Evaluation of BER for the EHF Communication System Serving Sharp-Coned Reentry Vehicles
- 1School of Resources and Environment, Nanchang University, Nanchang, China
- 2School of Information Engineering, Nanchang University, Nanchang, China
“Communication blackout” could lead to severe risks to reentry vehicles. Previous studies have shown that EHF (extremely high frequency) communication is a potential solution to the “communications blackout” and was mostly used for blunt-coned vehicles. EHF communication with sharp-coned vehicles was rarely concerned before. In the present study, the propagation characteristics of the modulated EHF signals in a time-varying plasma sheath covering sharp-coned vehicles were studied numerically. The plasma sheath was obtained by solving a hypersonic fluid dynamics model. The signals propagation model is developed based on geometric optical theories. The carrier frequencies concerned in the present study are the “atmospheric window” frequencies, which are 94, 140, and 225 GHz, respectively. Based on the signal propagation characteristics, the EHF communication system was modeled. The modulation modes concerned in the present study are the 2ASK, 2PSK, and 2FSK, respectively. The results show that 1) the transmission coefficient of EHF signals in the side channel of the plasma sheath of the sharp-coned reentry vehicle increases with carrier frequency, 2) the gap between different channels at specific carrier frequency decreases with time, 3) the phase shift tends to be stable as the carrier frequency increases, and 4) the bit error rate (BER) varies with time and is affected by the location of the onboard antenna, carrier frequency, and modulation mode. The study shows that, in order to achieve good BER performance for the EHF communication system, 2PSK modulation of the signal at 140 GHz is recommended, and the onboard antenna is suggested to be installed on the wall and close to the bottom of the sharp-coned vehicle.
1 Introduction
Due to aerodynamic heating, the neutral gas surrounding the hypersonic vehicle in near space could be ionized. As a result, a weakly ionized gas layer called plasma sheath is formed. The plasma sheath covers the entire vehicle. The plasma sheath absorbs, reflects, and scatters the communication signals, and eventually, the communication is interrupted (Gillman et al., 2010; Starkey 2015). The attenuation of the signals in the plasma sheath decreases rapidly as the carrier frequency increases (Starkey, 2003; Yuan et al., 2018a). The electron density in the plasma sheath can reach 1020 m−3, and the corresponding cut-off frequency is 89 GHz. Electromagnetic (EM) waves with frequencies higher than 89 GHz can penetrate the plasma sheath. The extremely high-frequency (EHF) band is from 30 to 300 GHz. Therefore, it is feasible to use the EHF-band communication system to effectively mitigate the communication “blackout.”
Many scholars have studied the propagation characteristics of EHF signals in the plasma sheath of a reentry vehicle. Tang et al. (2019) studied the relationship between the attenuation of the EHF signal in plasma sheath and the speed of the vehicle. The study by Rao et al. (2021) found that the reflectivity and transmittance of EHF waves are affected by the frequency of the incident wave, the electron density of the plasma, and the radius of the colliding particles, etc. Zhao et al. (2022a) found that the gain, lobe width, and lobe direction of 0.14 THz phased array antenna is affected by the weakly ionized plasma of the reentry vehicle. Yang et al. (2022a) found that the attenuation of the EHF signals decreases and the phase shift fluctuates more severely with an increase in the angle between the tail channel and the tail of the vehicle.
On the other hand, Wei et al. (2019) pointed out that the BER changes with the evolution of the plasma sheath. In Wei’s work, the plasma parameters measured in the RAMC-II reentry experiment are used to study their effect on the BER of the evolved plasma sheath. The relevant frequencies are 1.575, 2.3, 4.0, 8.4, 32, and 100 GHz. According to this study, NC-MFSK (M ≥ 8) modulation is recommended for communication systems serving reentry vehicles. Liu et al. (2020) studied the influence of dynamic plasma sheath on the BER of two typical modulation signals of BPSK and NC-MFSK under the action of the external magnetic field. The results show that the NC-8FSK has a better performance than BPSK, followed by NC-4FSK and NC-2FSK. According to the study by Yang et al. (2022b), the suggested modulation modes are 2PSK and 4QAM at the carrier frequency of 140 GHz, which could lead to smaller and more stable BER for the EHF communication system utilized by reentry vehicles.
