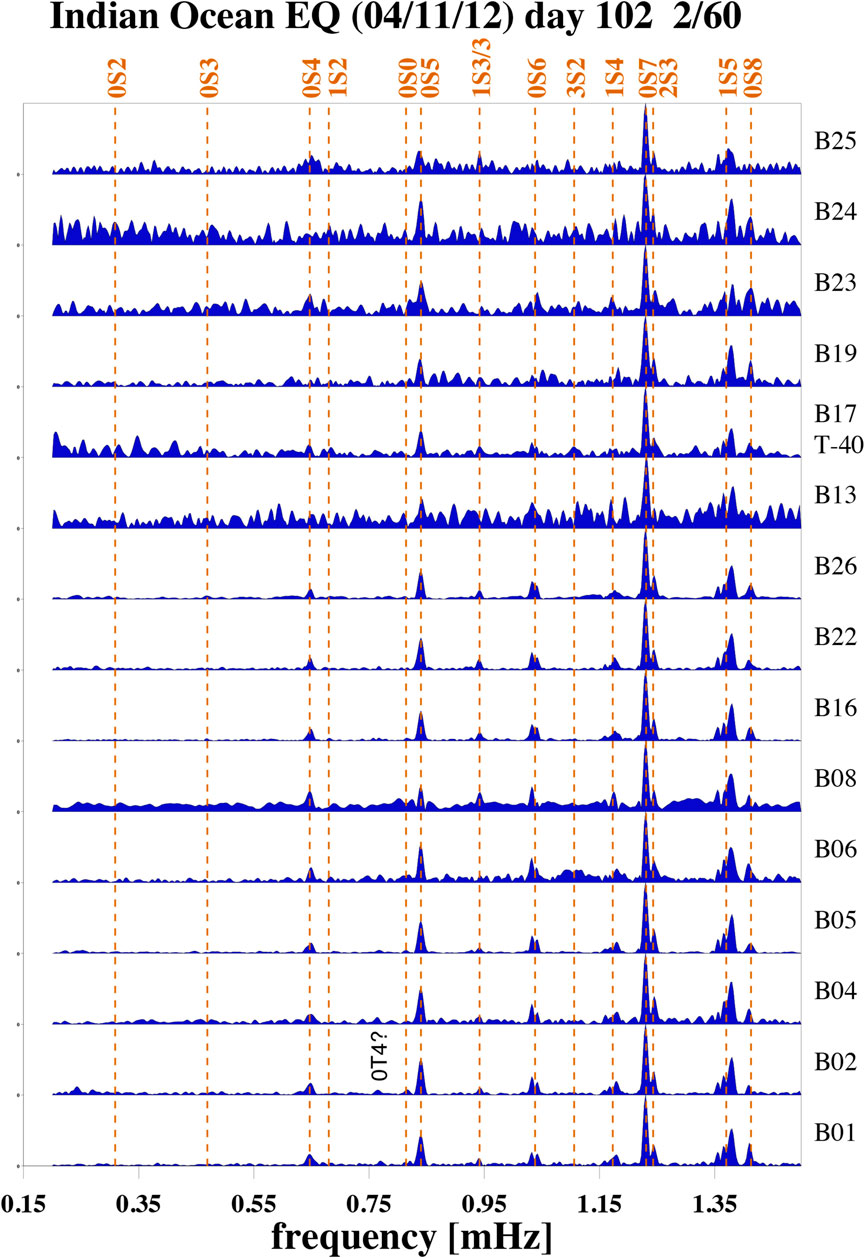
FIGURE 11. Normalized raw vertical-component spectra for the April 11, 2012 Indian Ocean earthquake of 60-hour long segments, starting 2 h after the event time. See Supplementary Table S1 in the supplement for earthquake parameters. Site B17 featured a Nanometrics Trillium T-40 wideband sensor.
