- 1Shandong Provincial Key Laboratory of Deep Oil and Gas, Qingdao, China
- 2School of Geosciences, China University of Petroleum (East China), Qingdao, China
- 3Energy & Geoscience Institute, University of Utah, Salt Lake City, UT, United States
- 4State Key Laboratory of Petroleum Resources and Prospecting, China University of Petroleum, Beijing, China
- 5Key Laboratory of Tectonics and Petroleum Resources, Ministry of Education, China University of Geosciences, Wuhan, China
- 6Energy Department of CNPC Greatwall Drilling Company, Beijing, China
- 7School of Geosciences and Info-Physics, Central South University, Changsha, China
The presence and content of water will certainly affect the gas adsorption capacity of shale and the evaluation of shale gas content. In order to reasonably evaluate the gas adsorption capacity of shale under actual reservoir conditions, the effect of water on methane adsorption capacity needs to be investigated. Taking the Da’anzhai Member of the Lower Jurassic Ziliujing Formation in the northeastern Sichuan Basin, China as an example, this study attempts to reveal the effect of pre-adsorbed water on methane adsorption capacity in shale-gas systems by conducting methane adsorption experiments in two sequences, firstly at different temperatures under dry condition and secondly at different relative humidity levels under the same temperature. The results show that temperature and relative humidity (i.e., water saturation) are the main factors affecting the methane adsorption capacity of shale for a single sample. The key findings of this study include: 1) Methane adsorption capacity of shale first increases then decreases with depth, reaching a peak at about 1,600–2,400 m. 2) Lower relative humidity correlates to greater maximum methane adsorption capacity and greater depth to reach the maximum methane adsorption capacity. 3) 20% increase of relative humidity results in roughly 10% reduction of maximum methane adsorption capacity. As a conclusion, methane adsorption capacity of shale is predominately affected by water saturation, pore type and pore size of shale. This study could provide a theoretical basis for the establishment of a reasonable evaluation method for shale adsorbed gas content.
Introduction
Shales have large accumulations of hydrocarbons and are not merely regarded nowadays as source rocks and seals anymore (Krooss et al., 1995; Hill et al., 2007; Tian et al., 2013; Chen et al., 2017a; Gao et al., 2020). Shale gas is known for its self-generation and self-storage characteristics and large-scale continuous accumulations (Jarvie et al., 2007; Zou et al., 2017; Xu et al., 2020). Appraisal of recoverable shale gas reserves is the very basis for evaluating the development potential of a shale play and thereafter formulating a suitable gas field development plan (Li et al., 2016). In shale reservoirs, natural gas is usually stored in three different types of geological environment which include free gas in pores and fractures, adsorbed gas in organic matters and clay minerals and dissolved gas in residual oil and water (Curtis, 2002; Zhang et al., 2012). As nano-scale pores are most developed in shales (Zou et al., 2017; Chen et al., 2019a; Liu et al., 2020), the adsorbed gas content generally accounts for 20–85% of the total gas content (Curtis, 2002; Chen et al., 2019b; Qiao et al., 2020). The research of gas adsorption capacity forms the foundation for effective and efficient development of shale gas because it is paramount to appraising gas content in-situ as well as pinpointing the target areas (Gai et al., 2020). Shales have extremely low permeability, so more than 90% of shale gas wells need to rely on hydraulic fracturing and other stimulation measures to connect natural fractures for improving the conductivity of the reservoir in order to promote the economic development of shale gas (Zou et al., 2017; Gao et al., 2021; Liu et al., 2021). The average water saturation of shale gas reservoirs in Barnett, Marcellus and Woodford Formations in the US ranges from 15 to 35% (Ambrose et al., 2012), compared to 10–95% for the marine shale plays found in South China (Liu and Wang, 2013; Fang et al., 2014). Fang et al. (2014) explained the root cause of the ultra-low water saturation of marine shales in South China, pointing out that certain shale reservoirs have natural occurrence of water. Therefore, it is of great significance to study the methane adsorption capacity of water-bearing shales.
At present, the evaluation of adsorbed gas content is mainly based on methane adsorption experiments. Most researchers used dry shale samples for methane adsorption experiments and did not account for the effect of water (Chareonsuppanimit et al., 2012; Gasparik et al., 2012; Ji et al., 2015; Tian et al., 2016; Sander et al., 2018; Song et al., 2018; Shang et al., 2020). For example, Gasparik et al. (2013) studied the high-pressure methane sorption isotherms of black shales from Netherlands and found that clay minerals can contribute significantly to the overall adsorption capacity of shales. Ji et al. (2015) studied the Lower Silurian Longmaxi formation in the Upper Yangtze Platform and pointed out that total organic carbon (TOC) content, temperature and pressure are the most important factors affecting the methane adsorption capacity of shale. Tian et al. (2016) reported that TOC is a key parameter that dominates methane adsorption capacity because organic matter is the main contributor to specific surface area and micropore volume for shale. Sander et al. (2018) studied the controls on methane sorption capacity of Mesoproterozoic gas shales and found that for organic-lean shales with a TOC <2% clay may be the primary control on methane adsorption. Wang Y. et al. (2019) pointed out that the methane adsorption capacity shows a great positive correlation with TOC content, and clays also make some contribution to methane adsorption on organic-rich shales. Shang et al. (2020) reported that the methane adsorption capacity of shales is often, but not always, closely related to the TOC content, but not to clay minerals. All the above studies did not consider the influence of water on the methane adsorption of shale. Thus, methane adsorption experiments on moisture-equilibrated shale samples were produced.
