Quantitative analysis of the risk of hydrogen sulfide release from gas hydrates
- 1Sanya Institute of South China Sea Geology, Guangzhou Marine Geological Survey, Sanya, China
- 2Academy of South China Sea Geological Science, China Geological Survey, Sanya, China
- 3Guangzhou Marine Geological Survey, Guangzhou, China
- 4Southern Marine Science and Engineering Guangdong Laboratory, Guangzhou, China
The role that H2S plays in the global sulfur cycle has been studied extensively in recent years. This paper focuses on the influence of H2S released from gas hydrates on sulfur cycle and establishes a one-dimensional mathematical model to calculate the amount of H2S released from the dissociation of gas hydrates present in multiple layers in the Qiongdongnan Basin China. The results show that the sulfate and methane transition zone that covers an area of about 100 km2in the Qiongdongnan Basin contains 2.3 × 1012 g of pyrite, which requires 4.06 × 1011 mol of H2S for its formation. The H2S released from the dissociation of gas hydrates is 5.4 ×1011 mol, which is about 1.3 times that needed for the formation of pyrite. Therefore, the H2S released from the gas hydrates is an important source of H2S for the formation of pyrite in the sulfate-methane transition zone of Qiongdongnan Basin. According to the flux of H2S and the partial pressure of O2 (PO2) in the atmosphere, the critical value of the balance between the flux of H2S and PO2 turns out to be 0.13 mol kg−1∙bar−1. Furthermore, considering the effect of global sea-level changes, three risk modes are identified to categorize the amount of H2S released from the dissociation of gas hydrate into the atmosphere. We classify the periods from 5–12 Ma BP, 25–29 Ma BP, 47–52 Ma, and 57–61 Ma BP into the high-risk mode. Furthermore, the results show that a part of the H2S released from the gas hydrate dissociation is oxidized by the Fe (III) oxide metal, with much of the metal ions being released into the pore water. Another part of the H2S is re-oxidized by the O2 in the ocean, with much of SO42- released into the seawater. Therefore, the process also provides metal ions and SO42- to pore water or seawater when the H2S released from gas hydrate diffuses from the bottom. This paper provides new insights into the source of H2S in the ocean and shows that the H2S contained in gas hydrates plays an important role in the global sulfur cycle.
1 Introduction
Pyrites are the most important sulfur sinks and are widely distributed in marine sediments (Lin et al., 2017). They have a significant influence on the sulfur cycle and have resulted in a series of studies involving major scientific issues, such as the evolution of oxygen and the origin of life (Butler et al., 2004; Chen et al., 2006; Formolo and Lyons 2013; Akhoudas et al., 2018). Therefore, many scholars have carried out detailed research on their cause and isotope composition (Hu et al., 2012; Pan et al., 2018). Two pyrite formation pathways have been proposed. One pathway is the polysulfide one:
The other is the H2S pathway, initially observed as the reaction of pyrrhotite (Fe1-xS) with H2S(aq):
There have been many studies related to the formation of pyrite, right from the identification of the macroscopic sedimentary environment to the microscopic microbial culture, and are mainly focused on the redox state of the sedimentary environment and the global C-S-Fe cycle (Peckmann and Thiel 2004; Kraal et al., 2009; Lin et al., 2016). In recent years, the influence of the local depositional environment on the formation of pyrite and its isotopes has received increasing attention, and the idea of using pyrite sulfur isotopes to trace the evolution of depositional environment (such as sea-level changes) has been put forward (Wang et al., 2018a), especially in shallow seas (Peckmann et al., 2001; Lim et al., 2011; Sima et al., 2011). During OSR and SO42--AOM processes, higher HS- concentrations are produced in the pore water and HS- reacts with Fe2+ in pore water or sediment to form pyrite. During this process, metal oxides are gradually converted into FeS and finally into pyrite under the action of excessive hydrogen sulfide. Therefore, studying the source of hydrogen sulfide has important scientific significance to understand the change of depositional environments. However, the speciation of H2S in seawater is complex, with the species most often described in terms of free sulfide (H2S+ HS−+ S2-). Furthermore, questions remain regarding the role that H2S plays in the global sulfur cycle, particularly with respect to its presence in the remote oceanic atmosphere and possible transfer across the air/sea interface. Previous studies have found that the main source of hydrogen sulfide for the formation of pyrite in marine sediments is from the anaerobic oxidation of methane (AOM) and organic sulfur reduction (OSR) (Xie et al., 2019; Wei et al., 2020). The production of hydrogen sulfide also occurs in hydrothermal systems due to geochemical processes (Yao and Millero, 1996). Huene and Pecher, (1999) summarized the H2S concentrations found in a large number of hydrothermal fluids at various locations in the Pacific and Atlantic oceans, whereas the concentrations ranged from 1.1 to 110 mmol kg−1 (Von et al., 1995). However, previous studies have focused more on the mechanism of the formation of hydrogen sulfide and the source of hydrogen sulfide from volcanic eruptions, hydrothermal flux, or from the AOM and OSR (Radford- Knwry and Cutter, 1994, Shen et al., 2008; Wu et al., 2018). Only a handful of studies have focused on hydrogen sulfide contained in hydrates. At present, H2S has been observed in gas hydrates using Raman spectroscopy at the Hydrate Ridge (Hester et al., 2007), the Nigerian Margin (Chazallon et al., 2007), and the South China Sea (Fang et al., 2019). In marine sediments, there are huge reserves of gas hydrates, which are widely distributed. There are also huge reserves of H2S in gas hydrates, which are universally present across the Earth. Moreover, H2S released from gas hydrates has an important influence on the environment. Considering the huge reserves of gas hydrates, the amount of H2S contained in gas hydrates is also huge. Furthermore, when gas hydrates dissociate, much of the H2S released from the gas hydrates also affect the environment, the sulfur cycle in the ocean, and the local ecosystem (Kastner et al., 1998). Therefore, it is important to study the role that gas hydrates play in pyrite formation.