A direct quantitative comparison with land installations is somewhat hampered as the closest GSN station, XMAS (Kirimati Island, Kiribati), is about 1,500 km away. At such distances, spectral amplitudes for a mode can be vastly different between stations while that of other modes are not affected as much. In a somewhat extreme case, for a station about 90° away from the source, every other fundamental mode may experience a greatly reduced amplitude (Laske and Widmer-Schnidrig, 2015). Bearing this in mind, Figure 12 displays seven representative spectra in the southwestern Pacific ocean. For all but one station (MSVF) we consider both the primary and the secondary sensors (location codes 00 and 10) and make a decision which sensor in the better one to include in this study. For stations WAKE, RAR and XMAS, we choose the secondary sensor, a Wielandt-Streckeisen STS-2. At XMAS, the record of the primary sensor was a grade F, at WAKE we see a Rayleigh wave on the primary sensor but normal mode peaks appear only at frequencies above 2 mHz and only if we take shorter records (grade E). At station RAR, the quality of the spectrum for the primary sensor was of marginally less quality than that of the secondary sensor though it would hamper analysis of mode
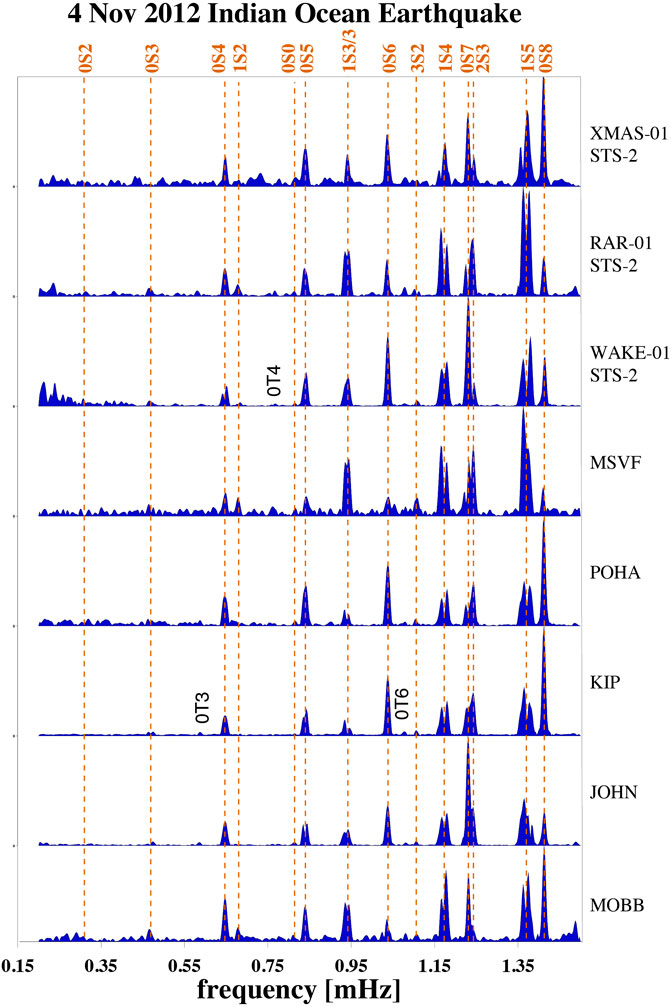
FIGURE 12. Normalized raw vertical-component spectra at GSN stations for the April 11, 2012 Indian Ocean earthquake of 60-hour long segments, starting 2 h after the event time. A code 01 after the station code means that we use the secondary sensor for analysis (sensor type below the station name). Also shown is the spectrum of seafloor observatory MOBB off-shore California (see Figure 13).