Some researchers carried out equilibrium moisture treatment on rock samples prior to the experiments (Ross and Bustin, 2009; Gasparik et al., 2013; Merkel et al., 2015; Wang et al., 2018; Tian et al., 2020). For example, Ross and Bustin (2009) studied the methane adsorption capacity of shale under moisture-equilibrated condition and reported a 20–90% reduction compared to the value under dry condition. Gasparik et al. (2013) studied the adsorption characteristics of shales at different relative humidity levels under 0–25 MPa equilibrium pressure and found that when the water content is increased at a given temperature, shale gas adsorption capacity reduces and the adsorption isotherm moved downward as a result. Merkel et al. (2015) conducted the similar experiments and arrived at the same conclusions reported by Gasparik et al. (2013). Wang et al. (2018) investigated the influences of water on methane adsorption in three partially saturated shales. They found that a hint of water affects the methane adsorption less in samples with lower water contents and when the water content is increased, water clearly impedes methane adsorption. Zou et al. (2018) investigated the effect of water on methane adsorption capacity of shale and found that the water effect on methane adsorption in shales decreases with increasing pore diameter: small pores like micropores can be thoroughly blocked by adsorbed water, but bigger pores like mesopores are less likely to be blocked. Then, Zou et al. (2019) also investigated the combined effect of high temperature and moisture on methane adsorption in shale and found that moisture and high temperature can reduce the adsorbed gas content in shale individually, and the two factors have a synergistic-negative effect on methane adsorption in shale. Ren et al. (2019) studied the high-pressure methane adsorption of wet shales and found that the methane adsorption capacities of wet shales are controlled by TOC content, kerogen maturity, and the content of clay minerals, which is due to the fact that adsorbed water molecules have a large impact on the methane adsorption capacities of clay minerals but little effect on that of high maturity kerogen. Li et al. (2020) pointed out that the water content directly inhibits shale adsorption. In the process of increasing the water content, the relationship between the adsorption amount and the moisture content is negatively correlated. Tian et al. (2020) investigated the influence of pore water on the gas storage of organic-rich shale and pointed out that the adsorption space of methane onto clay mineral sufaces in micro- and mesopores is occupied by water, which results in a decrease of adsorbed gas, and the occurrence of adsorbed gas is dominated by organic matter adsorption. In addition, some scholars have also investigated the influence of water on methane adsorption through molecular simulation and found that water has an important effect on methane adsorption (Huang et al., 2018; Li et al., 2019; Chen G. et al., 2021).
The findings above show that water content is key to the methane adsorption capacity of shale. Consequently, the experiments under wet and dry conditions shall inevitably yield completely different evaluation results. Furthermore, the current geological analyses show that the actual shale reservoirs generally have a certain water saturation under original formation conditions (Ambrose et al., 2012; Liu and Wang, 2013; Fang et al., 2014). The presence of water shall undoubtedly affect the gas adsorption capacity evaluation of shale and, more importantly, the appraisal of shale gas content in the region. This necessitates the study of how water affects the gas adsorption capacity of shale.
Although some scholars have analysed the influence of water on the methane adsorption in shale, some details of the adsorption mode of methane in pore systems at different water saturation levels are not well understood. In particular, the effect of pore water on methane adsorption at different water saturation levels is unclear, and the model for illustrating how methane adsorption capacity of shale changes with depth at different water saturation levels has not been established. In this study, in order to investigate the influence of pre-adsorbed water on the methane adsorption capacity of shale and attempt to solve the above problems, the Da’anzhai Member of the Lower Jurassic Ziliujing Formation in the northeastern Sichuan Basin, China was selected as an example to conduct methane adsorption experiments in two sequences, firstly at different temperatures under dry condition and secondly at different relative humidity levels under the same temperature. On this basis, a model was established illustrating how methane adsorption capacity of shale changes with depth at different relative humidity levels. In the end, the influence of pre-adsorbed water on methane adsorption capacity in shale-gas systems was discussed. Our studies are of great significance to establish a theoretical basis for building more precise empirical correlations to quantify shale adsorbed gas content.
Materials and Methods
Materials Preparation
The samples, cored at 3756.2 m vertical depth from the Da’anzhai Member of the Lower Jurassic Ziliujing Formation in the northeastern Sichuan Basin, China, have an average TOC content of 1.43% and an average vitrinite reflectance of 1.75% reflecting high organic matter maturity, which mainly generate wet gas. The mineralogy is characterized by a high proportion of clay minerals (52.3%), moderate amount of quartz (32.5%), and low abundance of carbonates (10.1%), feldspar (2.9%) and pyrite (2.2%). The samples were crushed to 40–100 mesh and dried to constant weight at 100°C for 24 h. Both the purity of methane and helium used in the experiments are 99.999%.
Methane Adsorption Experiments
Two series of methane adsorption experiments were carried out in this study, one at different temperatures under dry condition, and the other at different relative humidity levels under the same temperature.
A part of the dried shale samples were selected to conduct methane adsorption experiments at 20, 30, 40, and 50°C, respectively. All methane adsorption isotherms were measured up to 32 MPa and fluctuations in temperature during a given isotherm were <0.2°C. The detailed steps of methane adsorption experiments can be referred to Tian et al. (2016).
Another part of the dried shale samples were used to conduct water vapor adsorption experiments using a water vapor adsorption analyzer. In the analyzer, the shale sample adsorbs water molecules until reaching an equilibrium state when the sample water content becomes stable. Four groups of shale samples were obtained using the water vapor adsorption analyzer where the relative humidity was set at 20, 40, 60, and 80%, respectively. Following the water vapor adsorption experiments, methane adsorption experiments were carried out on these four groups of samples at the same temperature. Considering the fact that methane adsorption experiments are very time-consuming and evaporation of water usually occurs at high temperature, methane adsorption experiments were only conducted at 20°C in this study. All methane adsorption isotherms were also measured up to 32 MPa and fluctuations in temperature during a given isotherm were <0.2°C. The detailed steps of water vapor adsorption experiments can be referred to Dang et al. (2021), Yang et al. (2021).
It is important to note that the amount of methane adsorbed in the experiments is the surface excess adsorption amount, not the absolute or actual adsorption amount. Therefore, conversion from excess adsorption to absolute adsorption is required for the evaluation of shale gas adsorption capacity (Rexer et al., 2013, 2014; Yang et al., 2017; Zhou et al., 2018; Chen L. et al., 2021). To simplify the calculations, the liquid density of methane at boiling temperature and ambient pressure (0.421 g/cm3) was used as the adsorbed phase density for the methane excess adsorption correction.
Langmuir Equation Fitting of Absolute Adsorption Isotherms
At present, there are two most commonly used equations for fitting methane adsorption isotherms of shale, which are Langmuir equation and DR equation (Ji et al., 2015; Tian et al., 2016; Li et al., 2018; Song et al., 2018; Wang Y. et al., 2019; Dang et al., 2020). We have conducted relevant researches about the difference between these two equations and believe that Langmuir equation is adequate for most of the practical reservoir assessments since it gives quite similar results with the DR model (Chen et al., 2017b). Therefore, Langmuir equation was used in this study for fitting the methane adsorption isotherms of shale.
