- Heilongjiang Province Key Laboratory of Geographical Environment Monitoring and Spatial Information Service in Cold Regions, School of Geographical Sciences, Harbin Normal University, Harbin, China
Climate change is one of the greatest threats to high-latitude permafrost and leads to serious permafrost degradation. However, few attention has been paid to whether peat soil carbon or nitrogen is sensitive to permafrost degradation. This study has selected three typical sample areas (MoHe-continuous permafrost, TaHe-Island-shaped melting permafrost, Jagdaqi-Island-shaped melting permafrost) as research object to compare the response rate and degree of peat soil carbon and nitrogen under permafrost degradation. The results show that soil organic carbon and nitrogen contents are the highest in 0–10 cm soil and permafrost regions show obvious surface aggregation. The carbon content of different types of frozen soil decreases with the depth of soil layer, and the differences are significant (p < 0.01). The distribution pattern of total nitrogen content in each soil layer among different permafrost types is Mohe < Tahe < Jagedaqi. And when it is getting vertically deeper than the surface layer, there is no significant difference between the soil layers in soil profile. The study also focuses on the variations of carbon and nitrogen content in different soil layers of peatland in typical permafrost regions. The results show that soil carbon responds faster to the degradation of frozen soil than soil nitrogen. Moreover, the accumulation degree of soil carbon is also significantly higher than soil nitrogen. Under climate change and for better permafrost conservation, it is necessary to study how the peatland’s soil carbon and the nitrogen are influenced by the permafrost degradation in high latitude.
Introduction
Climate change is one of the greatest threats to global carbon cycle. Over the past three decades, climate change caused by permafrost degradation has greatly affected soil organic carbon and organic carbon components (He et al., 2021; Yang et al., 2021; Yang et al., 2022). However, as part of the carbon pool, nitrogen does not receive equal attention. Higher availability of soil nitrogen not only increases organic carbon accumulation but also reduces the decomposition rate of organic carbon. The changing pattern of soil carbon and nitrogen distribution under global warming are crucial to the research of permafrost degradation and climate change (Gorham, 1991; Zimov et al., 2006; Pizano et al., 2014), but the accumulation of carbon and nitrogen in permafrost peatlands has received little attention. The study of severely degradated permafrost is necessary for better conservation of soil carbon and nitrogen in the peatlands.
Permafrost, which occupies 23.9% of the land in the Northern hemisphere and covers a large amount of soil organic carbon and nitrogen, is a vital soil carbon pool. The northern high-latitude permafrost regions, which store 1/3 of the global soil organic carbon, are most sensitive to climate change (Mack et al., 2004). The CO2 emissions due to global warming have caused changes in peat dryness and humidity to as high as 80 ± 20 Pg and the value will reach 249 ± 38 Pg by the end of the 21st century (IPCC, 2007). CO2 and CH4 are both important greenhouse gases, among which CH4 emissions account for approximately 3% (IPCC, 2007) of the total. Studies have shown that temperature and humidity greatly affect the mineralization of soil nitrogen. Under suitable water condition, higher temperature will maintain microbial respiration and improve the mineralization of soil nitrogen (Sierra, 1997; Gao et al., 2008; Guntiñas et al., 2012).
Peatland is an important carbon pool for terrestrial ecosystems and it has a regulating effect on global climate change. The continued increase in temperature alters the freezing and thawing patterns of the frozen soil and peatland in cold regions. In the northern part of the Greater DaXing’an Mountains, the thickness of the active layer in the wetland permafrost area has increased by 20–40 cm. As a result, a large number of greenhouse gas are released from peatlands, which may change the functions of the carbon sources and sinks in peatland, and significantly affect the biogeochemical cycle of carbon and nitrogen in the region (Zhou et al., 2016; Song et al., 2018; Xu et al., 2021; Zhao et al., 2021). Moreover, the biochemical reactions of the soil, the interaction between soil bacteria and fungi, and the microbial physiological capacity are closely related to available nutrients (Yan et al., 2009; Yang et al., 2014). The most common bacteria is the heterotrophic denitrifying bacteria. The conditions for denitrification include low oxygen, high nitrate concentration, and carbon availability. The decrease in soil availability will inhibit the denitrification (Zhang, 2011; Luo, 2014). As denitrifying bacteria requires different ratios of carbon to nitrogen to complete optimal denitrification, changes in nitrogen availability will directly affect the abundance and composition of the soil microbial communities. Therefore, the balance of carbon and nitrogen is very important for soil nutrients and microbial activity in peatlands. However, more studies are required to fully understand variations in the soil carbon and nitrogen in permafrost regions.
Soil carbon storage in peatlands serves as a critical indicator of climate change (Gao et al., 2007). High-latitude permafrost and bog peatland concentrate in the cold region of northeastern China. The decomposition rate of soil organic carbon (SOC) increases with temperature, resulting in a large loss of carbon stored in the soil (Cox et al., 2000; Davidson et al., 2000). Therefore, global warming and permafrost degradation greatly affect the carbon pool of marsh ecosystem and the carbon cycle in this region. According to Joosten’s statistics, peatlands contain one third of the global soil carbon reserve, equal to 75% of the global atmospheric carbon pool (Joosten et al., 2003). These soils are very sensitive to rising temperature (Mu et al., 2015; Schuur et al., 2015; Cheng et al., 2019). Global warming accelerates the thawing of permafrost. In permafrost regions, the warming changes the extremely low temperature of peatland soils, resulting in the decomposition of organic matter and the release of carbon CO2 and CH4 (Schuur and Abbott, 2011; Sierra et al., 2015; Wu and Wu, 2020). In turn, the massive emission of greenhouse gases also accelerates global warming, and thus a vicious cycle is formed (Dorrepaal et al., 2009; Zhang, 2017).
Nitrogen is the most important limiting nutrient in peat soil. Its content will seriously affect the productivity and carbon storage of the wetland ecosystems (Mistch and Gosselink, 2020). Nitrogen availability increases with soil temperature. When temperature becomes higher, the net primary productivity of the nitrogen-limiting ecosystem (Kaiser and Benner, 2009) and the carbon storage of the ecosystem also improves. Ammonia nitrogen and nitrate nitrogen play an important role in the nitrogen cycle (Kaiser and Benner, 2009). Turner and Henry (2010) investigated the impact of rising temperature on soil nitrogen, they demonstrated that higher temperature would increase net nitrogen mineralization in soil and the possibility of soil nitrogen loss. Shibata (2016) revealed that global warming significantly affected the size of soil nitrogen pool and the transformation of microbial nitrogen. The experimental results of Reinmann et al. (2019) demonstrated that elevated temperature decreased soil capacity for microbial nitrogen fixation and soil nitrogen uptake, increased the mortality of plant fine roots, and increased nitrogen pools.
This study has selected some peatlands in the permafrost regions of the Greater Da Xing’an Mountains as the research region. This region contains three typical permafrost types (large continuous permafrost regions, island areas in permafrost regions, and island permafrost regions). It aims to compare the vertical changing patterns of carbon and nitrogen under different degradation degrees. Its focus is on factors that affect the variations in: 1) soil carbon and nitrogen; and 2) the microbial effects on organic carbon and nitrogen. The results will offer a reference for studying the permafrost peatlands in cold regions and shed some lights on the response mechanism of carbon and nitrogen cycles to the climate change.