The spectra for this event are remarkable, for a number of reasons. Firstly, mode
To complement the comparison, seafloor observatory MOBB off-shore California (Romanowicz et al., 2006) (Figure 13) also recorded this event with high fidelity. This site has a broadband Güralp CMG-1T seismometer that is buried beneath at least 10 cm of sediments and is located at a water depth of 1,000 m. At this water depth, we normally expect high noise levels in the infragravity band at frequencies below about 10 mHz. Yet, the spectrum at MOBB is also of extremely high quality where mode
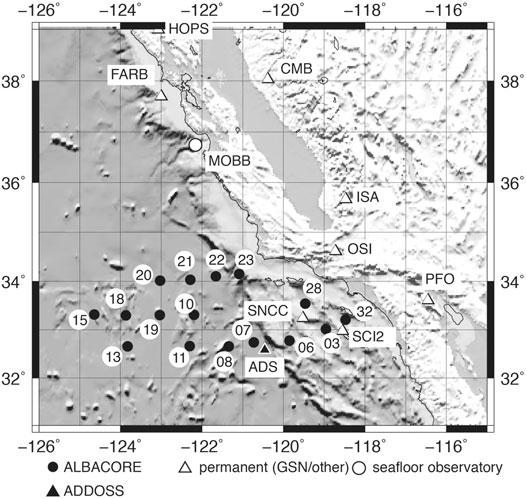
FIGURE 13. Site locations of the 16 broadband stations of the ALBACORE network (Aug 2010–Sep 2011) used in this study. Also shown are sites of permanent observatory stations of the GSN: PFO (Piñon Flat Observatory; primary sensor: STS-1), stations of the CI network (OSI, Osito Adit, STS-1; ISA, Isabella; ,STS-1; SNCC, San Nicolas Island, STS-1; SCI2, San Clemente Island, STS-2), stations of the BK network (HOPS, Hopland Field station, STS-1; CMB, Columbia College, STS-1; BKS, Berkeley, Byerly Vault, STS-1; FARB, Farallon Islands, STS-2) and seafloor observatory MOBB (Monterey Bay Broadband Ocean Botton Seismic Observatory, CMG-1T). The ADDOSS OBS as site ADS operated Dec 2013 through Mar 2014 (Berger et al., 2016). A student-led repeat deployment followed in summer 2015.
Noteworthy, a number of GSN stations but also NoMelt OBSs exhibit spectral peaks at the frequencies for toroidal modes
We also inspect all of the NoMelt DPG spectra. While it is tempting to associate individual peaks across all stations with a normal mode, none of the 22 DPG exhibits a consistent spectrum that displays at least three adjacent fundamental modes with clear SNRs, regardless of the time series length. On the other hand, such sensors allow the analysis of semi-diurnal tidal peaks (e.g. Doran et al., 2019). Since the tidal signal is much larger than that of earthquakes, we infer that noise levels in the free oscillation band are too high to observe even very large earthquakes.
4.2 ALBACORE
The passive broadband OBS deployment for the ALBACORE experiment in the California Borderland (Reeves et al., 2015) occurred from August 2010 through September 2011 and so immediately preceded the NoMelt experiment. The metadata at the IRIS DMC includes station information for 36 OBS sites. Twelve of these were equipped with short-period instruments. Three of the remaining sites were equipped with a T-40 wideband sensor, while the rest had a T-240 broadband sensor. We obtained data for 21 stations. Five of these are grade F, exhibiting only bit noise (i.e. signals of a few counts), one T-40 record was suspicious (counts were primarily single-sided). This leaves us with usable records at 16 OBS sites (see Figure 13), one of which featured a T-40. In the following, we use four letters to name the stations though the original station names consist of five (OBS?? where ‘?’ is a number). During this deployment, four large distinct earthquakes occurred. By far the largest was the March 11, 2011 Tohoku, Japan earthquake (scalar seismic moment
Accordingly, spectra collected on land stations are of the highest quality and set a rather high bar for any OBS recording (Figure 14). The spectra of the best stations clearly show the gravest mode
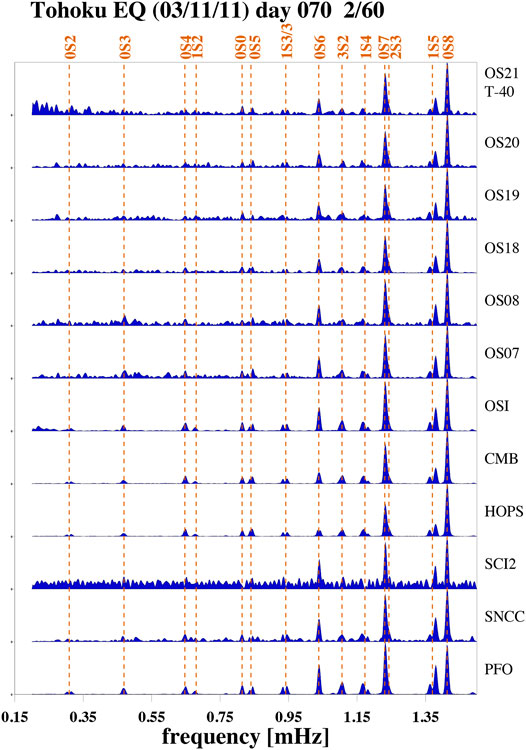