2 Geological setting
Qiongdongnan Basin is located in the western part of the northern continental slope of the South China Sea (Figure 1). The northern part of the basin is bound by the Hainan Island, while the west is adjacent to Indochina. Additionally, the east is close to the Pearl River Mouth Basin. The Qiongdongnan Basin covers more than 80,000 km2, and approximately 60% of the basin has a water depth of more than 300 m (Wang et al., 2015). The seafloor water temperature at the Qiongdongnan Basin is 2°C–3°C, and the mean geothermal gradient is approximately 40°C/km. However, due to the common gas-bearing fluid activity in the Qiongdongnan Basin, the geothermal gradient in the study area is relatively much higher (Yuan et al., 2009). Oil and gas have been discovered in multiple reservoirs in different structural tectonic belts in the Qiongdongnan Basin (QDNB) (Zhang et al., 2014; Wang et al., 2015; W. Zhang et al., 2015; Zhang et al., 2016; Qin et al., 2019). The sediments deposited during the Pliocene and Quaternary possess favorable conditions for hosting biogenic gases, and these were commonly encountered in the strata shallower than 2,300 m during gas logging. In addition, the coal-measure source rocks deposited in the Oligocene are in the thermal evolution stage of mature-to-high-mature, with favorable conditions for thermogenic gas generation (Huang et al., 2015; Huang et al., 2017). Overpressure was common during the formation of QDNB when the rapidly filling sediments deposited in the Cenozoic became deeply buried under compaction (Shi et al., 2019; Wang et al., 2020). The mud diapirs and gas chimneys caused by overpressure are widely distributed in the deep water, providing an important vertical migration pathway for hydrocarbons and for the formation and accumulation of natural gas hydrates (Zhang et al., 2016; Wang et al., 2020). Furthermore, there is a large concentration of bivalve shells in the methane seep area (Figures 1B–E), indicating that the methane flux is higher. Meanwhile, multiple layers of gas hydrate are also found in the study area (Liang et al., 2019) (Figure 2).
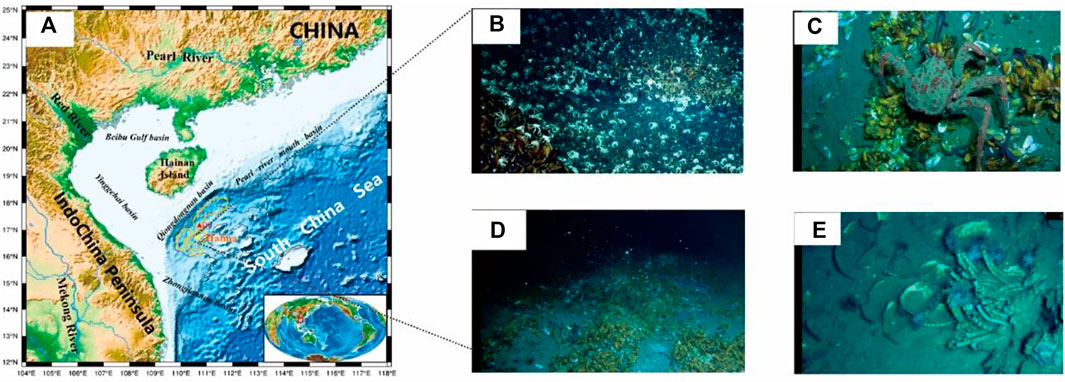
FIGURE 1. The methane seep area was observed in Qiongdongnan Basin. [(A): Study Area, (B–E): Biological status of methane leakage area].
3 Methods
In the current study, we used mass conversation equations to calculate the amount of H2S in the sink and how much H2S is released from the sink.
The total mass of H2S at any given time can be described as:
where
In the present study, the percentage of H2S in gas hydrates was confirmed by the relative peak intensities of H2S to CH4. in Spectroscopy. Moreover, their Raman quantification factor ratios were calibrated using the crystal established absolute cage occupancies of a pure H2S sample (Figure 3) (Qin and Kuhs 2013), which is similar to the procedures described in (Qin and Kuhs, 2013) for CH4 hydrate.
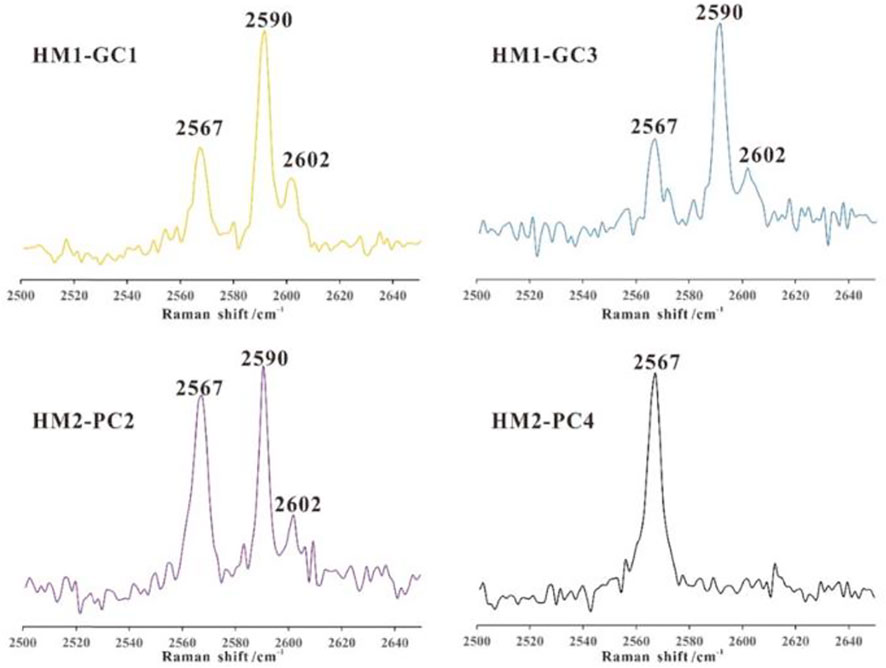
FIGURE 3. The concentration of H2S in gas hydrate at Qiongdongnan basin (Referend from huang et al., 2017).
The percentage values of the partial pressure of atmospheric oxygen (PO2) are determined by Liu et al. (2021) and the present value of PO2 is 212.28 mbar. The geological time of PO2 is determined by multiplying the fraction of
4 Results and discussion
4.1 Influence of the H2S released from gas hydrates on the formation of pyrite
In marine sediments, the formation of H2S occurs in a variety of settings. The production of H2S in the pore water of sediments and the water column of stagnant basins is due to the anaerobic oxidation of methane (Yang et al., 2007). Submarine hydrothermal emissions are also a possible source of H2S to the ocean. However, previous studies have shown that H2S released from submarine hydrothermal emissions are not easily transferred into the atmosphere and shallow sediments (Yao and Milero., 1996). Therefore, the organic sulfur reduction (OSR) and anaerobic oxidation of methane (AOM) are the main sources of H2S for the formation of pyrite (Commeau et al., 1987; Egger et al., 2015; Lin et al., 2015; Xie et al., 2019; Wu 2020; Wu, 2020; Wu, Xie, et al., 2020). However, the question remains as to how to evaluate the quantity of H2S involved in the process of the formation of pyrites. As we all know, the AOM is widely present in the seepage area of gas hydrates (Wang et al., 2018b). Pyrites are also concentrated in sulfate and methane transition zone. During the process of AOM, the methane diffused from the bottom reacts with the sulfate from the overlying water at the sulfate-methane transition zone (SMTZ). The specific process is as follows:
From Eq. 4, assuming the same reaction rate conditions, when 1 mol of methane is reduced by the sulfate, 1 mol of HS− is produced at the same time. However, during the process of pyrite formation, 2 mol HS− is needed to form 1 mol of pyrite (Peckmann et al., 2001; Canet et al., 2006; Lin et al., 2017). The specific reactions are as follows:
Moreover, 1 mol of hydrogen sulfide is provided by the AOM process, and another 1 mol is provided by another unknown mechanism. The presence of H2S in the gas hydrates provides an explanation (Qin et al., 2020). Of course, organic sulfur reduction (OSR) also provides part of the missing H2S. The specific reaction is as follows:
However, OSR often takes place in the open environment and shallower sediments as compared to AOM (Xie et al., 2019). Therefore, the hydrogen sulfide produced by the OSR is always diffused upwards under the influence of pressure gradient. Due to this reason, it is difficult for it to be diffused downwards.