Materials and Methods
Study Area
The study area is located in the permafrost region of the Greater DaXing’an Mountains, (52°20′N, 124°42′E) where temperate continental climate prevails. Spreading from the south to the north, there are three types of permafrost, including the sporadic island permafrost, the island thaw zone, and the large continuous permafrost. As the area is in cold temperate zone with continental monsoon, it has dry and windy spring, hot and humid summer, windy and dry autumn, and cold and long winter. During the past 50 years, the temperature in this study area has increased by 0.9–2.2°C, resulting in an obvious northward shift of southern permafrost margin. Currently, the permafrost covers an area of (26–27) × 104 km2, which is (9–10) ×104 km2 (35%–37%) lower than the area in the 1970’s. In this study, typical carex peatlands in Mohe (53°48′N, 122°37′E), Tahe (52°32′N, 124°07′E), and Jiagedaqi (52°20′N, 124°07′E) are selected as the research objects. The three peatlands are located in a large continuous permafrost area, an island thawing permafrost area, and an island permafrost area. The depths of permafrost thawing are 50–60 cm, 60–75 cm and 70–90 cm, respectively (Figure 1).
Sample Collection and Material
In this study, three 1 m × 1 m samples were randomly chosen from the three types of permafrost peatlands. The study was carried out in September 2019, and a 3-cm diameter soil drill was used. Five sampling points (one in each of the four corners and one in the center) were adopted to drill into the deepest place and permafrost active layers. Figures 2A,B shows the vegetation in the selected sample plots, and they are all peatland tower heads. The soil samples were divided into four layers: 0–10 cm, 10–30 cm, 30–50 cm, and below 50 cm. The soil samples in different soil layers were mixed (Figures 2C,D) and removed of visible plant roots and gravel. Then, they were screened by 2 mm and refrigerated at −20°C. The fully mixed samples were to analyze the carbon and nitrogen components, and basic physical and chemical indexes.
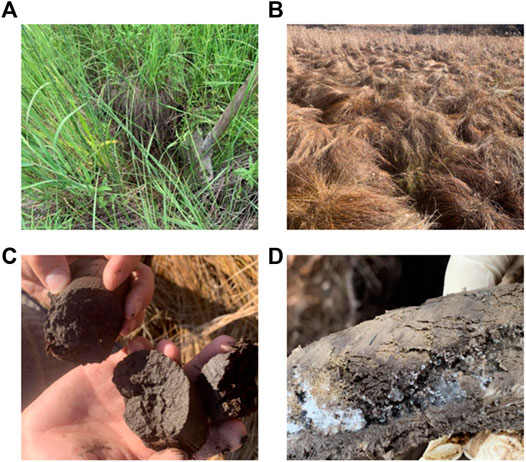
FIGURE 2. Vegetation on peatland (A,B) including tower head, (C,D) at the bottom of the peatland active layer to the permafrost layer.
Soil organic carbon (TOC) was measured with an Multi N/C 3100 analyzer (Analytik Jena, Germany). The soil extracts were shaken, centrifuged and filtered with an N/C 3100 analyzer to measure the dissolved organic carbon (DOC). Microbial biomass carbon (MBC) was determined by the N/C 3100 analyzer after chloroform fumigation. Under the catalyst, the soil was digested with concentrated sulfuric acid at high temperature. After that, the appropriate supernatant was taken, and the total nitrogen (TN) was measured with a SKALAR++ (SKALAR, Netherlands) continuous flow analyzer. After shaking and centrifuging, the treated soil was filtered by adding 2 mol L−1 potassium chloride solution. Based on the KCl extraction-spectrophotometry principle, the SKALAR SAN++ (Netherlands SKALAR) continuous flow analyzer was used to determine the ammonia nitrogen (NH+4-N) and nitrate nitrogen (NO−3-N) in the soil. Moisture was calculated through moisture loss, which was obtained after the samples were dried in the oven at 105° to a constant weight. Soil pH was measured with a pH meter (pb10, Sartorius, China) based on the glass electrode method and the water-soil ratio was set as 1:10. Moreover, the DNA of solid samples on the filter membrane was extracted, and the bacterial abundance was determined by fluorescence quantitative PCR.
Data Methods
Statistical analysis was carried out based on SPSS 24.0, in which the data was denoted as mean ± SE. A one-way ANOVA was conducted for different depths of each index and different types of the frozen soil. At the same time, a two-way ANOVA was performed for the impact of soil depth and frozen soil types on each index, with the significance level of p < 0.01. Based on the method of two-factor variance, the interaction effects of permafrost type and soil depth on carbon and nitrogen were analyzed. Origin 9.0 was employed to display the results.
Results and Analysis
Analysis of Soil Carbon and Nitrogen Variations in the Active Layer
Frozen soil type interacts with soil depth and they exert a statistically significant influence on the dependent variable (F = 65.651, p = 0.000, Partial η2 = 0.995). In other words, soil carbon and nitrogen in different soil layers are affected differently by frozen soil type. According to the multivariate analysis of variance, there is a statistical significance of impacts from frozen soil type and soil layer on the soil carbon and nitrogen (F = 774.976, p = 0.000, partial η2 = 0.995; F = 64.055, p = 0.000, partial η2 = 0.946). According to analysis of the one-way variance main effect, there is statistical significance of impacts from frozen soil type on soil carbon (F = 4069.143, p = 0.000, partial η2 = 0.997). This study also pairwisely compares the soil carbon in the different permafrost types across the four soil layers (Figure 3). For the layers 0–10 cm (A), 10–30 cm (B) and 30–50 cm (C), island permafrost is much larger than the island thawing permafrost and large continuous permafrost areas. Island thawing permafrost is larger than large continuous permafrost areas (Table 1). However, below 50 cm (D), island permafrost is obviously larger than island thaw permafrost (p < 0.01) and large continuous permafrost (p < 0.01) areas, while no significant difference is found between island thaw permafrost and large continuous permafrost (p > 0.05) areas.
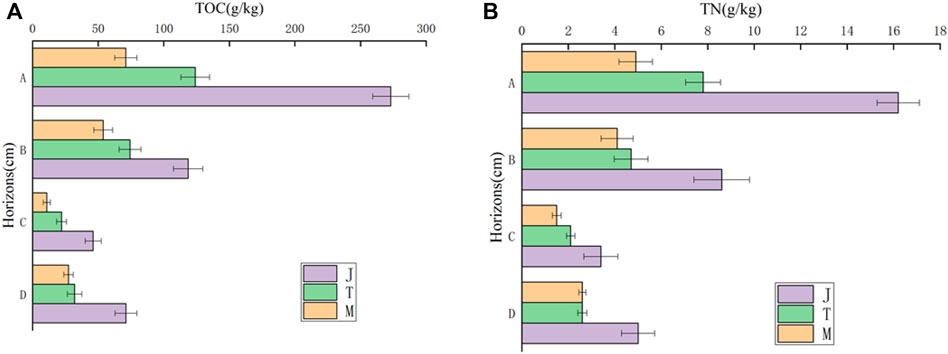
FIGURE 3. The vertical distribution of soil carbon and nitrogen in the peatland of different permafrost regions [(A)- total content of organic carbon, (B)- total nitrogen content].