FIGURE 14. Normalized raw vertical-component spectra for the March 11, 2011 Tohoku, Japan earthquake of 60-hour long segments, starting 2 h after the event time. The bottom six spectra are from permanent land stations (see 15), while the top six spectra are for ALBACORE OBS sites. Site OS21 featured a Nanometrics Trillium T-40 wideband sensor. See online supplement for the spectra of all broadband OBSs.
Most remarkably, the sensor at site OS21 is the wideband T-40. A double-check of the raw spectra confirms a similar finding as that of site B17 for NoMelt and confirms that we are dealing with a T-40 and not a typo in the metadata. At this point, we doubt that all T-40s perform this well but as described above, the other two T-40s in this experiments did not provide usable data. One must wonder whether this was the same sensor as that at NoMelt site B17. Unfortunately, this sensor is likely no longer in the fleet as the SIO OBS group subsequently exchanged T-40 sensors for T-240s. While T-240s are expected to perform better in the free oscillation band, the loss of this particular T-40 is arguable a great loss for ocean bottom normal-mode seismology and engineering alike. It would have been nice to explore in more detail exactly what made this particular sensor so unique in its performance.
The deployment of ALBACORE OBSs in a wide range of water depths allows us to explore the influence of water depth on the quality of the free-oscillation SNR. While most of the OBSs were deployed in the deeper ocean at depths greater than 3,500 m, even the spectrum for OS23 at a water depth near 2000 m is acceptable (see Supplementary Figure S5). But a deployment at shallower depths clearly increases noise levels. To quantify this further, we measure the SNR for six modes at the six shallowest sites and compare that against the SNR at the other ten sites. A split into four shallow vs twelve deeper sites would follow the narrative above better, but we feel that the subsequently smaller standard deviations for smaller samples would not be representative. Figure 15 confirms that the shallower sites have lower SNRs for all modes than the deeper sites. The relatively low SNR for mode
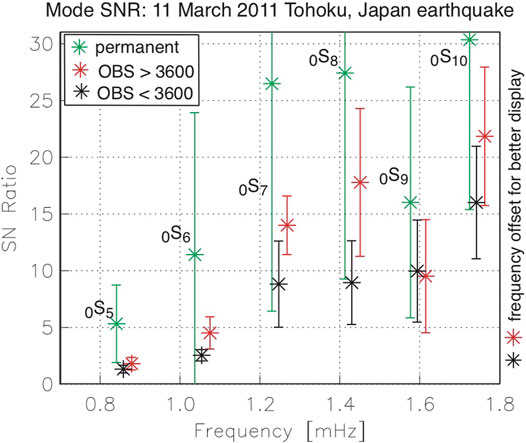
FIGURE 15. Average of signal-to-noise ratios for the 16 ALBACORE stations that had useful seismic data (15 broadband, 1 wideband). The results are slightly offset in frequency between instrument types for better display. Six grade-E or F stations were discarded and not included (5 broadband, 1 wideband). Black: 10 OBSs in deeper water (
We briefly also discuss the other large earthquakes observed at the ALBACORE deployment. With a scalar seismic moment
With a scalar seismic moment
The December 21, 2010 Izu Bonin was an earthquake with a scalar seismic moment less than
4.3 ADDOSS
Another deployment of broadband OBSs in the California Borderland was the ADDOSS (Autonomously Deployable Deep-Ocean Seismic System) experiment (Berger et al., 2016). The final of several deployments occurred from December 2013 through March 2014 on the deep-ocean side of the Patton Escarpment (site ADS3 in Figure 13) where two broadband OBSs were deployed within 1 km of each other. The primary goal of this deployment was a proof-of-concept study that a wave glider can hold station around the OBS drop location and serve as acoustic-satellite relay to transmit data from the ocean floor to a desktop computer on land in near-real time. During the three months, we recorded numerous teleseismic, regional and local earthquakes, but the scalar seismic moment of the largest earthquake remained below