In the study area, the SMTZ is mainly distributed at 6–9 mbsf in the Taixinan Basin and the content of pyrite in the sediments is about 1.16%–1.03% at Taixinan Basin (Wu et al., 2019; Wei, et al., 2020). Previous studies have shown that the content of pyrite at the Zhujiangkou Basin, where the SMTZ is present at 5–7 mbsf, is about 2.5%–2.6% (Liu et al., 2016). In the Qiongdongnan Basin, the content of pyrite lies within the range of 1%–3%, and the SMTZ is distributed at about 2–3.9 mbsf. Furthermore, the SMTZ is also very close to the water-sediment seafloor, indicating that the methane flux is high in the Qiongdongnan area (Miao et al., 2021). During the GMGS5 voyages, the methane seep area was found, which covered an area of about 100 km2 in the Qiongdongnan Basin (Figure 1). In this area, there is a rich concentration of gas hydrates and pyrites in the sediments. Based on the above analysis, the amount of pyrite in the SMTZ deposits in the methane seep area in the Qiongdongnan Basin can be calculated using the following equation
In this paper, m represents the relative molecular weight of pyrite. The depth of the SMTZ is 1.9 m, whereas the cover area is 100 km2. Moreover, Q ranges from 1.64 to 1.9 g/cm3 (Zhang et al., 2015; Liang et al., 2019). We selected a sediment density of 1.8 g/cm3 and a pyrite percentage in the sediment of 2% in the Qiongdongnan Basin (Figure 4) (Mao et al., 2021; A. Haggerty 1991; Lim et al., 2011). The calculated quantity of pyrite is 6.9 × 1012 g. Therefore, the H2S needed to form pyrite is 4.06 × 1011 mol. The same methods can be applied to calculate the quantity of H2S released from the gas hydrates (see Eq. 10).
In this equation, the Depth gas hydrate has been confirmed to be deposited at the three sediments sections (18.55–40.42 mbsf, 43.42 to 56.12 mbsf, and 58.6 to 98.42 mbsf) and was based on coring and sampling results and pilot hole LWD anomalies, which showed a high resistivity, low density, high gamma-ray values, and elevated acoustic velocity (Liang et al., 2019) Herein, the first Depth gas is 21.87 m, the second Depth gas is 13 m, and the saturation of gas hydrate is 31%. The quantity of gas hydrate in the first layer is 1.22×1015 g. The percentage of H2S in the gas hydrate ranges from 1% to 2%, and, therefore, the quantity of H2S in the gas hydrate of the first layer is 1.83 × 1013 g. The moles of H2S released from the dissociation of gas hydrates in the first layer can be calculated using the following equation:
According to this analysis, the released quantity of H2S is 5.4 ×1011 mol. The H2S released from the gas hydrate is about 1.3 times the H2S needed for the formation of pyrite. The calculation results show that the H2S released from the dissociation of gas hydrates may be the source of H2S required to form pyrites. What is more, a greater volume and continual release of H2S from the gas hydrate could cause the H2S to seep into the water and even into the atmosphere. If so, it is still not clear what its biological and chemical consequences could be.
4.2 Evaluating the concentration of H2S released from gas hydrates into the ocean and atmosphere
In general, H2S can be depleted in seawater via oxidation. Recognized loss processes include photochemical oxidation, reaction with dissolved oxygen (Millero et al., 1987), After hydrogen sulfide (HS=H2S+HS−+S2-) is produced, it mainly participates in three types of reaction processes: 1) It gradually diffuses to the seabed and enters the bottom seawater body or oxidizes during the diffusion process. The oxidants in this process include oxygen, Iron oxides and manganese-containing oxides, products include elemental sulfur (S0), complex sulfides (e.g., S2O32-) and sulfates; 2) Combine with organic matter to form organic sulfur; (three is also the most important reaction process, that is, it combines with iron-bearing minerals to form iron-bearing polysulfides and monosulfides and finally converts to pyrite. This is also the main formation mechanism of pyrite in marine sediments. In addition, a large number of laboratory studies have shown that pyrite can also be formed directly without the transformation of iron polysulfides. However, it is not all HS required for pyrite formation comes from SO42--AOM. (Kelly and Kadish, 1982). However, in marine anoxic sediments, the concentration of oxygen and is low. Yao and Millero, 1996 found that elemental sulfur was the dominant product (95–100%) produced by the oxidation of H2S by hydrous Fe(III) oxides. In fact, elemental sulfur is seldom found in the marine sediments in the Qiongdongnan Basin. Therefore, in anoxic sediments, the H2S released from gas hydrates cannot be easily consumed, except in the process of forming pyrite. Nanomolar levels (0.1–2 nM) of H2S have been found in the surface waters of the oceans (Cutter and Oatts, 1987; Iii and Tsamakis, 1989). However, the mechanism of the production and maintenance of hydrogen sulfide in surface seawater remains unclear. As is discussed in Section 4.1, if the second layer of gas hydrate dissociation results in the release of hydrogen sulfide into seawater, the main controlling factor for the release of hydrogen sulfide into the atmosphere is oxygen. In fact, in anoxic basins, mildly sulfidic deep waters are separated from the atmosphere by an oxygenated surface layer, at the base of which is a sulfide chemocline through which O2 concentrations fall to zero. Kump et al. (2005) found that a significant buildup of H2S in the deep sea could have led to toxic emissions of H2S into the atmosphere, methane accumulation, and global warming Kump et al. (2005). The question is how to evaluate the seepage of H2S into the water or atmosphere, and that the fundamental characteristic affecting the supply of H2S into the water or atmosphere is the supply of O2, whose transport through the surface layer must exceed the upwelling and diffusive flux of the reductant (H2S) from below. The flux of O2 must be at least two times the concentration of H2S given the stoichiometry of the following reaction:
The fundamental characteristic of a stable chemocline is that the supply of O2 from the atmosphere across the air-sea interface and its transport through the surface layer must exceed the upwelling and diffusive flux of the reductant (H2S) from below. Kump et al. (2005) treated the exchange of gases, including O2, between the atmosphere and ocean by using a piston-velocity formulation, whereby the flux of gases occurs at a rate (in this case, FO2) that is proportional to the contrast in gas concentrations between the atmosphere and surface ocean with the proportionality constant being the piston velocity (k):
The supply of H2S from below by upwelling (FH2S) can be written as
where u is the upwelling rate (four in m·yr−1), [H2S]deep is the concentration of H2S in deep waters (in mol·kg−1), ρoce is the density of seawater (1,002 kg/m3), and KH is the Henry’s law constant for O2 (for warm surface waters, KH is 10–3 mol kg−1·bar−1).