TABLE 1. Two-factor variance analysis of soil physical and chemical properties on frozen soil type and soil depth (F-value).
According to the one-way analysis of variance, there is a statistical significance on influence of different frozen soil types on soil nitrogen (F = 400.964, p = 0.000, partial η2 = 0.973). This study has also performed a pairwaise comparison of the soil nitrogen in different types of permafrost across the four soil layers. From 0 to 10 cm, island permafrost is significantly larger than the other two types of areas. At the same time, island thawing permafrost is significantly larger than large continuous permafrost areas (Table 1). In layers B, C, and D, island permafrost is significantly higher than island thaw permafrost (p < 0.01) and large continuous permafrost (p < 0.01) areas. However, no significant difference is found between island thaw permafrost, island thaw permafrost and large continuous permafrost (p > 0.05) areas.
Variations in the Active Carbon and Available Nitrogen of the Active Layer
This study has compared soil dissolved organic carbon (F = 655.454, p = 0.000, partial η2 = 0.994) and microbial biomass carbon (F = 1176.656, p = 0.000, partial η2 = 0.997) in the four soil layers of different frozen soil types. Island permafrost has much highly dissolved organic carbon and microbial biomass carbon at 0–10 cm (A) than the large continuous permafrost and island thaw (p < 0.01) areas (Figure 4). For the B, C, and D soil layers, the island permafrost zone has much higher carbon content than the island thaw zone permafrost and large continuous permafrost (p < 0.01) areas.
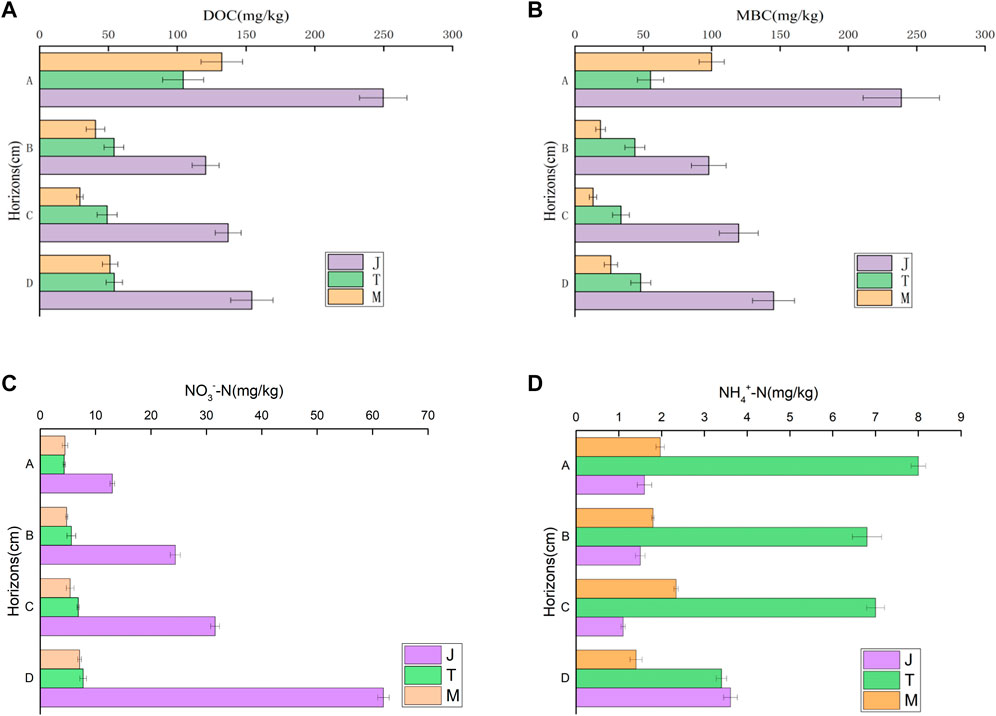
FIGURE 4. Vertical distribution of soil microbial biomass carbon, dissolved organic carbon, ammonia nitrogen and nitrate nitrogen in peatlands of different [Permafrost regions: (A)- Dissolved organic carbon content, (B)- Microbial biomass carbon content, (C)- nitrate nitrogen concentration, (D)- Ammonia nitrogen content].
The paper has compared the content of ammonia nitrogen (F = 88.129, p = 0.000, partial η2 = 0.960) and nitrate nitrogen (F = 1841.332, p = 0.000, partial η2 = 0.998) in four soil layers of different frozen soil types. Across the vertical section, the island permafrost has much higher ammonia nitrogen than the island thaw permafrost (p < 0.01), yet no significant difference is obsereved in the large continuous permafrost area (p > 0.05). At 0–10 cm, 10–30 cm, 30–50 cm and <50 cm soil layers, the nitrate nitrogen content of the island thaw permafrost area is significantly higher than those of the island permafrost and large continuous permafrost (p < 0.001) areas.
Variations in the Microorganisms of the Active Layer
According to the detection results, the soil samples contained over 17 types of fungi, among which the dominant bacteria included acidobacteria, actinobacteria, chlorobacteria, proteobacteria, bacteroidetes, and firmicutes determined (Figure 5). Based on multivariate analysis of variance, there was statistical significance on the influence of the frozen soil type and soil layer to soil bacteria (F = 1.969, p = 0.004, partial η2 = 0.405). This paper pairwisely compared the six dominant phyla in the four soil layers of different permafrost types. At the 0–10 cm layer, acidobacteria levels were significantly greater in the large continuous permafrost areas compared to the island-thaw and island- (p < 0.001) permafrost areas, while no significant difference was found among the different soil layers in the other areas (p > 0.05). At the 0–10 cm and 10–30 cm soil layers, the large continuous permafrost area contained much more actinobacter than the other two areas (p < 0.001), while in the soil layer below 10–50 and 50 cm, the levels in the island permafrost area were obviously higher than the other two areas (p > 0.05). The variations in the vertical profile of firmicutes were similar to those of acidobacteria. Levels in the large continuous permafrost area at 0–10 cm were significantly higher than those in the island permafrost and island thaw (p < 0.001) areas. In the remaining soil layers, the firmicutes in the island strong permafrost area was significantly higher than the other two permafrost areas (p > 0.05). However, there was no significant difference in the four soil layers among different frozen soil types (p > 0.05).