Although there are many studies on the propagation of EHF waves in the plasma sheath of a blunt-coned reentry vehicle, the plasma sheath covering the sharp-coned reentry vehicle is rarely studied. The sharp-coned and blunt-coned reentry vehicles represent ballistic missiles and reentry capsules respectively. The blunt-coned reentry vehicle has been studied for a long time, and its channel characteristics have been relatively clear. Tang et al. (2021) studied the electron density, collision frequency of different channels on the side of the plasma sheath of the sharp-coned reentry vehicle and the propagation characteristics of EHF wave in the plasma sheath of the sharp-coned reentry vehicle, analyzed and compared its characteristics with that of plasma sheath of a blunt-coned reentry vehicle, analyzed the mechanism of wave energy loss, found that the signal attenuation mechanism of sharp-coned reentry vehicle is different from that of a blunt-coned reentry vehicle. Compared with the effect of a sharp-coned plasma sheath on a 0.14 THz array antenna, the blunt-coned plasma sheath refracts the radiation direction of the antenna, resulting in the deterioration of communication quality in the expected direction (Zhao et al., 2022b). The difference in attenuation mechanism between sharp-coned and blunt-coned reentry vehicles will inevitably lead to their different communication performance. However, the influence of plasma sheath on carrier phase shift and the evolution characteristics of plasma sheath are not considered in the literature on sharp-coned reentry vehicles. Without external disturbance, the plasma sheath has natural evolution characteristics, so the channel conditions will inevitably change with time, and then the performance of the communication system will also change with time. Therefore, the time-varying characteristics of EHF channels in the plasma sheath of sharp-coned reentry vehicles is an unavoidable problem in the design of a reentry communication system for ballistic missiles.
All signals propagating in the plasma sheath are modulated. The quality of communication performance is not only related to signal attenuation, but also to phase shift influenced by the plasma sheath (Shi et al., 2012). The plasma sheath of the reentry vehicle is time-varying (He et al., 2014). The time scale of plasma sheath change can be less than 1 ms (Yuan et al., 2018b). The carrier period is much smaller than the time scale of plasma sheath evolution when the communication system works in the EHF band. Therefore, it is worth studying how the BER changes with time without external disturbance. In the reference “Yang et al. (2022b),” the flight object is a blunt-coned vehicle, yet in the present work, the object is a sharp-coned vehicle. Previous work has revealed that the structures of blunt-coned plasma sheaths and sharp-coned plasma sheaths are quite different. Also, the mechanics of EHF signal attenuations are different in the two types of plasma sheaths. As the result, the SNR for the received signals differs. According to our previous works, the BER performance significantly depends on the SNR. Thus, the BER performances for identical modulation modes in sharp-coned plasma sheath are different from that in blunt-coned plasma sheath. In addition, the plasma sheath is inhomogeneous (Ouyang et al., 2020). Therefore, the location of the onboard antenna will make an important contribution to the conditions of the EHF communication channel in the plasma sheath.
The present study mainly focused on the BER of the EHF communication system serving sharp-coned reentry vehicles. The evolution of the plasma sheath was solved with a numerical hypersonic hydrodynamic model. The whole evolution cycle is 1 ms. The typical modulation modes concerned in the present study were 2ASK, 2PSK, and NC-2FSK. The carrier frequencies involved are “atmospheric windows” of 94, 140, and 225 GHz in the EHF band, respectively. The influence on the BER of EHF signals of antenna location, carrier frequency, and modulation method in the plasma sheath of a sharp-coned reentry vehicle will be investigated.
2 Propagation Characteristics of Extremely High-Frequency Waves in the Sharp-Coned Plasma Sheath
In order to study the propagation characteristics of EHF waves in the plasma sheath of a sharp-coned reentry vehicle, the plasma sheath was modeled based on fluid theory. The evolution of the plasma sheath in 1 ms was simulated under the conditions of specific atmospheric temperature, atmospheric mass density, flight speed, and angle of attack (AOA). The model used here is the same as the literature (Tang et al., 2021). The length and the bottom size of the sharp-coned are 1.295 and 0.6667 m, respectively. The angle of attack is 0°. The atmospheric mass density was 1.65 × 10–1 kg/m3, which corresponds to the atmospheric mass density at the altitude of 30 km in middle latitudes. The temperature was 215 K. The flight speed of the vehicle is 6,550 m/s (Yuan et al., 2017). A hydrodynamic model was employed to simulate the plasma sheaths.
Figure 1 shows the electron density distribution of the plasma sheath of the sharp-coned reentry vehicle and the maximum electron density curves of the selected six channels at t = 1 ms. The white short lines in Figure 1A indicate the side channels to be analyzed, which are marked as 1, 2, 3, 4, 5, and 6. The ends of the channels close to the vehicle are the locations of the antennas. The abscissas of the six antenna locations are 0.6, 0.7, 0.8, 0.9, 1, and 1.1 m, respectively. The color axis represents the electron density. Figure 1B shows the maximum electron density of the six channels in Figure 1A at 1 ms. It can be seen from the figure that the maximum electron densities of different channels are different at a specific time. The electron density of channel 3 is the smallest and that of channel 6 is the largest, which reflects the spatial heterogeneity of the plasma sheath of the sharp-coned reentry vehicle.