We were luckier when one of the ADDOSS instruments was redeployed 18 months later as a piggy-back to a short student-led experiment near the ADS3 site. With a scalar seismic moment
5 Summary and Discussion
We showed vertical-component free oscillation spectra for data collected during a number of ocean bottom seismometer (OBS) deployments in the Pacific Ocean that occurred over the last 20 years. Broadband seismology on the ocean floor using free-fall OBSs is expected to be hampered at long periods as a result of noise contamination by ocean infragravity waves. Yet, our examples showed that some spectra collected on broadband seismic sensors in the free oscillation band can reach the quality a data analyst is used from land observatory stations such as those of the Global Seismographic Network (GSN). This includes mode observations at frequencies below 1.5 mHz which require low-noise records several days long.
The SIO OBSs that are equipped with a Nanometrics Trillium T-240 sensor were found to produce consistently high-quality spectra to very low frequencies. Records include even diurnal and semi-diurnal tidal modes (e.g. Doran et al., 2019) which helps recalibrate nominal instrument responses for the pressure sensors, typically a broadband Cox-Webb differential pressure gauge (Cox et al., 1984) where sensor sensitivities can effectively vary by a factor of two. Earlier SIO OBS records are contaminated by approximately hourly transients that hamper a normal modes analysis, at least for certain modes. A removal of these signals in post-processing is moderately successful, as we and others showed (Deen et al., 2017). The low-frequency transients are generated in the electronics to check whether the seismometer needs to be relevelled even though the sensor is not mechanically relevelled. For the SIO OBSs that were part of the national OBS Instrument Pool (OBSIP), the relevel schedule was changed shortly after our Hawaiian PLUME deployment to have less frequent relevel checks. However, the approximately hourly schedule may still be set on instruments sold by the SIO OBS group to others (e.g. Deen et al., 2017).
The T-240s have also been deployed in the Atlantic, where a recent study reported earthquake-generated free oscillation observations (Bécel et al., 2011). Our study concentrated on normal modes at ultra-low frequencies that are excited by large earthquakes. At higher frequencies, Earth’s ‘hum’ that consists primarily of fundamental modes and that is thought to be excited by ocean infragravity waves interacting on shelves (Webb, 2007) was recently observed on free-fall OBSs in the Indian ocean Deen et al. (2017).
We performed minimal data processing, and so did not correct the vertical seismometer components for pressure nor tilt noise (e.g. Webb and Crawford, 1999; Crawford and Webb, 2000; Bell et al., 2015). A correction may improve the signal-to-noise ratio, even in the normal mode band below 5 mHz. We also did not correct the records of the 1998 ocean seismic network (OSN) pilot experiment that was located within our PLUME network. Crawford et al. (2006) describe a procedure to remove much of the noise for this specific deployment. Using a barometer, noise corrections on vertical components can also be performed for land observatory stations (e.g. Zürn and Widmer, 1995), and many, if not most GSN stations are now equipped with such a sensor.