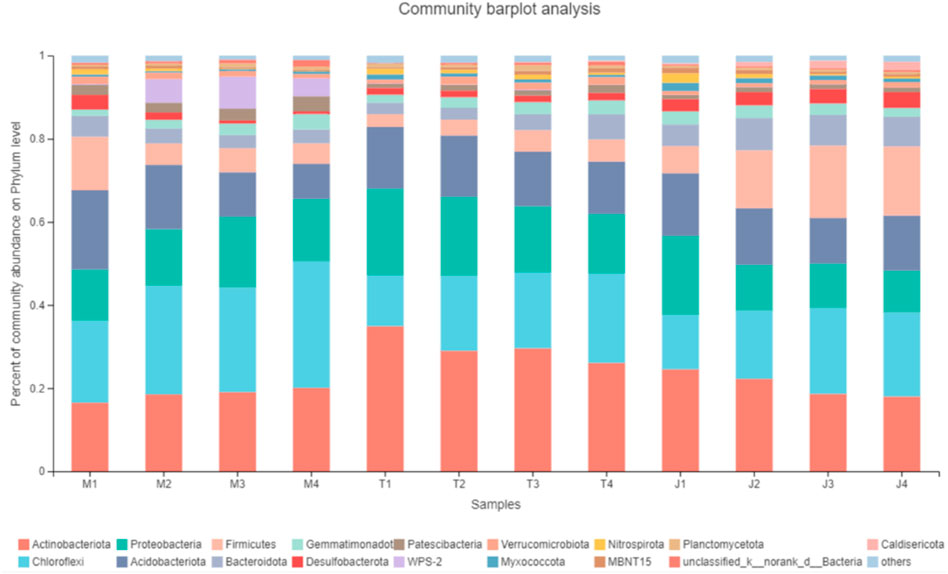
FIGURE 5. Vertical distribution of bacterial species in peatlands across different permafrost regions (M1, M2, M3, and M4 represent the permafrost region of 0–10 cm, 10–30 cm, 30–50 cm and below 50 cm in Mohe, respectively; T1, T2, T3, and T4 represent the permafrost region of 0–10 cm, 10–30 cm, 30–50 cm and below 50 cm in Tahe, respectively; J1, J2, J3, and J4 represent the permafrost region of 0–10 cm, 10–30 cm, 30–50 cm, and below 50 cm in Jiagdaqi, respectively).
Discussion
Accumulation Rate and Organic Carbon and Nitrogen Contents Across Different Frozen Soil Types
Global warming leads to rising temperature, degrading permafrost, and the alternative freezing and thawing of active layers. The type of permafrost and the depth of the active layer will influence the soil carbon and nitrogen in the soil carbon pool. Related studies on soil carbon cycle in active layer of permafrost area have provided useful information, but few research focus on the difference of the carbon and nitrogen distribution in the active layer of peatland in different permafrost areas. Our study demonstrates that permafrost degradation increases the soil carbon and nitrogen in the peatland of the permafrost region, with Mohe < Tahe < Jagdaqi, showing significant differences among regions. Global warming and permafrost degradation have seriously affected the accumulation of soil carbon and nitrogen in the peatlands. As the temperature rises, permafrost degrades and the active layer freezes and thaws alternately. Consequently, more soil organic carbon accumulates in the surface layer than other soil layers due to the type, quantity and decomposition of the overlying vegetation, which decreases vertically.
The soil nitrogen cycle is severely affected by the nitrification and denitrification due to rising temperature, melting permafrost, and soil nitrogen cycling (Yin et al., 2014). Our study finds similar changing pattern of total soil nitrogen and organic carbon in peatlands of permafrost regions, but soil carbon accumulates significantly faster than soil nitrogen. This is because water fills the cold and wet peatland as the permafrost thaws. The production rate of organic carbon is fater than its decomposition rate, thus resulting in a high accumulation rate. The relatively low nitrogen reaction rate may be due to NH4+-N and NO3−—N being the most easily absorbed and utilized forms of nitrogen in the soil (Geng et al., 1999), and the vertical diffusion of the serious influence peatland nitrogen losses (Patrick and Reddy, 1976; Reddy et al., 1980). Dissolved organic carbon and microbial biomass carbon are important components of soil active organic carbon (Powlson et al., 1987; Xu et al., 2010), the key components of which originate from plant litter, root and its exudates, soil organic matter and soil microorganisms (Zhao et al., 2017; Meng et al., 2020). Assuming that these would be a 1–1.5°C temperature rise in the next 40–50 years, the southern permafrost margin will move further northward, and the permafrost area may decrease by another 35% (Jin et al., 2007). Rising temperature will add more organic matter and boost microbial activity, thus increasing the accumulation rate.
As the soil depth increases, the underground biomass sharply decreases and the activity of decomposers weakens. This will slow down the decomposition of plant debris at deeper soil layers (Jobbagy and Jackson, 2002).With lower decomposition rate of organic matter and input of organic nutrients in soil, nitrogen’s enrichment is relatively small. However, long-term anaerobic peatlands are prone to soil REDOX, with a number of active denitrifying bacteria. As a result, the oxidation of ammonia nitrogen accelerates and much amount of nitrate nitrogen is lost from the soil (Yin, 2016). However, higher nitrogen deposition will greatly enhance the amount of active nitrogen, leading to excessive nitrogen in the soil (Li et al., 2021; Li et al., 2021b; Li et al., 2021c). Consequently, the soil nitrogen cycle is affected. As the nitrogen cycle microorganisms respond differently to environmental changes,the coupling relationship and the balance of the nitrogen cycle are both disturbed (Zhang et al., 2008; Fang et al., 2011; Isobe et al., 2012; Levy-Booth et al., 2014).
Studies have shown that rising soil temperature can accelerate the turnover and metabolism of peatland microorganisms. On one hand, higher microbial activity at the surface soil and more mineralization may increase the decomposition of organic matter (Durán et al., 2014) and affect the accumulation of organic carbon. On the other hand, the withered vegetation accumulated in the surface layer may continuously accumulate nutrients, with numerous roots providing abundant carbon for the surface layer. As the soil colloid absorption of the soil nitrate nitrogen (NO3−- N) is complicated, the soil is relatively stable, susceptible to erosion, and the soil nitrogen content under the surface and the other layers is not significant difference according to frozen peat soil types. These conditions may slow down the accumulation rate and degree of soil nitrogen.
Response of Microorganisms in the Active Layer to Soil Organic Carbon and Nitrogen
Numerous studies have demonstrated that the feedback direction between microorganisms (Xiong et al., 2010; Hu et al., 2012) and climate varies according to the region and magnitude of climate change (Durán et al., 2014; Gao et al., 2017; Ren et al., 2017; Xu and Yuan, 2017; Chen et al., 2018). In this study, nitrifying and denitrifying bacteria were the key functional microorganisms involved in the soil carbon and nitrogen cycle (A); proteobacteria were dominant in the metabolism of carbon and nitrogen (A); bacteroidetes were principally involved in the nitrification process (A); chlorocurved promoted the degradation of residues; and firmicutes were key in the iron REDOX process (A). Furthermore, the number of bacteria and their activity varied with different content of soil carbon and nitrogen. Therefore, correctly measuring accumulation rates of the soil carbon and nitrogen is crucial to understand the regional carbon balance. Peatland is a highly climate-sensitive carbon pool, playing a significant role in the global carbon cycle and climate change, and has a potential in controlling carbon emissions. However, once the carbon and nitrogen accumulation exceeds a certain limit, the transformation of organic matter proves to be difficult and the soil texture is extensively damaged, resulting in immeasurable losses. In addition, microorganisms in the soil are an important factor that regulates the carbon and nitrogen cycle (Prosser, 2007). They participate in and directly influence the biogeochemical cycle through substance metabolism (Gao et al., 2010; Ma et al., 2016). According to the experimental results, microorganisms exert a vital effect on the decomposition of organic carbon (Joosten et al., 2003). Based on field monitoring across multiple years, Durán et al. (2014) found that organic carbon have a great effect on the respiration of microorganisms.