The critical conditions for the balance of O2 and H2S must conform to the following equations:
Given these values, and setting the surface water O2 partial pressure (PO2oce) to zero, the PO2oce critical ratio of H2S in the deep to atmospheric O2, above which the steady-state surface-water O2 concentration is zero, is given by Eq. 17.
In the study area, the dissociation of the first gas hydrate layer would cause the H2S to reach 0.135 mol kg−1, and, at present, PO2 atm is 212.28 mbar. Hence,
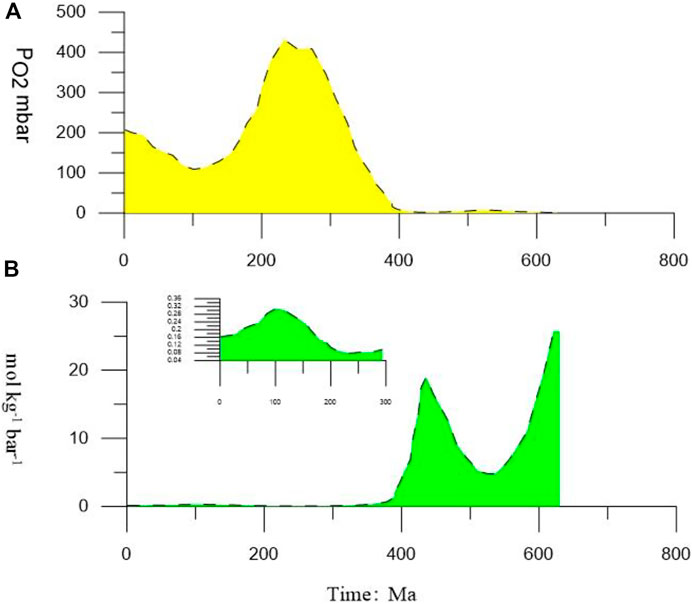
FIGURE 5. (A) Variation in the value of PO2 from present to 600 Ma BP. (B) Critical values changes from present to 600 Ma BP under the H2S released from the complete dissociation of the first layer of gas hydrate. Moreover, from the present to 400 Ma, the H2S released from the complete dissociation of the first layer of gas hydrate hardly moves into the atmosphere.
4.3 Evaluation of the influence of gas hydrate dissociation on H2S release through geological time
Gas hydrates are sensitive to temperature and pressure (Wan et al., 2022). When these factors change, the gas hydrates can dissociate. Previous studies have shown that gas hydrates are sensitive to sea-level changes (Chown et al., 2000; Blendinger 2004; Bangs and Nathan, 2005; Jang-Jun et al., 2011). A decrease in sea level will cause a corresponding change in the pressure. These pressure changes can gradually cause the gas hydrates to dissociate. In fact, in the sediments with normal methane seepage, it is difficult for the hydrogen sulfide produced by AOM to reach the water, but the existence of methane-hydrogen sulfide hydrate makes large-scale hydrate decomposition that may lead to massive hydrogen sulfide release. At this time, it is particularly important to evaluate the conditions under which the hydrogen sulfide gas reaches the water body. In this study, we assume that the gas hydrate in the first layer is more susceptible to changes in the sea-level, resulting in a dissociation of the gas hydrate in the first layer. The gas hydrate in the second layer is assumed to be gradually released, causing a 20%, 40%, 60%, 80%, and 100% release of H2S. Previous studies have shown that the H2S released from the dissociation of gas hydrates in the first layer easily seeps into the atmosphere, except during 192–307 Ma BP. When 20% of H2S is released from the gas hydrate in the second layer, it easily seeps into the atmosphere, except from 199 to 325 Ma. When the H2S released by the dissociation of gas hydrates in the second layer reaches 40%, it also easily seeps into the atmosphere, except from 199 to 273 Ma (Figure 6). When the H2S released by the dissociation of gas hydrates in the second layer reaches 60%, it easily seeps into the atmosphere, except from 212 to 293 Ma. When the H2S released by the dissociation of gas hydrates in the second layer reaches 80%, it easily seeps into the atmosphere, except from 212 to 273 Ma. When the H2S released by the dissociation of gas hydrates in the second layer reaches 100%, it easily seeps into the atmosphere, except from 232 to 273 Ma. For more precise estimates of the risk of H2S being released into the atmosphere from the dissociation of gas hydrates, we incorporated the effects of sea-level change from 100 Ma BP to today and identified three risk modes: 1) the high-risk mode indicates that the sea level decreased sharply causing large-scale gas hydrate dissociation. The PO2 value within this geological period is low. In this case, the H2S released into the atmosphere is high. 2) The moderate-risk mode indicates that the sea level decreased slower than in the high-risk mode and caused partial gas hydrate dissociation. However, the PO2 values during geological time are at a higher level. In this case, the amount of H2S released into the atmosphere was moderate. 3) The low-risk mode indicates that the sea level is high and the PO2 values during this geological period are also high. In this case, the H2S released from the gas hydrate would not easily seep into the atmosphere. Based on the three risk modes, we classified 5–12 Ma BP, 25–29 Ma BP, 47–52 Ma, and 57–61 Ma BP into the high-risk mode. In addition, we draw a conclusion through detailed analysis: When geological time of the PO2 was low and the gas hydrate’s temperature and pressure changed greatly, it caused massive gas hydrate dissociation resulting in a massive amount of H2S being released from the gas hydrates and diffusing into the pore water from the bottom. During the process, part of the H2S released from the gas hydrate was oxidized by the Fe (III) oxide metals, and part of it was used to form pyrite. Most of the H2S entered the ocean and even into the atmosphere. When geological time of the PO2 is high, the sea-level changed greatly. Meanwhile, massive H2S was released from the gas hydrate into the pore water. This released H2S will diffuse from the bottom. During the process, a small part of H2S was released from the gas hydrate (being the H2S source) to form pyrite. However, most of the H2S will enter the ocean and get re-oxidized to become sulfate by the O2 in the ocean. Furthermore, the H2S released from the dissociation of the gas hydrates would be oxidized by Fe (III) oxide metals, with much of these metal ions being released into the pore water. In addition, the H2S that was re-oxidized by the O2 in the ocean also released much of the SO42-. Therefore, the process also provides metal ions and SO42- into pore water or seawater when the H2S released from gas hydrate diffuses from the bottom.