Langmuir adsorption isotherm equation is the first and most widely used equation for monolayer adsorption (Ji et al., 2015; Ibad and Padmanabhan, 2020). The equation is as follows:
where, V (cm3/g) is the adsorbed gas content, P (MPa) is the gas pressure, VL (cm3/g) is the Langmuir volume reflecting the maximum gas adsorption capacity at a given temperature, and PL (MPa) is the Langmui pressure when the adsorbed gas is a half of the Langmuir volume.
Results and Discussion
Methane Adsorption Isotherms
The absolute methane adsorption isotherms of four different temperatures are shown in Figure 1A. It can be seen that the absolute adsorption amount of methane shows a gradually increasing trend as pressure increases, and the absolute adsorption isotherms are similar to the type I adsorption isotherm recommended by the International Union of Pure and Applied Chemistry (IUPAC). The characteristic of type I is that it is close to adsorption saturation after a certain pressure, which is often called Langmuir type. The maximum absolute methane adsorption amount shows a negative relationship with temperature. At the same temperature, the absolute methane adsorption amount first increases rapidly, and then slowly increases with increasing pressure, indicating slower adsorption rate at higher pressure. At the same pressure, the absolute methane adsorption amount shows a negative relationship with temperature. These understandings are similar to our previous research (Chen et al., 2019b). Subsequently, the absolute methane adsorption isotherms were fitted using the Langmuir equation, where the Langmuir volume values of the shale sample range from 1.54 to 1.69 cm3/g, and the Langmuir pressure values range from 1.75 to 2.39 MPa.
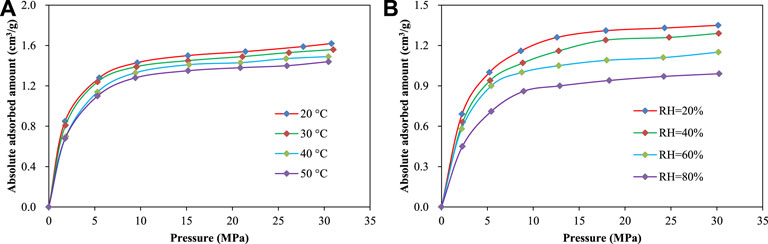
FIGURE 1. Methane absolute adsorption isotherms at (A) different temperatures under dry condition and (B) different relative humidity levels under 20°C.
The absolute methane adsorption isotherms at different relative humidity levels are shown in Figure 1B. It can also be seen that the absolute adsorption amount of methane shows a gradually increasing trend as pressure increases, and the absolute adsorption isotherms are also similar to the type I adsorption isotherm. The maximum absolute methane adsorption amount shows a negative relationship with relative humidity. At the same relative humidity, the absolute methane adsorption amount first increases rapidly, and then slowly increases with increasing pressure. At the same pressure, the absolute methane adsorption amount shows a negative relationship with relative humidity. The absolute methane adsorption isotherms were also fitted using the Langmuir equation, where the Langmuir volume values of the shale sample range from 1.12 to 1.49 cm3/g, and the Langmuir pressure values range from 2.39 to 3.22 MPa.
Effect of Temperature and Pressure on Methane Adsorption Capacity
Previous studies have shown that in addition to the physicochemical properties of shale, external factors such as temperature and pressure also have important effects on the methane adsorption capacity of shale (Gasparik et al., 2014; Tian et al., 2016; Hu H. et al., 2018; Gai et al., 2020). As shown in Figure 1A, at a given pressure, the absolute methane adsorption amount decreases with temperature. To further investigate the relationship between methane adsorption capacity of shale and temperature, the plot of Langmuir volume versus temperature was established (Figure 2A). It is obvious that Langmuir volume is negatively correlated with temperature, to put it differently, the maximum methane adsorption capacity of shale reduces with temperature. This negative relationship is highly consistent with the conclusions of previous studies (Ji et al., 2015; Tian et al., 2016; Chen et al., 2017b; Li et al., 2017) and is largely owning to the exothermic process of methane adsorption on shale (Chen et al., 2019b; Hu et al., 2021).
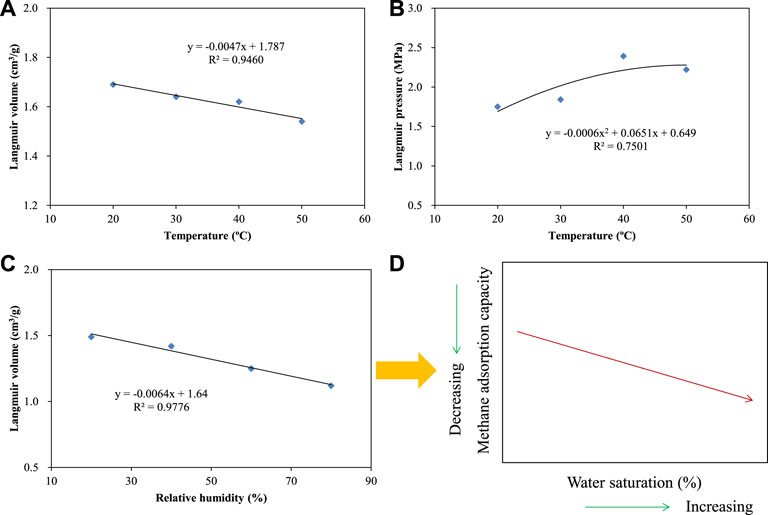
FIGURE 2. (A): Correlation between Langmuir volume and temperature. (B): Correlation between Langmuir pressure and temperature. (C): Correlation between Langmuir volume and relative humidity. (D): Correlation between methane adsorption capacity and water saturation.
In order to facilitate the establishment of the extended Langmuir equation in Methane Adsorption Capacity as Function of Depth to predict the methane adsorption capacity of shale, the plot of Langmuir pressure versus temperature was also established here (Figure 2B). It can be seen that the relationship between Langmuir pressure and temperature can be well fitted by a quadratic polynomial equation.
Generally, the adsorbed gas content increases with pressure and when the pressure exceeds a certain value the increase becomes marginal constrained by the limited specific surface area of pores. The methane adsorption isotherms of shale shown in Figure 1 show that, in low pressure regimes, the amount of gas adsorbed increases rapidly with pressure. However, beyond a certain point, adsorption rate significantly slows until adsorption saturation occurs and almost a straight-line appears towards the end of the curve. Adsorption saturation means that all the adsorption sites on the adsorbent have been occupied by the adsorbate, and the adsorption cannot continue. Ji et al. (2015) also pointed out that, other than TOC content, temperature and pressure are the most important factors affecting the methane adsorption capacity of shale from the Lower Silurian Longmaxi formation in the Upper Yangtze Platform, south China.