Compared with high-temperature permafrost, low-temperature permafrost in the northern permafrost region has much less species and soil microorganisms, and the impacts on soil organic carbon decomposition also show the difference (Chen et al., 2011; Niu et al., 2011; Chen et al., 2012; Chen et al., 2013; Oliva et al., 2018; Chen and Zeng, 2021; Li et al., 2022). This study (Figure 6) demonstrates that the bacterial communities in different types of frozen soil vary with vertical depth. Permafrost degradation greatly affects the ecosystem in cold regions of high latitudes (Li et al., 2014). For example, permafrost thawing and a higher water content in peatlands increase the reproduction of soil microorganisms, promote the conversion of ammonium nitrogen to nitrate nitrogen, and reduce the accumulation of ammonium nitrogen in soil. Shi et al. (2014) have demonstrated that more available nitrogen will increase the soil microbial activity and hasten the decomposition of organic matter, thus accelerating the accumulation of organic carbon. As the accumulation of organic carbon increases with the nitrogen availability, the carbon accumulation will be promoted once again. The microorganisms, carbon and nitrogen in the soil interact with each other. Some bacteria gradually disappear from the large continuous permafrost area to the peatland soil in the island-shaped permafrost area, and the decreased microbial activity may explain why the organic carbon increase in the soil. The study of Baumann et al. (2009) has shown that soils with high organic carbon content are more abundant with microbial, and a suitable soil pH value boosts the metabolism and the reproduction of microorganisms. The unique environmental adaptability of soil microorganisms plays an important role in soil carbon cycling in peatlands of permafrost regions. Soil acidification alters biological activity and affects soil respiration, which in turn affects soil organic carbon accumulation. Therefore, the thawing permafrost, the deepening active layer, less acidity, and the changing bacterial activity, all affect the concentration of organic carbon in large continuous permafrost regions, island-shaped thawed permafrost regions, and peat in island-shaped permafrost regions. The concentration of organic carbon is an important factor in geoaccumulation.
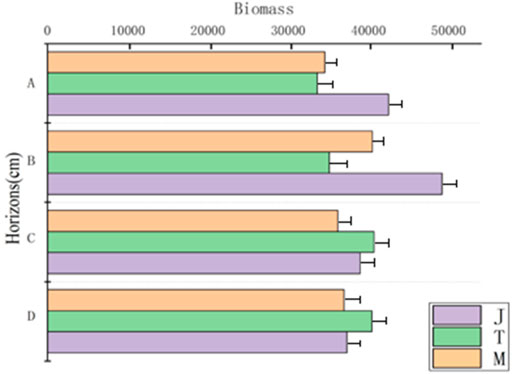
FIGURE 6. Bacterial community composition at the phylum level of different frozen soil types (M1, M2, M3 and M4 represent the permafrost region of 0–10 cm, 10–30 cm, 30–50 cm and below 50 cm in Mohe, respectively; T1, T2, T3, and T4 represent the permafrost region of 0–10 cm, 10–30 cm, 30–50 cm and below 50 cm in Tahe, respectively; J1, J2, J3, and J4 represent the permafrost region of 0–10 cm, 10–30 cm, 30–50 cm, and below 50 cm in Jiagdaqi, respectively).
Conclusion
To conclude, current studies have explored the impact of permafrost degradation on soil carbon and nitrogen in peatlands across permafrost regions. The results have shown that under global warming, permafrost degradation alters the soil environment in peatlands of permafrost regions, increasing the accumulation of carbon and nitrogen in soil. In addition, the accumulation rate of soil organic carbon is faster and the response is greater. This study has revealed the process of how carbon and nitrogen change in peatland soil across the permafrost regions after permafrost degradation. It offers a scientific foundation for more in-depth exploration on the response of alpine ecosystems to the global change and contributes to the regulation of soil quality in the peatlands of permafrost regions.
Data Availability Statement
The original contributions presented in the study are included in the article/supplementary material, further inquiries can be directed to the corresponding author.
Author Contributions
DW and DM contributed to the design of this research. DW, ML, and LW contributed to the methodology of the study. DW and LW performed the data analysis. SZ was responsible for the project management. DW wrote the first draft of the manuscript. All authors contributed to manuscript revision, read, and approved the submitted version.
Conflict of Interest
The authors declare that the research was conducted in the absence of any commercial or financial relationships that could be construed as a potential conflict of interest.
Publisher’s Note
All claims expressed in this article are solely those of the authors and do not necessarily represent those of their affiliated organizations, or those of the publisher, the editors and the reviewers. Any product that may be evaluated in this article, or claim that may be made by its manufacturer, is not guaranteed or endorsed by the publisher.