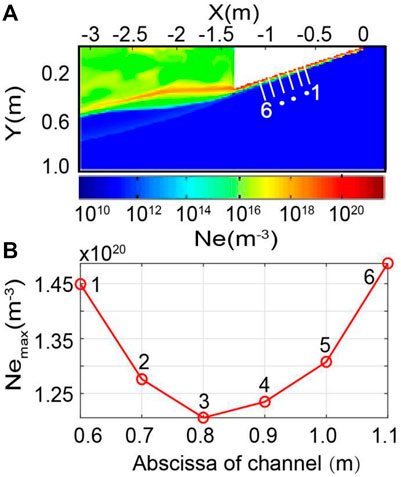
FIGURE 1. (A) Schematic diagram of side channels of plasma sheath of a sharp-coned reentry vehicle, (B) Maximum electron density of each channel at t = 1 ms.
The wavelength of the EHF wave is smaller than the spatial scale of inhomogeneity in the plasma sheath. Therefore, the propagation of the EHF wave in the plasma sheath can be studied as the propagation of the EM wave in one-dimensional inhomogeneous collisional plasma. In this article, the propagation of EHF waves in plasma sheath was studied by using the one-dimensional scattering matrix method (SMM). The SMM method is a common calculation method for geometrical optics analysis in the frequency domain. It deduces the propagation and reflection coefficients based on the wave impedance of each layer of medium. The reflection term caused by impedance jump is also considered. Therefore, it is suitable for the medium with drastic changes in EM characteristic gradient and the approximate calculation of radio wave propagation in inhomogeneous plasma sheath.
In this article, it is assumed that the signal is vertically incident. The plasma slab is divided into n thin layers, as shown in Figure 2. Each thin layer is regarded as a homogeneous layer. The wave travels from free space (neutral atmosphere in this model) along a certain path to the plasma sheath until it reaches the antenna on the surface of the vehicle. In Figure 2, the region I is the free space corresponding to the blue area in Figure 1A, region II is the plasma slab corresponding to the part of the plasma sheath in the selected channel in Figure 1A, and Region III is the free space where the antenna is located corresponding to the onboard antenna of the vehicle in Figure 1A. The scattering matrix is calculated as the same as that in the literature (Hu et al., 1999). The transmission coefficient and phase shift of different channels at different times are calculated by the SMM method, and finally, time-varying curves of the transmission coefficient and phase shift of different channels are obtained.
Figure 3 illustrates the transmission coefficient and phase shift. Panels A, B, and C show signal attenuation for carrier frequencies of 94, 140, and 225 GHz, respectively. The evolution of the plasma sheath of the sharp-coned reentry vehicle under certain conditions starts from the plasma generation on the top of the vehicle until it envelops the entire vehicle. The simulation lasts for 1 ms. If it continues to evolve, the overall plasma sheath changes little. The time on the abscissa of Figure 3 starts from 0.3 ms when the entire plasma is basically formed and ends at 1 ms, which is relatively stable at the end.
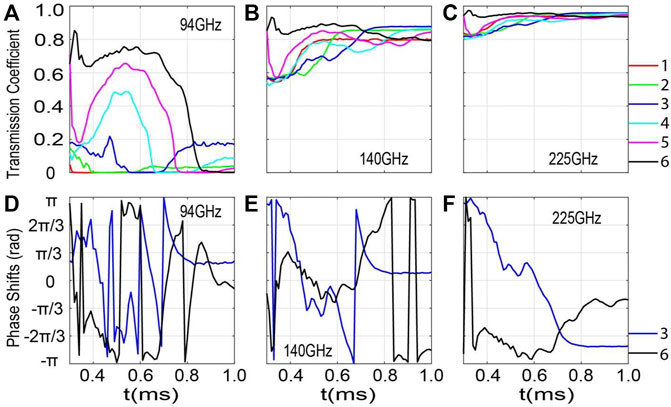
FIGURE 3. Temporal variation of transmission coefficient (A–C) and phase shift (D–F) of EHF signals in the side plasma sheath channels of a sharp-coned reentry vehicle. The corresponding carrier frequencies from left to right are 94, 140, and 225 GHz respectively. Different channels are represented by curves of different colors [as shown on the right side of (C)].
When the carrier frequency is 94 GHz, as shown in Figure 3A, the transmission coefficients of channels (1–3) near the tip are always lower than 0.2. The transmission coefficients of channels (4–6) close to the tail are significantly higher than those of channels (1–3) in the early stage of the whole evolution period, and the transmission coefficient increases as the channel move back. In the later stage of evolution, the transmission coefficients of channels 3 and 4 in the middle are higher than that of channels close to the tip and tail, and the transmission coefficient of channel 3 is the largest.