Effect of Relative Humidity on Methane Adsorption Capacity
The water content of shale directly affects the content of adsorbed natural gas (Merkel et al., 2015; Zou et al., 2018). In general, the higher the water content, the lower the gas adsorption capacity. Water molecules have stronger adsorption capacity than methane molecules (Liu et al., 2016), so for water-bearing shale, adsorption sites on the shale pore surface are partially occupied by water molecules, hence reducing the methane adsorption capacity of shale (Merkel et al., 2016; Li et al., 2020). It is noteworthy that typical high productivity shale gas wells in the United States have low water content (Ambrose et al., 2012), which suggests that water content is key to shale gas accumulation and production. In addition, water content also affects gas flow capacity (Hu Z. M. et al., 2018; Li et al., 2021). In summary, lower water content is favorable for shale gas exploration and production.
As shown in Figure 1B, under the same pressure, the methane adsorption capacity gradually decreases as the relative humidity increases, that is to say, the greater the water content, the lower the methane adsorption capacity. Meanwhile, the maximum methane adsorption capacity (Langmuir volume) of shale shows a negative relationship with relative humidity (Figure 2C), indicating that water content has an important effect on the methane adsorption capacity of shale. The greater the water content, the more the adsorption sites on the shale pore surface will be occupied by water molecules, thereby reducing the adsorption spaces for methane molecules and reducing the methane adsorption capacity of shale. In other words, the higher the water saturation, the lower the methane adsorption capacity, which can be represented by Figure 2D. The influence of water on methane adsorption of shale is mainly reflected in the following two aspects: 1) At low water saturation, the pore surface is partially occupied by water molecules, thus reducing the adsorption spaces for methane molecules. 2) At higher water saturation, the micropores are completely blocked by water molecules and thus unable to absorb methane molecules, while at the surface of mesopores and macropores, water molecules tend to form water film hindering the adsorption of methane molecules (Li et al., 2016, 2021; Zhu D. et al., 2021). The schematic diagram of the influence of water molecules on the methane adsorption capacity of shale is shown in Figure 3. It should be noted that the occurrence mode of water molecules in shale pores refers to the research conclusion reported by Li et al. (2016), and the occurrence mode of adsorbed methane molecules in shale pores refers to the research conclusion reported by Zhou et al. (2017). The existence of water remarkably degrades the methane adsorption capacity of shale, so the failure of taking this effect into account will result in inaccurate evaluation of shale gas reserves in-situ.
Methane Adsorption Capacity as Function of Depth
The discussions above have pointed out that temperature and relative humidity (water saturation) are the main factors influencing the methane adsorption capacity for a single shale sample. To obtain and predict the methane adsorption capacity of shale, an extended Langmuir equation was built upon the original equation.
According to multiple linear regression analysis, the relationship between Langmuir volume and temperature and relative humidity was best fitted using the following equation:
where, T (°C) is the formation temperature, and RH (%) is the relative humidity.
Using nonlinear regression method, the correlation between Langmuir pressure and temperature was established as follows:
Substituting Eqs 2, 3 into Eq. 1, an extended Langmuir equation for methane adsorption capacity estimation under geological conditions was determined:
Using this extended Langmuir equation, the methane adsorption capacity of shale under actual geological conditions can be calculated. To convert temperature and pressure to depth, a geothermal gradient of 30°C/km, a hydrostatic pressure gradient of 10 MPa/km and a nominal surface temperature of 15°C were assumed. Consequently, the methane adsorption capacity of shale as a function of burial depth was established (Figure 4).
As shown in Figure 4, the methane adsorption capacity first increases with depth due to the dominating effect of pressure, then passes through a maximum value and decreases with depth because the effect of temperature exceeds the pressure. The maximum adsorption capacity occurs at approximately from 1,600 to 2,400 m. Figure 4 also reveals that lower relative humidity (water saturation) is associated with greater maximum methane adsorption capacity and greater corresponding burial depth, and quantitatively, 20% increase of relative humidity results in roughly 10% reduction of maximum methane adsorption capacity.
Effect of Pre-Adsorbed Water on Methane Adsorption in Pore Systems
The key finding from above is that water saturation has a significant impact on methane adsorption capacity of shale. Previous studies have found that methane molecules are adsorbed to a large extent on the pore surface of organic matter and clay minerals rather than other minerals (Merkel et al., 2015; Yang et al., 2015; Ju et al., 2019; Zhu et al., 2020), and the contribution of organic matter almost doubles that of clay minerals (Yang et al., 2015). Furthermore, due to strong hydrophilicity of clay minerals, their adsorption capacity for water molecules exceeds that of organic matter (Zolfaghari et al., 2017; Chen J. et al., 2021). Based on discussions above, distinctive adsorption mode of methane molecules in shale were constructed in Figure 5 for organic matter and clay minerals in both low and high water saturations. Generally speaking, based on the observation of high-resolution electron microscope, most of the pores related to clay minerals are fracture type or strip type, and a few are spherical (Zhu H. et al., 2021). There are significant differences in pore morphology between clay minerals-associated pores and organic matter-hosted pores. However, in order to better distinguish the adsorption mode of methane in organic matter-hosted pores and clay minerals-associated pores, we regard their pore shapes as spherical in this study.
As shown in Figure 5, at low water saturation, most adsorption sites on the pore surface are occupied by methane molecules regardless of organic matter or clay minerals, and yet adsorbed methane molecules are more concentrated in the pores of organic matter. Meanwhile, at high water saturation, the micropores of both organic matter and clay minerals are blocked predominantly by water molecules and therefore barely capable of adsorbing methane molecules, while for mesopores and macropores, water molecules occupy the majority of adsorption sites forming water film, and yet clay minerals pores have higher water molecules concentration compared to organic matter counterparts. In addition, since organic matter tends to absorb more methane molecules than water molecules (Tian et al., 2020; Sun et al., 2021), its methane molecules concentration at low water saturation is higher than its water molecules concentration at high water saturation. Likewise, as clay minerals are more prone to adsorb water molecules (Zolfaghari et al., 2017; Wang T. et al., 2019; Chen J. et al., 2021), its water molecules concentration at high water saturation is higher than its methane molecules concentration at low water saturation.