The ALBACORE deployment off-shore southern California (Reeves et al., 2015) allowed us to investigate long-period background noise as function of water depth. We found a clear separation in quality between mode spectra for deep deployments (3,600 m or deeper) and shallower deployments. A spectrum collected at 2000 m also was of acceptable quality, while spectra at shallower sites contained more low-frequency noise but still revealed modes at frequencies down to 1 mHz. The best OBS spectra for the ALBACORE and NoMelt (Lin et al., 2016) deployments contained modes down to
Our study focused on the vertical seismometer components. The horizontal seismometer components are noisier in general, and much more so in the oceans. Here, tilt noise contaminates the seismic record in a wide range of frequencies. At PLUME, we noted a semidiurnal cycle that suggests that tidal currents trigger the noise. Yet, it is possible to observe useful signals such as Love waves or shear-wave splitting observations during relatively low-noise times. Finally, while ground motion spectra are of high quality, we have yet to find a convincing pressure spectrum in which Earth’s normal modes rise above the background noise. There have been debates whether the money spent on an expensive broadband seismometer for an OBS is a wise investment since it is often contested that observations at low and ultra-low frequencies are possible. This paper documents that, given the high costs of an OBS in general, the investment in a high-quality broadband seismic sensor is well justified.
Data Availability Statement
The original contributions presented in the study are included in the article/Supplementary Material, further inquiries can be directed to the corresponding author.
Author Contributions
The author confirms being the sole contributor of this work and has approved it for publication.
Funding
National Science Foundation grants EAR11-13075 and OCE18-30959.
Conflict of Interest
The author declares that the research was conducted in the absence of any commercial or financial relationships that could be construed as a potential conflict of interest.
Acknowledgments
The author would like to thank the teams and operators of the OSN1 pilot experiment, the NoMelt team and the ALBACORE team for their efforts to conduct their experiments and ultimately deliver and share high-quality broadband seismic data. Instruments for the latter two as well as the Hawaiian PLUME experiment were provided by the NSF OBS Instrument Pool. The OBS instruments were prepared, deployed and recovered by the OBS teams at WHOI and at SIO. Network operators of land seismic observatories include the IRIS-IDA (network code II) and IRIS-USGS (network code IU) operators of the GSN, and the operators of GEOSCOPE (network code G) and GEOFON (network code GE). Stations in California are operated by the University of California Berkeley Digital Seismograph Network team (network code BK) and the Caltech/USGS Southern California Seismic Network team (network code CI). Station characteristics in the supplement were taken from the Federation of Digital Seismograph Networks (FDSN) station book. All data are available for download at the IRIS data management center (DMC). The author is indebted to SIO OBS team member Martin Rapa for many fruitful discussions. The ADDOSS follow-up cruise was funded through UC Shipfunds and led by graduate student Adrian Doran. The maps were drawn using the Generic Mapping Tools (GMT Wessel et al., 2013).