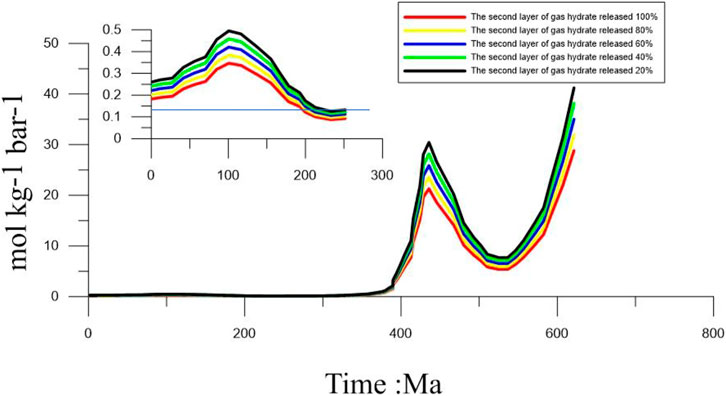
FIGURE 6. Variation in the values from 600 Ma BP under the gradual dissociation of second gas hydrate from 20% to 100%.
5 Conclusion
It is important to evaluate how H2S is released from gas hydrates. In this study, we established a one-dimensional mathematical model to calculate the amount of H2S released from multiple layers of gas hydrates in the Qiongdongnan Basin. We investigated the role that H2S released from the dissociation of gas hydrates plays on the sulfur cycle. Furthermore, we established the relationship between H2S released from gas hydrate dissociation and the concentration of H2S in the atmosphere. Our results show that the sulfate and methane transition zone (SMTZ) in the Qiongdongnan Basin contains 2.3 × 1012 g of pyrite, which requires 4.06 × 1011 mol of H2S for its formation. The amount of H2S released from the gas hydrate dissociation is 5.4 ×1011 mol, which is about 1.3 times more than that needed for the formation of pyrite. Therefore, the H2S released from the dissociation of gas hydrates is an important source of H2S for the formation of pyrites in the SMTZ in the Qiongdongnan Basin. Based on the flux of H2S and the partial pressure of O2 (PO2) in the atmosphere, we calculated the critical value of the balance between the flux of H2S and PO2 to be 0.13 mol kg−1∙bar−1. Furthermore, considering the effects of global sea-level change, we determined three risk modes to evaluate the possible seepage of H2S from gas hydrate dissociation into the atmosphere. These are as follows: 1) the high-risk mode indicates that the sea level decreased sharply causing large-scale gas hydrate dissociation. The PO2 values during this geological time are low. In this situation, there was a larger amount of H2S released into the atmosphere. 2) The moderate-risk mode indicates that the sea level decreased gradually and caused partial gas hydrate dissociation. However, the PO2 values at this geological time are higher. In this case, the amount of H2S released into the atmosphere was moderate. 3) The low-risk mode indicates that the sea level is high, and the PO2 values at this geological time are also higher (Figure 7). Therefore, it was not easy for the H2S released from the gas hydrate dissociation to seep into the atmosphere. Based on the three risk modes, we classified 5–12 Ma BP, 25–29 Ma BP, 47–52 Ma, and 57–61 Ma BP into the high-risk mode. Furthermore, the H2S released from the gas hydrate was oxidized by Fe (III) oxide metals, with much of the metal ions being released into the pore water. The H2S that was re-oxidized by the O2 in the ocean also released a lot of SO42- (Figure 8). Therefore, the whole process also provides the raw materials for the process itself. This paper provides new insights into the source of H2S found in the atmosphere and shows that the H2S contained in gas hydrates possibly plays an important role in the global sulfur cycle.
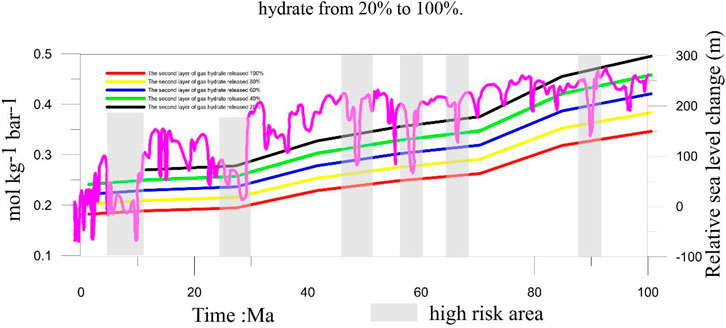
FIGURE 7. Risk ranging from present to 100 Ma BP. The purple curve represents the change in global sea level. The grey area represents the high risk of H2S going into the atmosphere.
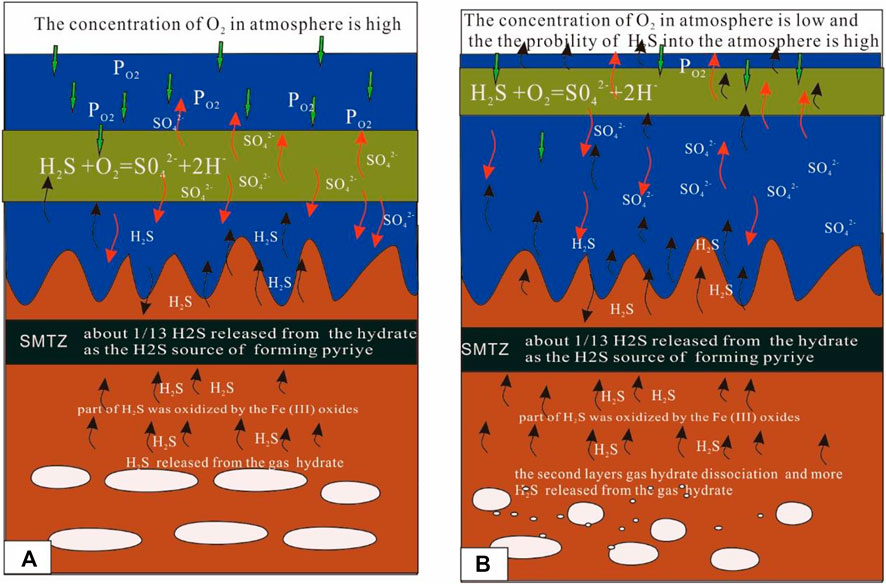
FIGURE 8. Mode of the sulfur cycle. The results show that a part of the H2S released from the dissociation of the gas hydrate is oxidized by Fe (III) oxide metal, with much of the metal ions being released into the pore water. Another part of the H2S is re-oxidized by the O2 in the ocean, with much of SO42- released into the seawater. Therefore, the process also provides metal ions and SO42- to pore water or seawater when the H2S released from the gas hydrate diffuses from the bottom. The difference in A and B is the level of PO2. In (A), the PO2 is high and the released hydrogen sulfide from the decomposition of hydrate finds it difficult to enter the atmosphere. In (B), the PO2 is low and the released hydrogen sulfide from the decomposition of hydrate finds it easy to enter the atmosphere.
Data availability statement
The original contributions presented in the study are included in the article/supplementary material, further inquiries can be directed to the corresponding author.