Implications for Shale Adsorbed Gas Content Evaluation
Adsorption is one of the important mechanisms of shale gas occurrence (Rexer et al., 2013, 2014; Gasparik et al., 2014; Hu H. et al., 2018; Chen et al., 2019b; Gou and Xu, 2019). At present, the method for evaluating shale gas adsorption capacity is mainly through high-pressure methane adsorption experiments under dry condition, which do not account for the effect of water (Gasparik et al., 2012; Yang et al., 2015; Tian et al., 2016; Sander et al., 2018; Song et al., 2018; Gou et al., 2020; Shang et al., 2020). However, under actual formation conditions, the shale reservoir generally has a certain degree of water saturation (Ambrose et al., 2012; Fang et al., 2014). Therefore, clarifying how water affects the gas adsorption capacity of shale is of great signifiance for scientific and reasonable evaluation of shale adsorbed gas content.
Firstly, the methane adsorption experiments were conducted at different temperatures under dry condition and at different relative humidity levels under the same temperature, respectively. Then, on this basis, a model was established illustrating how methane adsorption capacity of shale changes with depth at different relative humidity levels. In the end, the influence of pre-adsorbed water on methane adsorption capacity in shale-gas systems was discussed. Our studies are of great significance to establish a theoretical basis for building more precise empirical correlations to quantify shale adsorbed gas content under actual geological conditions.
Conclusion
The main conclusions are made as follows:
1) For a single shale sample, temperature and relative humidity (water saturation) are the main factors influencing the methane adsorption capacity of shale. Methane adsorption capacity shows a decreasing trend with increasing temperature and relative humidity, respectively.
2) Lower relative humidity (water saturation) is associated with greater maximum methane adsorption capacity and greater corresponding burial depth, and quantitatively, 20% increase of relative humidity results in roughly 10% reduction of maximum methane adsorption capacity.
3) At low water saturation, most adsorption sites on the pore surface are occupied by methane molecules, and yet adsorbed methane molecules are more concentrated in the pores of organic matter.
4) At high water saturation, the micropores are blocked predominantly by water molecules and therefore barely capable of adsorbing methane molecules, while for mesopores and macropores, water molecules occupy the majority of adsorption sites forming water film, and yet clay minerals pores have higher water molecules concentration compared to organic matter counterparts.
Data Availability Statement
The original contributions presented in the study are included in the article/Supplementary Material, further inquiries can be directed to the corresponding author.
Author Contributions
LC: Conceptualization, Methodology, Writing-Original Draft, Software. ZJ: Methodology, Supervision. SJ: Resources, Investigation, Validation. SG: Formal analysis. JT: Investigation, Validation.
Funding
The authors would like to acknowledge the financial support of the National Science and Technology Major Project (No. 2017ZX05009-001), the National Natural Science Foundation of China (No. 41872135), the National Postdoctoral Program for Innovative Talent (No. BX20190387), China Postdoctoral Science Foundation (No. 2020M682262) and the Fundamental Research Funds for Central Universities (No. 20CX06014A). Special thanks are given to the China Scholarship Council (No. 201706440133) for sponsoring the first author to be a visiting scholar in the University of Utah.
Conflict of Interest
Author SG was employed by CNPC.
The remaining authors declare that the research was conducted in the absence of any commercial or financial relationships that could be construed as a potential conflict of interest.
Publisher’s Note
All claims expressed in this article are solely those of the authors and do not necessarily represent those of their affiliated organizations, or those of the publisher, the editors and the reviewers. Any product that may be evaluated in this article, or claim that may be made by its manufacturer, is not guaranteed or endorsed by the publisher.
References
Ambrose, R. J., Hartman, R. C., Diaz-Campos, M., Akkutlu, I. Y., and Sondergeld, C. H. (2012). Shale gas-in-place calculations Part I: New pore-scale considerations. SPE J. 17, 219–229. doi:10.2118/131772-pa
Chareonsuppanimit, P., Mohammad, S. A., Robinson, R. L., and Gasem, K. A. M. (2012). High-pressure adsorption of gases on shales: Measurements and modeling. Int. J. Coal Geology. 95, 34–46. doi:10.1016/j.coal.2012.02.005
Chen, G., Li, C., Lu, S., Guo, T., Wang, M., Xue, Q., et al. (2021a). Critical factors controlling adsorption capacity of shale gas in Wufeng-Longmaxi formation, Sichuan Basin: Evidences from both experiments and molecular simulations. J. Nat. Gas Sci. Eng. 88, 103774. doi:10.1016/j.jngse.2020.103774
Chen, J., Gai, H., and Xiao, Q. (2021c). Effects of composition and temperature on water sorption in overmature Wufeng-Longmaxi shales. Int. J. Coal Geology. 234, 103673. doi:10.1016/j.coal.2020.103673
Chen, L., Jiang, Z., Liu, K., Ji, W., Wang, P., Gao, F., et al. (2017b). Application of Langmuir and Dubinin-Radushkevich models to estimate methane sorption capacity on two shale samples from the Upper Triassic Chang 7 Member in the southeastern Ordos Basin, China. Energy Exploration & Exploitation 35, 122–144. doi:10.1177/0144598716684309
Chen, L., Jiang, Z., Liu, K., Tan, J., Gao, F., and Wang, P. (2017a). Pore structure characterization for organic-rich Lower Silurian shale in the Upper Yangtze Platform, South China: A possible mechanism for pore development. J. Nat. Gas Sci. Eng. 46, 1–15. doi:10.1016/j.jngse.2017.07.009
Chen, L., Jiang, Z., Liu, Q., Jiang, S., Liu, K., Tan, J., et al. (2019a). Mechanism of shale gas occurrence: Insights from comparative study on pore structures of marine and lacustrine shales. Mar. Pet. Geology. 104, 200–216. doi:10.1016/j.marpetgeo.2019.03.027
Chen, L., Liu, K., Jiang, S., Huang, H., Tan, J., and Zuo, L. (2021b). Effect of adsorbed phase density on the correction of methane excess adsorption to absolute adsorption in shale. Chem. Eng. J. 420, 127678. doi:10.1016/j.cej.2020.127678
Chen, L., Zuo, L., Jiang, Z., Jiang, S., Liu, K., Tan, J., et al. (2019b). Mechanisms of shale gas adsorption: Evidence from thermodynamics and kinetics study of methane adsorption on shale. Chem. Eng. J. 361, 559–570. doi:10.1016/j.cej.2018.11.185
Curtis, J. B. (2002). Fractured shale-gas systems. AAPG Bull. 86, 1921–1938. doi:10.1306/61eeddbe-173e-11d7-8645000102c1865d
Dang, W., Jiang, S., Zhang, J., Li, P., Nie, H., Liu, Y., et al. (2021). A systematic experimental and modeling study of water adsorption/desorption behavior in organic-rich shale with different particle sizes. Chem. Eng. J. 426, 130596. doi:10.1016/j.cej.2021.130596
Dang, W., Zhang, J., Nie, H., Wang, F., Tang, X., Wu, N., et al. (2020). Isotherms, thermodynamics and kinetics of methane-shale adsorption pair under supercritical condition: Implications for understanding the nature of shale gas adsorption process. Chem. Eng. J. 383, 123191. doi:10.1016/j.cej.2019.123191
Fang, C. H., Huang, Z. L., Wang, Q. Z., Zheng, D. W., and Liu, H. L. (2014). Cause and significance of the ultra-low water saturation in gas-enriched shale reservoir. Nat. Gas Geosci. 25, 471–476.