Supplementary Material
The Supplementary Material for this article can be found online at: https://www.frontiersin.org/articles/10.3389/feart.2021.679958/full#supplementary-material
References
Bécel, A., Laigle, M., Diaz, J., Montagner, J. -P., and Hirn, A. (2011). Earth’s Free Oscillations Recorded by Free-Fall OBS Ocean–Bottom Seismometers at the Lesser Antilles Subduction Zone. Geophys. Res. Lett. 38, L24305. doi:10.1029/2011GL049533
Bell, S. W., Forsyth, D. W., and Ruan, Y. (2015). Removing Noise from the Vertical Component Records of Ocean‐Bottom Seismometers: Results from Year One of the Cascadia Initiative. Bull. Seismological Soc. America 105 (1), 300–313. doi:10.1785/0120140054
Berger, J., Laske, G., Babcock, J., and Orcutt, J. (2016). An Ocean Bottom Seismic Observatory with Near Real‐time Telemetry. Earth Space Sci. 3, 68–77. doi:10.1002/2015EA000137
Collins, J. A., Vernon, F. L., Orcutt, J. A., Stephen, R. A., Peal, K. R., Wooding, F. B., et al. (2001). Broadband Seismology in the Oceans: Lessons from the Ocean Seismic Network Pilot Experiment. Geophys. Res. Lett. 28, 49–52. doi:10.1029/2000gl011638
Cox, C., Deaton, T., and Webb, S. (1984). A Deep-Sea Differential Pressure Gauge. J. Atmos. Oceanic Technol. 1, 237–246. doi:10.1175/1520-0426(1984)001<0237:adsdpg>2.0.co;2
Crawford, W. C., Stephen, R. A., and Bolmer, S. T. (2006). A Second Look at Low-Frequency marine Vertical Seismometer Data Quality at the OSN-1 Site off Hawaii for Seafloor, Buried, and Borehole Emplacements. Bull. Seismological Soc. America 96 (5), 1952–1960. doi:10.1785/0120050234
Crawford, W. C., and Webb, S. (2000). Identifying and Removing Tilt Noise from Low-Frequency. Bull. Seismological Soc. America 90 (4), 952–963. doi:10.1785/0119990121
Deen, M., Wielandt, E., Stutzmann, E., Crawford, W., Barruol, G., and Sigloch, K. (2017). First Observation of the Earth's Permanent Free Oscillations on Ocean Bottom Seismometers. Geophys. Res. Lett. 44 (), 10,988–10,996. doi:10.1002/2017GL074892
Doran, A. K., Rapa, M., Laske, G., Babcock, J., and McPeak, S. (2019). Calibration of Differential Pressure Gauges through In Situ Testing. Earth Space Sci. 6, 2663–2670. doi:10.1029/2019EA000783
Dziewonski, A. M., and Anderson, D. L. (1981). Preliminary Reference Earth Model. Phys. Earth Planet. Interiors 25, 297–356. doi:10.1016/0031-9201(81)90046-7
Dziewonski, A., Wilkens, R. H., Firth, J. V., and Science Party, Shipboard. (1991). Background and Objectives of the Ocean Seismographic Network, and Leg 136 Drilling Results. Proc. Ocean Drilling Program 136/137, 3–8.
Hanka, W. (2000). Comparison of GEOFON and GRSN Shielding. http://geofon.gfz-potsdam.de/geofon/manual/gfz-grsn-shield.html.
Laske, G., Collins, J. A., Wolfe, C. J., Solomon, S. C., Detrick, R. S., Orcutt, J. A., et al. (2009). Probing the Hawaiian Hot Spot with New Broadband Ocean Bottom Instruments. Eos Trans. AGU 90 (41), 362–363. doi:10.1029/2009eo410002
Laske, G., Orcutt, J. A., Collins, J. A., Detrick, R. S., Wolfe, C. J., Solomon, S. C., et al. (2007). Broadband Ocean Bottom Instruments Record Earth’s Free Oscillations during the Hawaiian PLUME experiment. Eos Trans. AGU 88 (52), 1. Fall Meeting suppl., abstract S23A-1107.
Laske, G., and Widmer-Schnidrig, R. (2015). “Theory and Observations: Normal Mode and Surface Wave Observations,”in. Treatise on Geophysics. Editor G. Schubert. Second Edition (Elsevier), 1, 117–167. doi:10.1016/b978-0-444-53802-4.00003-8
Lin, P.-Y. P., Gaherty, J. B., JinCollins, G. J. A., Collins, J. A., Lizarralde, D., Evans, R. L., et al. (2016). High-resolution Seismic Constraints on Flow Dynamics in the Oceanic Asthenosphere. Nature 535 (52), 538–541. doi:10.1038/nature18012
Masters, G., Laske, G., and Gilbert, F. (2000). Autoregressive Estimation of the Splitting Matrix of Free-Oscillation Multiplets. Geophys. J. Int. 141, 25–42. doi:10.1046/j.1365-246x.2000.00058.x
Masters, G., Park, J., and Gilbert, F. (1983). Observations of Coupled Spheroidal and Toroidal Modes. J. Geophys. Res. 88, 10,285–10,298. doi:10.1029/jb088ib12p10285
Montagner, J.-P., Karczewski, J.-F. o., Romanowicz, B., Bouaricha, S., Lognonne´, P., Roult, G. v., et al. (1994). The French Pilot Experiment OFM-SISMOBS: First Scientific Results on Noise Level and Event Detection. Phys. Earth Planet. Interiors 84, 321–336. doi:10.1016/0031-9201(94)90050-7
Reeves, Z., Lekić, V., Schmerr, N., Kohler, M., and Weeraratne, D. (2015). Lithospheric Structure across the California Continental Borderland from Receiver Functions. Geochem. Geophys. Geosyst. 16, 246–266. doi:10.1002/2014GC005617
Ringler, A. T., Mason, D., Storm, T., Laske, G., Templeton, M., and Anthony, R. E. (2021). Why Do My Squiggles Look Funny? A Gallery of Instrumentation Failure Modes. Seismol. Res. Lett. in review.