Author contributions
XW: Conceptualization, Methodology, Writing—Original Draft. SL: Data Curation. BZ: Conceptualization, Supervision, Writing—Review and Editing. YY and GW: Supervision. RX: Writing—Review and Editing. YF and ZN: Funding Acquisition, Resources, Supervision. All authors contributed to the manuscript.
Funding
The research was supported by the Project of Sanya Yazhou Bay Science and Technology City (No. SCKJ-JYRC-2022-14), GuangDong Basic and Applied Basic Research Foundation (No. 2020A1515110405), Geology Investigation Project of China Geological Survey (No. DD20221725), National Natural Science Foundation of China (No. 42106078).
Conflict of interest
The authors declare that the research was conducted in the absence of any commercial or financial relationships that could be construed as a potential conflict of interest.
Publisher’s note
All claims expressed in this article are solely those of the authors and do not necessarily represent those of their affiliated organizations, or those of the publisher, the editors and the reviewers. Any product that may be evaluated in this article, or claim that may be made by its manufacturer, is not guaranteed or endorsed by the publisher.
References
Akhoudas, C., Chevalier, N., Blanc-Valleron, M. M., Klein, V., Mendez-Millan, M., Demange, J., et al. (2018). Methane-derived stromatolitic carbonate crust from an active fluid seepage in the Western basin of the sea of marmara: Mineralogical, isotopic and molecular geochemical characterization. Deep Sea Res. Part II Top. Stud. Oceanogr. 153, 110–120. doi:10.1016/j.dsr2.2017.12.022
Bangs, N. L. B., Musgrave, R. J., and Trehu, A. M. (2005). Upward shifts in the southern hydrate ridge gas hydrate stability zone following postglacial warming, offshore Oregon. J. Geophys. Res. 110, B03102. doi:10.1029/2004jb003293
Blendinger, W. (2004). sea level changes versus hydrothermal diagenesis: Origin of triassic carbonate platform cycles in the dolomites, Italy. Sediment. Geol. 178, 141–144. doi:10.1016/j.sedgeo.2005.03.001
Butler, I. B., Bottcher, M. E., Rickard, D., and Oldroyd, A. (2004). Sulfur isotope partitioning during experimental formation of pyrite via the polysulfide and hydrogen sulfide pathways: Implications for the interpretation of sedimentary and hydrothermal pyrite isotope records. Earth Planet. Sci. Lett. 228 (3-4), 495–509. doi:10.1016/j.epsl.2004.10.005
Canet, C., Prol-Ledesma, R. M., Escobar-Briones, E., Mortera-Gutiérrez, C., Morales-Puente, P., Linares, C., et al. (2006). Mineralogical and geochemical characterization of hydrocarbon seep sediments from the gulf of Mexico. Mar. Petroleum Geol. 23 (5), 605–619. doi:10.1016/j.marpetgeo.2006.05.002
Chazallon, B., Focsa, C., Charlou, J. L., Bourry, C., and Donval, J. P. (2007). A comparative Raman spectroscopic study of natural gas hydrates collected at different geological sites. Chem. Geol. 244 (1-2), 175–185. doi:10.1016/j.chemgeo.2007.06.012
Chen, D. F., Dong, F., Zheng, S., Zhi, G. S., Chen, G. Q., and Iii, L. (2006). Pyrite crystallization in seep carbonates at gas vent and hydrate site. Mater. Sci. Eng. C 26 (4), 602–605. doi:10.1016/j.msec.2005.08.037
Chown, E. H., N'Dah, E., and Mueller, W. U. (2000). The relation between iron-formation and low temperature hydrothermal alteration in an archean volcanic environment. Precambrian Res. 101 (2), 263–275. doi:10.1016/s0301-9268(99)00091-1
Commeau, R. F., Paull, C. K., Commeau, J. A., and Poppe, L. J. (1987). Chemistry and mineralogy of pyrite-enriched sediments at a passive margin sulfide brine seep: Abyssal gulf of Mexico. Earth Planet. Sci. Lett. 82 (1-2), 62–74. doi:10.1016/0012-821x(87)90107-5
Cutter, G. A., and Oatts, T. J. (1987). Determination of dissolved sulfide and sedimentary sulfur speciation using gas chromatography-photoionization detection. Anal. Chem. 59 (5), 717–721. doi:10.1021/ac00132a008
Egger, M., Rasigraf, O., Sapart, C. J., Jilbert, T., Jetten, M., Roeckmann, T., et al. (2015). Iron-mediated anaerobic oxidation of methane in brackish coastal sediments. Environ. Sci. Technol. 49 (1), 277–283. doi:10.1021/es503663z
Fang, Y., Wei, J., Lu, H., Liang, J., Cao, J., Fu, J., et al. (2019). Chemical and structural characteristics of gas hydrates from the haima cold seeps in the qiongdongnan basin of the south China sea. J. Asian Earth Sci. 182, 103924. doi:10.1016/j.jseaes.2019.103924
Formolo, M. J., and Lyons, T. W. (2013). Sulfur biogeochemistry of cold seeps in the green canyon region of the gulf of Mexico. Geochimica Cosmochimica Acta 119, 264–285. doi:10.1016/j.gca.2013.05.017
Haggerty, J. A. (1991). Evidence from fluid seeps atop serpentine seamounts in the mariana forearc: Clues for emplacement of the seamounts and their relationship to forearc tectonics. Mar. Geol. 102 (1-4), 293–309. doi:10.1016/0025-3227(91)90013-t
Hester, K. C., Dunk, R. M., White, S. N., Brewer, P. G., Peltzer, E. T., and Sloan, E. D. (2007). Gas hydrate measurements at hydrate ridge using Raman spectroscopy. Geochimica Cosmochimica Acta 71 (12), 2947–2959. doi:10.1016/j.gca.2007.03.032
Hu, Y., Li, H., and Xu, J. (2012). Shallow gas accumulation in a small estuary and its implications: A case history from in and around xiamen bay. Geophys. Res. Lett. 39 (24), 2012GL054478. doi:10.1029/2012gl054478