Gai, H., Li, T., Wang, X., Tian, H., Xiao, X., and Zhou, Q. (2020). Methane adsorption characteristics of overmature Lower Cambrian shales of deepwater shelf facies in Southwest China. Mar. Pet. Geology. 120, 104565. doi:10.1016/j.marpetgeo.2020.104565
Gao, S., Dong, D., Tao, K., Guo, W., Li, X., and Zhang, S. (2021). Experiences and lessons learned from China's shale gas development: 2005-2019. J. Nat. Gas Sci. Eng. 85, 103648. doi:10.1016/j.jngse.2020.103648
Gao, Z., Fan, Y., Xuan, Q., and Zheng, G. (2020). A review of shale pore structure evolution characteristics with increasing thermal maturities. Adv. Geo-energy Res. 4, 247–259. doi:10.46690/ager.2020.03.03
Gasparik, M., Ghanizadeh, A., Gensterblum, Y., and Krooss, B. M. (2013). "Multi-temperature" method for high-pressure sorption measurements on moist shales. Rev. Sci. Instrum 84, 085116–085752. doi:10.1063/1.4817643
Gasparik, M., Bertier, P., Gensterblum, Y., Ghanizadeh, A., Krooss, B. M., and Littke, R. (2014). Geological controls on the methane storage capacity in organic-rich shales. Int. J. Coal Geology. 123, 34–51. doi:10.1016/j.coal.2013.06.010
Gasparik, M., Ghanizadeh, A., Bertier, P., Gensterblum, Y., Bouw, S., and Krooss, B. M. (2012). High-pressure methane sorption isotherms of black shales from the Netherlands. Energy Fuels 26, 4995–5004. doi:10.1021/ef300405g
Gou, Q., Xu, S., Hao, F., Zhang, B., Shu, Z., Yang, F., et al. (2020). Quantitative calculated shale gas contents with different lithofacies: A case study of Fuling gas shale, Sichuan Basin, China. J. Nat. Gas Sci. Eng. 76, 103222. doi:10.1016/j.jngse.2020.103222
Gou, Q., and Xu, S. (2019). Quantitative evaluation of free gas and adsorbed gas content of Wufeng-Longmaxi shales in the Jiaoshiba area, Sichuan Basin, China. Adv. Geo-energ. Res. 3, 258–267. doi:10.26804/ager.2019.03.04
Hill, R. J., Zhang, E., Katz, B. J., and Tang, Y. (2007). Modeling of gas generation from the Barnett shale, Fort Worth Basin, Texas. Bulletin 91, 501–521. doi:10.1306/12060606063
Hu, H., Hao, F., Guo, X., Dai, F., Lu, Y., and Ma, Y. (2018a). Investigation of methane sorption of overmature Wufeng-Longmaxi shale in the Jiaoshiba area, Eastern Sichuan Basin, China. Mar. Pet. Geology. 91, 251–261. doi:10.1016/j.marpetgeo.2018.01.008
Hu, R., Wang, W., Tan, J., Chen, L., Dick, J., and He, G. (2021). Mechanisms of shale gas adsorption: Insights from a comparative study on a thermodynamic investigation of microfossil-rich shale and non-microfossil shale. Chem. Eng. J. 411, 128463. doi:10.1016/j.cej.2021.128463
Hu, Z. M., Duan, X. G., He, Y. B., Wu, J. F., Chang, J., Liu, L., et al. (2018b). Inflence of reservoir primary water on shale gas occurrence and flw capacity. Nat. Gas Industry 38, 44–51.
Huang, L., Ning, Z., Wang, Q., Qi, R., Zeng, Y., Qin, H., et al. (2018). Molecular simulation of adsorption behaviors of methane, carbon dioxide and their mixtures on kerogen: Effect of kerogen maturity and moisture content. Fuel 211, 159–172. doi:10.1016/j.fuel.2017.09.060
Ibad, S. M., and Padmanabhan, E. (2020). Methane sorption capacities and geochemical characterization of Paleozoic shale Formations from Western Peninsula Malaysia: Implication of shale gas potential. Int. J. Coal Geology. 224, 103480. doi:10.1016/j.coal.2020.103480
Jarvie, D. M., Hill, R. J., Ruble, T. E., and Pollastro, R. M. (2007). Unconventional shale-gas systems: The Mississippian Barnett Shale of north-central Texas as one model for thermogenic shale-gas assessment. Bulletin 91, 475–499. doi:10.1306/12190606068
Ji, W., Song, Y., Jiang, Z., Chen, L., Li, Z., Yang, X., et al. (2015). Estimation of marine shale methane adsorption capacity based on experimental investigations of Lower Silurian Longmaxi formation in the Upper Yangtze Platform, south China. Mar. Pet. Geology. 68, 94–106. doi:10.1016/j.marpetgeo.2015.08.012
Ju, Y., He, J., Chang, E., and Zheng, L. (2019). Quantification of CH4 adsorption capacity in kerogen-rich reservoir shales: An experimental investigation and molecular dynamic simulation. Energy 170, 411–422. doi:10.1016/j.energy.2018.12.087
Krooss, B. M., Littke, R., Müller, B., Frielingsdorf, J., Schwochau, K., and Idiz, E. F. (1995). Generation of nitrogen and methane from sedimentary organic matter: Implications on the dynamics of natural gas accumulations. Chem. Geology. 126, 291–318. doi:10.1016/0009-2541(95)00124-7
Li, J., Li, B., and Gao, Z. (2021). Water vapor adsorption behavior in shale under different temperatures and pore structures. Nat. Resour. Res. 30, 2789–2805. doi:10.1007/s11053-021-09846-0
Li, J., Li, B., Ren, C., Yang, K., and Zhang, Y. (2020). Characterization of methane adsorption behavior on wet shale under different temperature conditions. Energy Fuels 34, 2832–2848. doi:10.1021/acs.energyfuels.9b03929
Li, J., Li, X., Wang, X., Li, Y., Wu, K., Shi, J., et al. (2016). Water distribution characteristic and effect on methane adsorption capacity in shale clay. Int. J. Coal Geology. 159, 135–154. doi:10.1016/j.coal.2016.03.012
Li, J., Zhou, S., Gaus, G., Li, Y., Ma, Y., Chen, K., et al. (2018). Characterization of methane adsorption on shale and isolated kerogen from the Sichuan Basin under pressure up to 60 MPa: Experimental results and geological implications. Int. J. Coal Geology. 189, 83–93. doi:10.1016/j.coal.2018.02.020
Li, T., Tian, H., Xiao, X., Cheng, P., Zhou, Q., and Wei, Q. (2017). Geochemical characterization and methane adsorption capacity of overmature organic-rich Lower Cambrian shales in northeast Guizhou region, southwest China. Mar. Pet. Geology. 86, 858–873. doi:10.1016/j.marpetgeo.2017.06.043
Li, W., Pang, X., Snape, C., Zhang, B., Zheng, D., and Zhang, X. (2019). Molecular simulation study on methane adsorption capacity and mechanism in clay minerals: Effect of clay type, pressure, and water saturation in shales. Energy Fuels 33, 765–778. doi:10.1021/acs.energyfuels.8b03462
Liu, H. L., and Wang, H. Y. (2013). Ultra-low water saturation characteristics and the identification of over-pressured play fairways of marine shales in south China. Nat. Gas Industry 33, 140–144.