References
Chen, H., Zhu, Q., Peng, C., Wu, N., Wang, Y., Fang, X., et al. (2013). The Impacts of Climate Change and Human Activities on Biogeochemical Cycles on the Qinghai-Tibetan Plateau. Glob. Change Biol. 19 (10), 2940–2955. doi:10.1111/gcb.12277
Chen, P., and Zeng, Q. (2021). Geochronology, Geochemistry and Tectonic Significance of Buziwannan Late Triassic I-Type Granites in the West Kunlun Orogen Belt, Northwest China. Petrology 29 (5), 575–596. doi:10.1134/S0869591121050052
Chen, S., Liu, W., Qin, X., Liu, Y., Zhang, T., Chen, K., et al. (2012). Response Characteristics of Vegetation and Soil Environment to Permafrost Degradation in the Upstream Regions of the Shule River Basin. Environ. Res. Lett. 7 (4), 045406. doi:10.1088/1748-9326/7/4/045406
Chen, S. Y., Liu, W. J., Ye, B. S., Yang, G. J., Yi, S. H., Wang, F. G., et al. (2011). Species Diversity of Vegetation in Relation to Biomass and Environmental Factors in the Upper Area of the Shule River. Acta Prataculturae Sin. 20 (3), 70–83. doi:10.1007/BF02026812
Chen, S. Y., Zhao, Q., Liu, W. J., Zhang, Z., Li, S., Li, H. L., et al. (2018). Effects of Freeze–Thaw Cycles on Soil N2O Concentration and Flux in the Permafrost Regions of the Qinghai–Tibetan Plateau. Sci. Cold Arid Regions (English Version) 10 (1), 69–79. doi:10.3724/SP.J.1226.2018.00069
Cox, P. M., Betts, R. A., Jones, C. D., Spall, S. A., and Totterdell, I. J. (2000). Acceleration of Global Warming Due to Carbon-Cycle Feedbacks in a Coupled Climate Model. Nature 408 (6809), 184–187. doi:10.1038/35041539
Davidson, E. A., Trumbore, S. E., and Amundson, R. (2000). Soil Warming and Organic Carbon Content. Nature 408 (6814), 789–790. doi:10.1038/35048672
Dorrepaal, E., Toet, S., van Logtestijn, R. S. P., Swart, E., van de Weg, M. J., Callaghan, T. V., et al. (2009). Carbon Respiration from Subsurface Peat Accelerated by Climate Warming in the Subarctic. Nature 460, 616–619. doi:10.1038/nature08216
Durán, J., Morse, J. L., Groffman, P. M., Campbell, J. L., Christenson, L. M., Driscoll, C. T., et al. (2014). Winter Climate Change Affects Growing-Season Soil Microbial Biomass and Activity in Northern Hardwood Forests. Glob. Change Biol. 20 (11), 3568–3577. doi:10.1111/gcb.12624
Fang, Y., Yoh, M., Koba, K., Zhu, W., Takebayashi, Y., Xiao, Y., et al. (2011). Nitrogen Deposition and Forest Nitrogen Cycling along an Urban-Rural Transect in Southern China. Glob. Change Biol. 17 (2), 872–885. doi:10.1111/j.1365-2486.2010.02283.x
Gao, J. Q., Lei, G. C., Li, L., Lv, S., and Bai, M. Y. (2010). The Distribution Characteristics of Soil Organic Carbon in Three Kinds of Wetland Soils in Zoige Plateau. Wetl. Sci. 8 (4), 327–330. doi:10.13248/j.cnki.wetlandsci.2010.04.014
Gao, J. Q., Ou, Y. H., Zhang, F., and Wang, C. M. (2008). The Response of Soil Nitrogen Mineralization to Soil Temperature and Soil Moisture in Zoige Alpine Wetland. Wetl. Sci. 6 (2), 229–234. doi:10.1016/S1872-2075(08)60071-0
Gao, J. Q., Ouyang, H., Zhang, F., and Wang, C. M. (2007). Spatial Distribution of Surface Soil Organic Carbon in Zoige Alpine Wetland. Ecol. Environ. 144 (6), 1723–1727. doi:10.1016/j.catena.2016.05.014
Gao, J., Wang, E., Ren, W., Liu, X., Chen, Y., Shi, Y., et al. (2017). Effects of Simulated Climate Change on Soil Microbial Biomass and Enzyme Activities in Young Chinese Fir ( Cunninghamia Lanceolata ) in Subtropical China. Acta Ecol. Sin. 37 (4), 272–278. doi:10.1016/j.chnaes.2017.02.007
Geng, Y. Q., Sun, X. Y., Kang, X. G., Tan, X., Zhou, Y. Q., Li, D. L., et al. (1999). Soil Fertility under Different Forest Types in Changbai Mountain. J. Beijing For. Univ. 21 (6), 97–101. doi:10.13332/j.1000-1522.1999.06.017
Gorham, E. (1991). Northern Peatlands: Role in the Carbon Cycle and Probable Responses to Climatic Warming. Ecol. Appl. 1 (2), 182–195. doi:10.2307/1941811
Guntiñas, M. E., Leirós, M. C., Trasar-Cepeda, C., and Gil-Sotres, F. (2012). Effects of Moisture and Temperature on Net Soil Nitrogen Mineralization: A Laboratory Study. Eur. J. Soil Biol. 48, 73–80. doi:10.1016/j.ejsobi.2011.07.015
He, G., Liu, X., and Cui, Z. (2021). Achieving Global Food Security by Focusing on Nitrogen Efficiency Potentials and Local Production. Glob. Food Secur. 29, 100536. doi:10.1016/j.gfs.2021.100536
Hu, X., Wu, N., Wang, Q., and Wu, Y. (2012). Effects of Snowpack and Litter Input on Soil Nitrogen Dynamics in the Eastern Tibetan Plateau. Ecol. Environ. Sci. 21 (11), 1789–1794. doi:10.16258/j.cnki.1674-5906.2012.11.004
Isobe, K., Koba, K., Suwa, Y., Ikutani, J., Fang, Y., Yoh, M., et al. (2012). High Abundance of Ammonia-Oxidizing Archaea in Acidified Subtropical Forest Soils in Southern China after Long-Term N Deposition. FEMS Microbiol. Ecol. 80 (1), 193–203. doi:10.1111/j.1574-6941.2011.01294.x
Jin, H., Yu, Q., Lü, L., Guo, D., He, R., Yu, S., et al. (2007). Degradation of Permafrost in the Xing'anling Mountains, Northeastern China. Permafr. Periglac. Process. 18 (3), 245–258. doi:10.1002/ppp.589
Jobbagy, E. G., and Jackson, R. B. (2002). The Vertical Distribution of Soil Organic Carbon and It’s Relation to Climate and Vegetation. Ecol. Appl. 10 (2), 423–636. doi:10.1890/1051-0761(2000)010[0423:TVDOSO]2.0.CO;2
Joosten, R., Schumacher, J., Wirth, C., and Schulte, A. (2003). Evaluating Tree Carbon Predictions for Beech (Fagus Sylvatica L.) in Western Germany. For. Ecol. Manag. 189 (1-3), 87–96. doi:10.1016/j.foreco.2003.07.037
Kaiser, K., and Benner, R. (2009). Biochemical Composition and Size Distribution of Organic Matter at the Pacific and Atlantic Time-Series Stations. Mar. Chem. 113 (1-2), 63–77. doi:10.1016/j.marchem.2008.12.004
Levy-Booth, D. J., Prescott, C. E., and Grayston, S. J. (2014). Microbial Functional Genes Involved in Nitrogen Fixation, Nitrification and Denitrification in Forest Ecosystems. Soil Biol. Biochem. 75, 11–25. doi:10.1016/j.soilbio.2014.03.021
Li, J., Charles, L. S., Yang, Z., Du, G., and Fu, S. (2022). Differential Mechanisms Drive Species Loss under Artificial Shade and Fertilization in the Alpine Meadow of the Tibetan Plateau. Front. Plant Sci. 13, 832473. doi:10.3389/fpls.2022.832473
Li, R., Wu, Q., Li, X., Sheng, Y., Hu, G., Cheng, G., et al. (2019). Characteristic, Changes and Impacts of Permafrost on Qinghai-Tibet Plateau. Chin. Sci. Bull. 64 (27), 2783–2795. doi:10.1360/TB-2019-0191
Li, W., Shi, Y., Zhu, D., Wang, W., Liu, H., Li, J., et al. (2021a). Fine Root Biomass and Morphology in a Temperate Forest Are Influenced More by the Nitrogen Treatment Approach Than the Rate. Ecol. Indic. 130, 108031. doi:10.1016/j.ecolind.2021.108031
Li, X., Zhang, C., Zhang, B., Wu, D., Shi, Y., Zhang, W., et al. (2021b). Canopy and Understory Nitrogen Addition Have Different Effects on Fine Root Dynamics in a Temperate Forest: Implications for Soil Carbon Storage. New Phytol. 231 (4), 1377–1386. doi:10.1111/nph.17460
Li, X., Zhang, C., Zhang, B., Wu, D., Zhu, D., Zhang, W., et al. (2021c). Nitrogen Deposition and Increased Precipitation Interact to Affect Fine Root Production and Biomass in a Temperate Forest: Implications for Carbon Cycling. Sci. Total Environ. 765, 144497. doi:10.1016/j.scitotenv.2020.144497
Li, Y., Yuan, X., and Zhu, H. (2014). Simulation Study on Soil Moisture Contents on Nitrogen Transformation and Enzyme Activities in Black Soil. Chin. J. Soil Sci. 45 (4), 903–908. doi:10.1061/41052(346)64
Luo, D. (2014). Nitrogen and Nitrogen Characteristics of Young Pure and Mixed Stands of Trellis and Masson Pine in Subtropical Region. PhD Thesis. Chinese Academy of Forestry Sciences. Retrieved from https://d.wanfangdata.com.cn/thesis/Y2630021.