Huang, H., Huang, B., Huang, Y., Li, X., and Tian, H. (2017). Condensate origin and hydrocarbon accumulation mechanism of the deepwater giant gas field in Western south China sea: A case study of lingshui 17-2 gas field in Qiongdongnan basin. Petroleum Explor. Dev. 44, 409–417. doi:10.1016/s1876-3804(17)30047-2
Huang, L., Su, Z., and Wu, N. Y. (2015). Evaluation on the gas production potential of different lithological hydrate accumulations in marine environment. Energy 91, 782–798. doi:10.1016/j.energy.2015.08.092
Huene, R. V., and Pecher, I. A. (1999). Vertical tectonics and the origins of bsrs along the Peru margin. Earth Planet. Sci. Lett. 166 (1-2), 47–55. doi:10.1016/s0012-821x(98)00274-x
Iii, G., and Tsamakis, E. (1989). Concentration and form of dissolved sulfide in the oxic water column of the ocean. Mar. Chem. 27 (3-4), 165–177. doi:10.1016/0304-4203(89)90046-7
Jang-Jun, B., Um, I. K., and Holland, M. (2011). Core lithologies and their constraints on gas-hydrate occurrence in the east sea, offshore Korea: Results from the site ubgh1-9. Mar. Petroleum Geol. 28 (10), 1943–1952. doi:10.1016/j.marpetgeo.2010.12.003
Kastner, M., Kvenvolden, K. A., and Lorenson, T. D. (1998). Chemistry, isotopic composition, and origin of a methane-hydrogen sulfide hydrate at the cascadia subduction zone. Earth Planet. Sci. Lett. 156 (3–4), 173–183. doi:10.1016/s0012-821x(98)00013-2
Kelly, S. L., and Kadish, K. M. (1982). Counterion and solvent effects on the electrode reactions of manganese porphyrins. Inorg. Chem. 21, 3631–3639. doi:10.1021/ic00140a010
Kraal, P., Slomp, C. P., Forster, A., Kuypers, M., and Sluijs, A. (2009). Pyrite oxidation during sample storage determines phosphorus fractionation in carbonate-poor anoxic sediments. Geochimica Cosmochimica Acta 73 (11), 3277–3290. doi:10.1016/j.gca.2009.02.026
Kump, L. R., Pavlov, A., and Arthur, M. A. (2005). Massive release of hydrogen sulfide to the surface ocean and atmosphere during intervals of oceanic anoxia. Geol. 33 (5), 397–400. doi:10.1130/g21295.1
Liang, J., Zhang, W., Lu, J., Wei, J., and He, Y. (2019). Geological occurrence and accumulation mechanism of natural gas hydrates in the eastern Qiongdongnan basin of the South China sea: Insights from site gmgs5-w9-2018. Mar. Geol. 418, 106042. doi:10.1016/j.margeo.2019.106042
Lim, Y, C., Lin, S., Yang, T, F., Chen, Y, G., and Liu, C, S. (2011). Variations of methane induced pyrite formation in the accretionary wedge sediments offshore southwestern Taiwan. Mar. Petroleum Geol. 28 (10), 1829–1837. doi:10.1016/j.marpetgeo.2011.04.004
Lin, Q., Wang, J. S., Shaoying, F. U., Hongfeng, L. U., Qingtao, B. U., Lin, R. X., et al. (2015). Elemental sulfur in northern south China sea sediments and its significance. Sci. China Earth Sci. 58, 2271–2278. doi:10.1007/s11430-015-5182-7
Lin, Q., Wang, J., Taladay, K., Lu, H., Hu, G., Sun, F., et al. (2016). Coupled pyrite concentration and sulfur isotopic insight into the paleo sulfate-methane transition zone (smtz) in the northern south China sea. J. Asian Earth Sci. 115, 547–556. doi:10.1016/j.jseaes.2015.11.001
Lin, Z., Sun, X., Strauss, H., Lu, Y., Gong, J., Xu, L., et al. (2017). Multiple sulfur isotope constraints on sulfate-driven anaerobic oxidation of methane: Evidence from authigenic pyrite in seepage areas of the South China sea. Geochimica Cosmochimica Acta 211, 153–173. doi:10.1016/j.gca.2017.05.015
Liu, S. W., Lai, Z. P., Wang, Y. X., Fan, X. L., Wang, L. L., Tian, M. Z., et al. (2016). Growing pattern of mega-dunes in the badain jaran desert in China revealed by luminescence ages. Quat. Int. 410, 111–118. doi:10.1016/j.quaint.2015.09.048
Liu, X. M., Kah, L. C., Knoll, A. H., Cui, H., Hazen, R. M., Bekker, A., et al. (2021). A persistently low level of atmospheric oxygen in Earth's middle age. Nat. Commun. 12 (1), 351. doi:10.1038/s41467-020-20484-7
Mao, S. H., Qin, J. Z., and Li, Y. K. (2021). Re: Mandatory submission of patient identifiable information to third parties: Fgm now, what next? BMJ 17 (4), 219–220. doi:10.1136/bmj.h5146
Miao, X., Feng, X., Li, J., and Lin, L. (2021). Tracing the paleo-methane seepage activity over the past 20, 000 years in the sediments of qiongdongnan basin, northwestern south China sea. Chem. Geol. 559, 119956. doi:10.1016/j.chemgeo.2020.119956
Millero, F. J., Sotolongo, S., and Izaguirre, M. (1987). Oxidation kinetics of fe(ii) in sea water. Geochim. Cosmochim. acta 51, 793–801. doi:10.1016/0016-7037(87)90093-7
Pan, M., Wu, D., Yang, F., Sun, T., Wu, N., and Liu, L. (2018). Geochemical sedimentary evidence from core 973-2 for methane activity near the jiulong methane reef in the northern south China sea. Interpretation 6, 1–39. doi:10.1190/INT-2017-0001.1
Peckmann, J., Reimer, A., Luth, U., Luth, C., Reitner, J., Heinicke, C., et al. (2001). Methane-derived carbonates and authigenic pyrite from the northwestern black sea. Mar. Geol. 177 (1-2), 129–150. doi:10.1016/s0025-3227(01)00128-1
Peckmann, J., and Thiel, V. (2004). Carbon cycling at ancient methane-seeps. Chem. Geol. 205 (3-4), 443–467. doi:10.1016/j.chemgeo.2003.12.025
Qin, J., and Kuhs, W. F. (2013). “Quantitative Raman scattering of guest molecules in gas hydrates and its relevance to gas exchange processes involving N2,” in Proceedings of the The Tenth (2013) ISOPE Ocean Mining and Gas Hydrates Symposium, Szczecin, Poland, September 2013.