Liu, R., Jiang, D., Zheng, J., Hao, F., Jing, C., Liu, H., et al. (2021). Stress heterogeneity in the Changning shale-gas field, southern Sichuan Basin: Implications for a hydraulic fracturing strategy. Mar. Pet. Geology. 132, 105218. doi:10.1016/j.marpetgeo.2021.105218
Liu, R., Zheng, J., Hao, F., Nie, Z., Heng, D., Tan, X., et al. (2020). Variation in pore systems with tectonic stress in the overthrust Wufeng-Longmaxi shale of the southern Sichuan Basin, China. J. Nat. Gas Sci. Eng. 83, 103617. doi:10.1016/j.jngse.2020.103617
Liu, X.-Q., He, X., Qiu, N.-X., Yang, X., Tian, Z.-Y., Li, M.-J., et al. (2016). Molecular simulation of CH4, CO2, H2O and N2 molecules adsorption on heterogeneous surface models of coal. Appl. Surf. Sci. 389, 894–905. doi:10.1016/j.apsusc.2016.08.021
Merkel, A., Fink, R., and Littke, R. (2016). High pressure methane sorption characteristics of lacustrine shales from the Midland Valley Basin, Scotland. Fuel 182, 361–372. doi:10.1016/j.fuel.2016.05.118
Merkel, A., Fink, R., and Littke, R. (2015). The role of pre-adsorbed water on methane sorption capacity of Bossier and Haynesville shales. Int. J. Coal Geology. 147-148, 1–8. doi:10.1016/j.coal.2015.06.003
Qiao, J., Littke, R., Zieger, L., Jiang, Z., and Fink, R. (2020). Controls on gas storage characteristics of Upper Paleozoic shales from the southeastern Ordos Basin. Mar. Pet. Geology. 117, 104377. doi:10.1016/j.marpetgeo.2020.104377
Ren, W., Guo, J., Zeng, F., and Wang, T. (2019). Modeling of high-pressure methane adsorption on wet shales. Energy Fuels 33, 7043–7051. doi:10.1021/acs.energyfuels.9b01024
Rexer, T. F., Mathia, E. J., Aplin, A. C., and Thomas, K. M. (2014). High-pressure methane adsorption and characterization of pores in Posidonia shales and isolated kerogens. Energy Fuels 28, 2886–2901. doi:10.1021/ef402466m
Rexer, T. F. T., Benham, M. J., Aplin, A. C., and Thomas, K. M. (2013). Methane adsorption on shale under simulated geological temperature and pressure conditions. Energy Fuels 27, 3099–3109. doi:10.1021/ef400381v
Ross, D. J. K., and Marc Bustin, R. (2009). The importance of shale composition and pore structure upon gas storage potential of shale gas reservoirs. Mar. Pet. Geology. 26, 916–927. doi:10.1016/j.marpetgeo.2008.06.004
Sander, R., Pan, Z., Connell, L. D., Camilleri, M., Grigore, M., and Yang, Y. (2018). Controls on methane sorption capacity of Mesoproterozoic gas shales from the Beetaloo Sub-basin, Australia and global shales. Int. J. Coal Geology. 199, 65–90. doi:10.1016/j.coal.2018.09.018
Shang, F., Zhu, Y., Hu, Q., Zhu, Y., Wang, Y., Du, M., et al. (2020). Characterization of methane adsorption on shale of a complex tectonic area in Northeast Guizhou, China: Experimental results and geological significance. J. Nat. Gas Sci. Eng. 84, 103676. doi:10.1016/j.jngse.2020.103676
Song, X., Lü, X., Shen, Y., Guo, S., and Guan, Y. (2018). A modified supercritical Dubinin-Radushkevich model for the accurate estimation of high pressure methane adsorption on shales. Int. J. Coal Geology. 193, 1–15. doi:10.1016/j.coal.2018.04.008
Sun, J., Xiao, X., Wei, Q., Cheng, P., and Tian, H. (2021). Occurrence of irreducible water and its influences on gas-bearing property of gas shales from shallow Longmaxi formation in the Xishui area, Guizhou, Southern China. Front. Earth Sci. 9, 654136. doi:10.3389/feart.2021.654136
Tian, H., Li, T., Zhang, T., and Xiao, X. (2016). Characterization of methane adsorption on overmature Lower Silurian-Upper Ordovician shales in Sichuan Basin, southwest China: Experimental results and geological implications. Int. J. Coal Geology. 156, 36–49. doi:10.1016/j.coal.2016.01.013
Tian, H., Pan, L., Xiao, X., Wilkins, R. W. T., Meng, Z., and Huang, B. (2013). A preliminary study on the pore characterization of Lower Silurian black shales in the Chuandong Thrust Fold Belt, southwestern China using low pressure N2 adsorption and FE-SEM methods. Mar. Pet. Geology. 48, 8–19. doi:10.1016/j.marpetgeo.2013.07.008
Tian, H., Wang, M., Liu, S., Zhang, S., and Zou, C. (2020). Influence of pore water on the gas storage of organic-rich shale. Energy Fuels 34, 5293–5306. doi:10.1021/acs.energyfuels.9b034152020
Wang, L., Wan, J., Tokunaga, T. K., Kim, Y., and Yu, Q. (2018). Experimental and modeling study of methane adsorption onto partially saturated shales. Water Resour. Res. 54, 5017–5029. doi:10.1029/2017wr020826
Wang, T., Tian, S., Li, G., Sheng, M., Ren, W., Liu, Q., et al. (2019b). Experimental study of water vapor adsorption behaviors on shale. Fuel 248, 168–177. doi:10.1016/j.fuel.2019.03.029