Ma, K., Zhang, Y., Tang, S., and Liu, J. (2016). Spatial Distribution of Soil Organic Carbon in the Zoige Alpine Wetland, Northeastern Qinghai-Tibet Plateau. Catena 144, 102–108. doi:10.1016/j.catena.2016.05.014
Mack, M. C., Schuur, E. A. G., Bret-Harte, M. S., Shaver, G. R., and Chapin, F. S. (2004). Ecosystem Carbon Storage in Arctic Tundra Reduced by Long-Term Nutrient Fertilization. Nature 431 (7007), 440–443. doi:10.1038/nature02887
Meng, T. G., Wu, L. Y., Zhang, S. L., Xu, Y. Y., Li, X., and Zhang, J. G. (2020). Vertical Distribution of Soil Dissolved Carbon and its Influencing Factors in the Artificial Shelterbelt Irrigated with Saline Water in an Extreme Drought Desert. Environ. Sci. 41 (4), 1950–1959. doi:10.13227/j.hjkx.201909003
Mu, C., Zhang, T., Wu, Q., Cao, B., Zhang, X., Peng, X., et al. (2015). Carbon and Nitrogen Properties of Permafrost over the Eboling Mountain in the Upper Reach of Heihe River Basin, Northwestern China. Arct. Antarct. Alp. Res. 47 (2), 203–211. doi:10.1657/AAAR00C-13-095
Niu, L., Ye, B., Li, J., and Sheng, Y. (2011). Effect of Permafrost Degradation on Hydrological Processes in Typical Basins with Various Permafrost Coverage in Western China. Sci. China Earth Sci. 54, 615–624. doi:10.1007/s11430-010-4073-1
Oliva, M., Pereira, P., and Antoniades, D. (2018). The Environmental Consequences of Permafrost Degradation in a Changing Climate. Sci. Total Environ. 616-617, 435–437. doi:10.1016/j.scitotenv.2017.10.285
Patrick, W. H., and Reddy, K. R. (1976). Nitrification‐Denitrification Reactions in Flooded Soils and Water Bottoms: Dependence on Oxygen Supply and Ammonium Diffusion. J. Environ. Qual. 5, 469–472. doi:10.2134/jeq1976.00472425000500040032x
Pizano, C., Barón, A. F., Schuur, E. A. G., Crummer, K. G., and Mack, M. C. (2014). Effects of Thermo-Erosional Disturbance on Surface Soil Carbon and Nitrogen Dynamics in Upland Arctic Tundra. Environ. Res. Lett. 9 (7), 075006. doi:10.1088/1748-9326/9/7/075006
Powlson, D. S., Prookes, P. C., and Christensen, B. T. (1987). Measurement of Soil Microbial Biomass Provides an Early Indication of Changes in Total Soil Organic Matter Due to Straw Incorporation. Soil Biol. Biochem. 19 (2), 159–164. doi:10.1016/0038-0717(87)90076-9
Prosser, J. I. (2007). “Microorganisms Cycling Soil Nutrients and Their Diversity,” in Books in Soils, Plants, and the Environment. Editors J. D. van Elsas, J. K. Jansson, and J. T. Trevors. 2nd Edition (Boca Raton, Florida, U.S.: CRC Press), 237–261.
Reddy, K. R., Patrick, W. H., and Phillips, R. E. (1980). Evaluation of Selected Processes Controlling Nitrogen Loss in a Flooded Soil. Soil Sci. Soc. Am. J. 44, 1241–1246. doi:10.2136/sssaj1980.03615995004400060022x
Reinmann, A. B., Susser, J. R., DeMaria, E. M. C., and Templer, P. H. (2019). Declines in Northern Forest Tree Growth Following Snowpack Decline and Soil Freezing. Glob. Change Biol. 25 (2), 420–430. doi:10.1111/gcb.14420
Ren, C., Zhao, F., Shi, Z., Chen, J., Han, X., Yang, G., et al. (2017). Differential Responses of Soil Microbial Biomass and Carbon-Degrading Enzyme Activities to Altered Precipitation. Soil Biol. Biochem. 115, 1–10. doi:10.1016/j.soilbio.2017.08.002
Schuur, E. A. G., and Abbott, B. (2011). Climate Change: High Risk of Permafrost Thaw. Nature 480, 32–33. doi:10.1038/480032a
Schuur, E. A. G., McGuire, A. D., Schädel, C., Grosse, G., Harden, J. W., Hayes, D. J., et al. (2015). Climate Change and the Permafrost Carbon Feedback. Nature 520, 171–179. doi:10.1038/nature14338
Shi, Y., Wang, Z. Q., Zhang, X. Y., Sun, X. M., Liu, X. Y., He, N. P., et al. (2014). Effects of Nitrogen and Phosphorus Addition on Soil Microbial Community Composition in Temperate Typical Grassland in Inner Mongolia. Acta Eco Sin. 34 (17), 4943–4949. doi:10.5846/stxb201306081430
Shibata, H. (2016). Impact of Winter Climate Change on Nitrogen Biogeochemistry in Forest Ecosystems: A Synthesis from Japanese Case Studies. Ecol. Indic. 65, 4–9. doi:10.1016/j.ecolind.2015.10.063
Sierra, C. A., Trumbore, S. E., Davidson, E. A., Vicca, S., and Janssens, I. (2015). Sensitivity of Decomposition Rates of Soil Organic Matter with Respect to Simultaneous Changes in Temperature and Moisture. J. Adv. Model. Earth Syst. 7, 335–356. doi:10.1002/2014MS000358
Sierra, J. (1997). Temperature and Soil Moisture Dependence of N Mineralization in Intact Soil Cores. Soil Biol. Biochem. 29 (9-10), 1557–1563. doi:10.1016/S0038-0717(96)00288-X
IPCC (2007). “Changes in Atmospheric Constituents and in Radiative Forcing,” in Climate Change 2007: The Physical Science Basis, Contribution of Working Group I to the Fouth Assessment Report of the Intergovermental Panel on Climate Change. Editors S. D. Q Solomon, M. Manning, Z. Chen, M. Marquis, K. B. Averyt, M. Tignoret al. (Cambridge, United Kingdom and New York, NY, USA: Cambridge University Press).