Qin, X., Liang, Q., Ye, J., Yang, L., Kou, B., Xie, W., et al. (2020). The response of temperature and pressure of hydrate reservoirs in the first gas hydrate production test in south China sea. Appl. Energy 278, 115649. doi:10.1016/j.apenergy.2020.115649
Qin, X. W., Zhao, B., Li, F. Y., Zhang, B. J., Wang, H. J., Zhang, R. W., et al. (2019). Deep structure research of the South China sea: Progresses and directions. China Geol. 2 (4), 530–540. doi:10.31035/cg2018125
Radford- Knwry, J., and Cutter, G. A. (1994). Biogeochemistry of dissolved hydrogen sulfide species and carbonyl sulfide in the Western north atlantic ocean. Geochimica Cosmochimica Acta 58 (24), 5421–5431. doi:10.1016/0016-7037(94)90239-9
Shen, B., Mao, S. H., Kaufman, A. J., Bao, H., Zhou, C., and Wang, H. (2008). Stratification and mixing of a post-glacial Neoproterozoic ocean: Evidence from carbon and sulfur isotopes in a cap dolostone from northwest China. Earth Planet. Sci. Lett. 265, 209–228. doi:10.1016/j.epsl.2007.10.005
Shi, Y. H., Liang, Q. Y., Yang, J. P., Yuan, Q. M., Wu, X. M., and Kong, L. (2019). Stability analysis of submarine slopes in the area of the test production of gas hydrate in the south China sea. China Geol. 2, 274–284. doi:10.31035/cg2018122
Sima, M., Dold, B., Frei, L., Senila, M., Balteanu, D., and Zobrist, J. (2011). 'Sulfide oxidation and acid mine drainage formation within two active tailings impoundments in the Golden Quadrangle of the Apuseni Mountains, Romania. J. Hazard. Mater. 189, 624–639. doi:10.1016/j.jhazmat.2011.01.069
Wan, Z. F., Zhang, W., Ma, C., Liang, J. Q., Li, A., Meng, D. J., et al. (2022). Dissociation of gas hydrates by hydrocarbon migration and accumulation-derived slope failures:an example from the south China sea. Geosci. Front. 13 (2), 101345. doi:10.1016/j.gsf.2021.101345
Wang, J., Wu, S., Kong, X., Ma, B., Li, W., Wang, D., et al. (2018a). Subsurface fluid flow at an active cold seep area in the qiongdongnan basin, northern south China sea. J. Asian Earth Sci. 168, 17–26. doi:10.1016/j.jseaes.2018.06.001
Wang, J., Wu, S., and Yao, Y. (2018b). Quantifying gas hydrate from microbial methane in the south China sea. J. Asian Earth Sci. 168, 48–56. doi:10.1016/j.jseaes.2018.01.020
Wang, S., Magalhães, V. H., Pinheiro, L. M., Liu, J., and Yan, W. (2015). Tracing the composition, fluid source and formation conditions of the methane-derived authigenic carbonates in the gulf of cadiz with rare Earth elements and stable isotopes. Mar. Petroleum Geol. 68, 192–205. doi:10.1016/j.marpetgeo.2015.08.022
Wang, X., Liu, B., Jin, J., Lu, J., Zhou, J., Qian, J., et al. (2020). Increasing the accuracy of estimated porosity and saturation for gas hydrate reservoir by integrating geostatistical inversion and lithofacies constraints. Mar. Petroleum Geol. 115, 104298. doi:10.1016/j.marpetgeo.2020.104298
Wei, J., Li, J., Wu, T., Zhang, W., Li, J., Wang, J., et al. (2020). Geologically controlled intermittent gas eruption and its impact on bottom water temperature and chemosynthetic communities—a case study in the “HaiMa” cold seeps, south China sea. Geol. J. 55 (9), 6066–6078. doi:10.1002/gj.3780
Wu, D., Sun, T., Xie, R., Pan, M., Wu, N., Ye, Y., et al. (2019). Characteristics of authigenic minerals around the sulfate-methane transition zone in the methane-rich sediments of the northern south China sea: Inorganic geochemical evidence. Int. J. Environ. Res. Public Health 16 (13), 2299. doi:10.3390/ijerph16132299
Wu, D., Xie, R., Liu, J., Yang, F., Wu, N., Liu, L., et al. (2020). Zone of metal-driven anaerobic oxidation of methane is an important sink for phosphorus in the taixinan basin, south China sea. Mar. Geol. 427, 106268. doi:10.1016/j.margeo.2020.106268
Wu, T., Deng, X., Yu, Z., and Wang, L. (2020). Acoustic characteristics of cold-seep methane bubble behavior in the water column and its potential environmental impact. Acta Oceanol. Sin. 39 (5), 133–144. doi:10.1007/s13131-019-1489-0
Wu, T., Wei, J., Liu, S., Guan, Y., Zhang, R., Su, M., et al. (2018). Characteristics and formation mechanism of seafloor domes on the north-eastern continental slope of the south China sea. Geol. J. 55, 1–10. doi:10.1002/gj.3402
Xie, R., Wu, D., Liu, J., Sun, T., and Wu, N. (2019). Evolution of gas hydrates inventory and anaerobic oxidation of methane (aom) after 40ka in the taixinan basin, south China sea. Deep Sea Res. Part I Oceanogr. Res. Pap. 152, 103084. doi:10.1016/j.dsr.2019.103084
Yang, X., Liu, Y., Li, C., Song, Y., Zhu, H., and Jin, X. (2007). Rare Earth elements of aeolian deposits in Northern China and their implications for determining the provenance of dust storms in Beijing. Geomorphology 87, 365–377. doi:10.1016/j.geomorph.2006.10.004
Yao, W., and Millero, F. J. (1996). Oxidation of hydrogen sulfide by hydrous fe(iii) oxides in seawater. Mar. Chem. 52, 1–16. doi:10.1016/0304-4203(95)00072-0
Yuan, S., Wu, S., Thomas, L., Yao, G., Lv, F., Cao, F., et al. (2009). Fine-grained pleistocene deepwater turbidite channel system on the slope of qiongdongnan basin, northern south China sea. Mar. Petroleum Geol. 26 (8), 1441–1451. doi:10.1016/j.marpetgeo.2009.03.007
Zhang, G., Liang, J., Lu, J., Yang, S., Zhang, M., Holland, M., et al. (2015). Geological features, controlling factors and potential prospects of the gas hydrate occurrence in the east part of the pearl river mouth basin, south China sea. Mar. Petroleum Geol. 67, 356–367. doi:10.1016/j.marpetgeo.2015.05.021
Zhang, M., Konishi, H., Xu, H., Sun, X., Lu, H., Wu, D., et al. (2014). Morphology and formation mechanism of pyrite induced by the anaerobic oxidation of methane from the continental slope of the ne south China sea. J. Asian Earth Sci. 92, 293–301. doi:10.1016/j.jseaes.2014.05.004
Keywords: sulfur cycle, hydrogen sulfide, pyrite formation, anaerobic oxidation, methane
Citation: Wang X, Liu S, Zhao B, Yao Y, Wu G, Xie R, Fu Y and Ning Z (2023) Quantitative analysis of the risk of hydrogen sulfide release from gas hydrates. Front. Earth Sci. 10:1018325. doi: 10.3389/feart.2022.1018325
Received: 13 August 2022; Accepted: 31 October 2022;
Published: 18 January 2023.
Edited by:
Lihua Zuo, Texas A&M University Kingsville, United StatesReviewed by:
Jiliang Wang, Institute of Deep-Sea Science and Engineering (CAS), ChinaQian-Zhi Zhou, Sun Yat-sen University, China
Copyright © 2023 Wang, Liu, Zhao, Yao, Wu, Xie, Fu and Ning. This is an open-access article distributed under the terms of the Creative Commons Attribution License (CC BY). The use, distribution or reproduction in other forums is permitted, provided the original author(s) and the copyright owner(s) are credited and that the original publication in this journal is cited, in accordance with accepted academic practice. No use, distribution or reproduction is permitted which does not comply with these terms.
*Correspondence: Bin Zhao, zbin_a@mail.cgs.gov.cn