Wang, Y., Liu, L., Sheng, Y., Wang, X., Zheng, S., and Luo, Z. (2019a). Investigation of supercritical methane adsorption of overmature shale in Wufeng-Longmaxi formation, southern Sichuan Basin, China. Energy Fuels 33, 2078–2089. doi:10.1021/acs.energyfuels.8b04344
Xu, S., Hao, F., Shu, Z., Zhang, A., and Yang, F. (2020). Pore structures of different types of shales and shale gas exploration of the Ordovician Wufeng and Silurian Longmaxi successions in the eastern Sichuan Basin, South China. J. Asian Earth Sci. 193, 104271. doi:10.1016/j.jseaes.2020.104271
Yang, F., Ning, Z., Zhang, R., Zhao, H., and Krooss, B. M. (2015). Investigations on the methane sorption capacity of marine shales from Sichuan Basin, China. Int. J. Coal Geology. 146, 104–117. doi:10.1016/j.coal.2015.05.009
Yang, F., Xie, C., Xu, S., Ning, Z., and Krooss, B. M. (2017). Supercritical methane sorption on organic-rich shales over a wide temperature range. Energy Fuels 31, 13427–13438. doi:10.1021/acs.energyfuels.7b02628
Yang, R., Jia, A., He, S., Hu, Q., Sun, M., Dong, T., et al. (2021). Experimental investigation of water vapor adsorption isotherm on gas-producing Longmaxi shale: Mathematical modeling and implication for water distribution in shale reservoirs. Chem. Eng. J. 406, 125982. doi:10.1016/j.cej.2020.125982
Zhang, T., Ellis, G. S., Ruppel, S. C., Milliken, K., and Yang, R. (2012). Effect of organic-matter type and thermal maturity on methane adsorption in shale-gas systems. Org. Geochem. 47, 120–131. doi:10.1016/j.orggeochem.2012.03.012
Zhou, S., Wang, H., Xue, H., Guo, W., and Li, X. (2017). Supercritical methane adsorption on shale gas: Mechanism and model. Chin. Sci. Bull. 62, 4189–4200. doi:10.1360/n972017-00151
Zhou, S., Xue, H., Ning, Y., Guo, W., and Zhang, Q. (2018). Experimental study of supercritical methane adsorption in Longmaxi shale: Insights into the density of adsorbed methane. Fuel 211, 140–148. doi:10.1016/j.fuel.2017.09.065
Zhu, D., Jiang, Z., Jiang, S., Yang, W., Song, Y., Gao, Z., et al. (2021a). Water-bearing characteristics and their influences on the reservoir capacity in terrestrial shale reservoirs: A case study of the lower Jurassic Ziliujing Formation in the Northeast Sichuan Basin, China. Mar. Pet. Geology. 123, 104738. doi:10.1016/j.marpetgeo.2020.104738
Zhu, H., Huang, C., Ju, Y., Bu, H., Li, X., Yang, M., et al. (2021b). Multi-scale multi-dimensional characterization of clay-hosted pore networks of shale using FIBSEM, TEM, and X-ray micro-tomography: Implications for methane storage and migration. Appl. Clay Sci. 213, 106239. doi:10.1016/j.clay.2021.106239
Zhu, H., Ju, Y., Huang, C., Chen, F., Chen, B., and Yu, K. (2020). Microcosmic gas adsorption mechanism on clay-organic nanocomposites in a marine shale. Energy 197, 117256. doi:10.1016/j.energy.2020.117256
Zolfaghari, A., Dehghanpour, H., and Holyk, J. (2017). Water sorption behaviour of gas shales: I. Role of clays. Int. J. Coal Geology. 179, 130–138. doi:10.1016/j.coal.2017.05.008
Zou, C., Zhao, Q., Dong, D., Yang, Z., Qiu, Z., Liang, F., et al. (2017). Geological characteristics, main challenges and future prospect of shale gas. J. Nat. Gas Geosci. 2, 273–288. doi:10.1016/j.jnggs.2017.11.002
Zou, J., Rezaee, R., Xie, Q., and You, L. (2019). Characterization of the combined effect of high temperature and moisture on methane adsorption in shale gas reservoirs. J. Pet. Sci. Eng. 182, 106353. doi:10.1016/j.petrol.2019.106353
Keywords: shale gas, pre-adsorbed water, methane adsorption, relative humidity, water saturation
Citation: Chen L, Jiang Z, Jiang S, Guo S and Tan J (2021) Effect of Pre-Adsorbed Water on Methane Adsorption Capacity in Shale-Gas Systems. Front. Earth Sci. 9:757705. doi: 10.3389/feart.2021.757705
Received: 12 August 2021; Accepted: 30 September 2021;
Published: 14 October 2021.
Edited by:
Rui Liu, Southwest Petroleum University, ChinaReviewed by:
Hongjian Zhu, Yanshan University, ChinaHao Xu, Chengdu University of Technology, China
Yang Wang, China University of Mining and Technology, China
Wei Dang, Xi’an Shiyou University, China
Copyright © 2021 Chen, Jiang, Jiang, Guo and Tan. This is an open-access article distributed under the terms of the Creative Commons Attribution License (CC BY). The use, distribution or reproduction in other forums is permitted, provided the original author(s) and the copyright owner(s) are credited and that the original publication in this journal is cited, in accordance with accepted academic practice. No use, distribution or reproduction is permitted which does not comply with these terms.
*Correspondence: Lei Chen, Y2hlbmxlaTE5ODgwODA0QDE2My5jb20=