Song, C. C., Song, Y. Y., Wang, X. W., Guo, Y. D., Sun, L., and Zhang, X. H. (2018). Research Progress of Carbon and Nitrogen Cycles in Wetland Ecosystem under Climate Change. Wetl. Sci. 16 (3), 424–431. doi:10.13248/j.cnki.wetlandsci.2018.03.020
Turner, M. M., and Henry, H. A. L. (2010). Net Nitrogen Mineralization and Leaching in Response to Warming and Nitrogen Deposition in a Temperate Old Field: The Importance of Winter Temperature. Oecologia 162 (1), 227–236. doi:10.1007/s00442-009-1435-5
Wu, X. D., and Wu, T. H. (2020). Permafrost Degradation Has Important Effects on Climate and Human Society. Chin. J. Nat. 42 (5), 425–431. doi:10.3969/j.issn.0253-9608.2020.05.011
Xiong, P., Xu, Z. F., Lin, B., and Liu, Q. (2010). Short-Term Response of Winter Soil Respiration to Simulated Warming in a Pinus Armandii Plantation in the Upper Reaches of the Minjiang River, China. Chin. J. Plant Ecol. 34 (12), 1369–1376. doi:10.3773/j.issn.1005-264x.2010.12.002
Xu, J., Lan, W., Ren, C., Zhou, X., Wang, S., and Yuan, J. (2021). Modeling of Coupled Transfer of Water, Heat and Solute in Saline Loess Considering Sodium Sulfate Crystallization. Cold Regions Sci. Technol. 189, 103335. doi:10.1016/j.coldregions.2021.103335
Xu, W., and Yuan, W. (2017). Responses of Microbial Biomass Carbon and Nitrogen to Experimental Warming: A Meta-Analysis. Soil Biol. Biochem. 115, 265–274. doi:10.1016/j.soilbio.2017.08.033
Xu, X., Cheng, X. Y., Zhou, Y., Luo, Y. Q., Ruan, H. H., and Wang, J. (2010). Variation of Soil Labile Organic Carbon Pools along an Elevational Gradient in the Wuyi Mountains, China. J. Resour. Ecol. 1 (4), 368–374. doi:10.3969/j.issn.1674-764x.2010.04.010
Yan, J. X., Qin, Z. D., Zhang, Y. H., and Li, H. J. (2009). Effects of Soil Moisture and Moisture on Soil Respiration in Chinese Pine Forest. Acta Ecol. Sin. 29 (12), 6366–6376. doi:10.3321/j.issn:1000-0933.2009.12.007
Yang, J. M., Jiang, X., Zhang, Z. Q., Liu, J. H., and Gao, Q. (2014). Study on Nitrification, Denitrification and Respiration in Baps System. J. Jilin Agric. Univ. 1 (1), 71–76. doi:10.13327/j.jjlau.2014.1556
Yang, Y., Dou, Y., Wang, B., Wang, Y., Liang, C., An, S., et al. (2022). Increasing Contribution of Microbial Residues to Soil Organic Carbon in Grassland Restoration Chronosequence. Soil Biol. Biochem. 170, 108688. doi:10.1016/j.soilbio.2022.108688
Yang, Y., Li, T., Wang, Y., Cheng, H., Chang, S. X., Liang, C., et al. (2021). Negative Effects of Multiple Global Change Factors on Soil Microbial Diversity. Soil Biol. Biochem. 156, 108229. doi:10.1016/j.soilbio.2021.108229
Yin, R., Xu, Z. F., Wu, F. Z., Yang, W. Q., Xiong, L., Zhang, X. T., et al. (2014). Effects of Snow Pack on Winter Soil Labile Nitrogen Pools and Nitrogen Mineralization Potential in an Subalpine Abies Faxoniana Forest of Western Sichuan. Chin. J. Appl. Environ. Biol. 20 (1), 15–21. doi:10.3724/SP.J.1145.2014.00015
Yin, S. Y. (2016). The Progress of Study on N2O Emissions in Cropland. Green Sci. Technol. 58 (12), 152–154. doi:10.16663/j.cnki.lskj.2016.12.058
Zhang, K. (2011). Effects of Alien Bobbinopsis on Carbon and Nitrogen Cycling in Soil. Master’s Thesis. Institutes of Technology of Henan. Retrieved from https://d.wanfangdata.com.cn/thesis/D567515.
Zhang, Q. (2017). The Effects of CH4 Fluxes on Forests in the Nanling Area. Master’s Thesis. Central South University of Forestry and Technology. Retrieved from http://cdmd.cnki.com.cn/Article/CDMD-10538-1017118134.htm.
Zhang, W., Mo, J. M., Fang, Y. T., Li, D. J., Lu, X. K., and Wang, H. E. (2008). Anisotrophic Effects of Simulated Nitrogen Deposition on Tropical Forests in Southern China. Soil Sci. Technol. 306 (1-2), 221–236. doi:10.1007/s11104-008-9575-7
Zhao, G. Y., Guo, D. N., Jiang, S., and Shao, Z. (2017). Effects of Freezing and Thawing on Soil Active Organic Carbon in the Xiaoxing’an Mountain Wetlands. Acta Ecol. Sin. 37 (16), 5411–5417. doi:10.5846/stxb201605170953
Zhao, X., Xia, H., Pan, L., Song, H., Niu, W., Wang, R., et al. (2021). Drought Monitoring over Yellow River Basin from 2003-2019 Using Reconstructed MODIS Land Surface Temperature in Google Earth Engine. Remote Sens. 13 (18), 3748. doi:10.3390/rs13183748
Zhou, W. C., Solang, T., Cui, L. J., Wang, Y. J., and Li, W. (2016). Effects of Soil Organic Carbon Storage System for Soil in Peatland, Northwest China. Chin. J. Ecol. 36 (8), 2123–2132. doi:10.5846/stxb201411022148
Zimov, S. A., Schuur, E. A. G., and Chapin, F. S. (2006). Permafrost and the Global Carbon Budget. Science 312 (5780), 1612–1613. doi:10.1126/science.1128908
Keywords: global warming, permafrost, peatland, soil carbon, soil nitrogen
Citation: Wang D, Zang S, Wang L, Ma D and Li M (2022) Effects of Permafrost Degradation on Soil Carbon and Nitrogen Cycling in Permafrost Wetlands. Front. Earth Sci. 10:911314. doi: 10.3389/feart.2022.911314
Received: 02 April 2022; Accepted: 07 June 2022;
Published: 27 June 2022.
Edited by:
Lu-Jun Li, Chinese Academy of Sciences (CAS), ChinaReviewed by:
Wei Shan, Northeast Forestry University, ChinaNa Li, Chinese Academy of Sciences (CAS), China
Copyright © 2022 Wang, Zang, Wang, Ma and Li. This is an open-access article distributed under the terms of the Creative Commons Attribution License (CC BY). The use, distribution or reproduction in other forums is permitted, provided the original author(s) and the copyright owner(s) are credited and that the original publication in this journal is cited, in accordance with accepted academic practice. No use, distribution or reproduction is permitted which does not comply with these terms.
*Correspondence: Shuying Zang, enN5NjMxMUBocmJudS5lZHUuY29t