Romanowicz, B., Stakes, D., Dolenc, D., Neuhauser, D., McGill, P., Uhrhammer, R., et al. (2006). The Monterey Bay Broadband Ocean Bottom Seismic Observatory. Ann. Geophys. 49, 607–623. doi:10.4401/ag-3132
Russell, J. B., Gaherty, J. B., Lin, P. Y. P., Lizarralde, D., Collins, J. A., Hirth, G., et al. (2019). High‐Resolution Constraints on Pacific Upper Mantle Petrofabric Inferred from Surface‐Wave Anisotropy. J. Geophys. Res. Solid Earth 124, 631–657. doi:10.1029/2018JB016598
Vernon, F. L., Collins, J. A., Orcutt, J. A., Stephen, R. A., Peal, K., Wolfe, C. J., et al. (1998). Evaluation of Teleseismic Waveforms and Detection Thresholds from the OSN Pilot Experiment. EOS Trans. AGU 79, F650. doi:10.1121/1.425544
Webb, S. C. (1998). Broadband Seismology and Noise under the Ocean. Rev. Geophys. 36 (1), 105–142. doi:10.1029/97rg02287
Webb, S. C., and Crawford, W. C. (1999). Long Period Seafloor Seismology and Deformation under Ocean Waves. Bull. Seismol. Soc. Am. 89, 1535–1542.
Webb, S. C. (2007). The Earth's 'hum' Is Driven by Ocean Waves over the continental Shelves. Nature 445, 754–756. doi:10.1038/nature05536
Wessel, P., Smith, W. H. F., Scharroo, R., Luis, J., and Wobbe, F. (2013). Generic Mapping Tools: Improved Version Released. EOS Trans. AGU 94, 409–410. doi:10.1002/2013EO450001
Zürn, W., Laske, G., Widmer-Schnidrig, R., and Gilbert, F. (2000). Observation of Coriolis Coupled Modes below 1 mHz. Geophys. J. Int. 143, 113–118. doi:10.1046/j.1365-246x.2000.00220.x
Keywords: seismology, instrumentation, ocean bottom seismometers, broadband seismology, earth’s normal modes, earth structure
Citation: Laske G (2021) Observations of Earth’s Normal Modes on Broadband Ocean Bottom Seismometers. Front. Earth Sci. 9:679958. doi: 10.3389/feart.2021.679958
Received: 12 March 2021; Accepted: 07 June 2021;
Published: 21 June 2021.
Edited by:
Charlotte A. Rowe, Los Alamos National Laboratory (DOE), United StatesCopyright © 2021 Laske. This is an open-access article distributed under the terms of the Creative Commons Attribution License (CC BY). The use, distribution or reproduction in other forums is permitted, provided the original author(s) and the copyright owner(s) are credited and that the original publication in this journal is cited, in accordance with accepted academic practice. No use, distribution or reproduction is permitted which does not comply with these terms.
*Correspondence: Gabi Laske, Z2xhc2tlQHVjc2QuZWR1