When the carrier frequency is 140 GHz, as shown in Figure 3B, the transmission coefficients of the six channels are always greater than 0.5. The transmission coefficient of channel 6 near the tail is higher than that of channels 1–5 in the early stage of the whole evolution period, and the transmission coefficient is larger as the channel closer to the tail. In the later stage of evolution, the transmission coefficient of channel 3 is the largest, but the transmission coefficients of all channels are not much different, about 0.8.
When the carrier frequency is 225 GHz, as shown in Figure 3C, the transmission coefficients of the six channels are always greater than 0.75. The transmission coefficient of channel 6 near the tail is higher than that of channels 1–5 in the early period of plasma sheath evolution. In the later stage of evolution, the transmission coefficient of channel 3 is the largest, and the transmission coefficient of all channels is about 0.95, the difference is very small.
It can be seen from Figures 3A–C that the gap of transmission coefficients of different channels decreases with time at a specific carrier frequency. In general, the transmission coefficient becomes larger in the same channel as the carrier frequency increases. The reason is that the plasma sheath is a dispersive lossy medium. For the vertical incident signal, the polarization loss is zero. The absorption caused by the collision of free electrons with neutral particles becomes the main mechanism for the energy loss of EHF signals. The total absorption rate depends on the electron density, collision frequency, and wave frequency (Chen et al., 2016). High frequencies are less absorbed than low frequencies (Ouyang et al., 2021). In addition, the transmission coefficient of channel 6 is the largest in the early stage and the transmission coefficient of channel 3 is the largest in the later stage. The following section will focus on the propagation characteristics of EHF signals in channels 3 and 6.
The temporal variation of the phase shifts of the EHF signals at the frequencies of 94, 140, and 225 GHz are shown in Figures 3D–F respectively. Since the phase shifts of each channel fluctuate significantly at a specific carrier frequency, it is messy to draw all of them. Here, only the temporal variations of phase shifts in channels 3 and 6 are shown. The vertical axis in the figure is from −π to π, which covers the entire phase cycle. It can be clearly seen from Figures 3D–F that the lower the frequency, the more drastic the phase shift changes over time. As the carrier frequency increases, the change of phase shift tends to be stable. When the carrier frequency is 225 GHz, the amplitude of phase shift change is less than one cycle during the whole evolution period. It should be noted that the phase shift is measured in the range from −π to π since it is not necessary to account for the full period ambiguity in the current modulation and demodulation problem. Once the phase changes from a value less than π to a value greater than π, it appears to drop sharply in Figures 3D–F, although it is still a continuous change. The temporal variation of the phase shift is caused by the temporal variation of the wavelength in the plasma sheath. The plasma sheath is a dispersive medium, and according to geometric optics theory, the refractive index of the plasma sheath for EHF waves depends on the electron density, electron collision frequency, and wave frequency. Therefore, the wavelength of EHF in the plasma sheath is significantly different from that in a vacuum or neutral atmosphere. In other words, the time-varying in homogeneous plasma sheath causes the wavelength of the propagating EHF signal to vary with time during propagation. Therefore, changes in wavelength cause the measured phase shift to change over time.
3 The Bit Error Rate
The BER of EHF wireless communication of sharp-coned reentry vehicles is affected by the spatiotemporal change of plasma sheath. This effect will be studied numerically in this section. According to Section 2, the transmission coefficient of channel 6 near the tail is the largest in the early stage, while that of channel 3 is the largest in the later stage. Therefore, in this section, channels 3 and 6 will be selected to study the propagation characteristics of EHF signals. Since the transmission coefficients of the six channels are relatively low in the later stage when the carrier frequency is 94 GHz, only the carrier frequencies of 140 and 225 GHz are selected in this section. In addition, the influence of antenna position and different modulation methods on BER is analyzed by Monte Carlo Simulation. The modulation modes are 2ASK, 2FSK, and 2PSK respectively.
The communication simulation system in this article includes a signal source module, modulation module, plasma sheath module, additive white Gaussian noise (AWGN) module, demodulation module, and BER statistics module. In the simulation communication system, the signal source generates random binary signals. The binary signals are modulated in the modulation module. Then, they are transferred to the plasma sheath module which causes amplitude attenuation and phase shift. After that, Gaussian white noise is added to them by the AWGN module, the noise-mixed signals are transmitted to the demodulation module, and the demodulated signals are transmitted to the BER statistics module for BER statistics. In order to compare the BER performance of various modulation methods, the AWGN module is set to the same. The power of the signal transmitter is set to 1 mW. The carrier amplitude is set to
Figure 4 shows the time-varying curves of BER of EHF signals with different modulation modes in side channels 3 and 6 of the plasma sheath of a sharp-coned reentry vehicle.
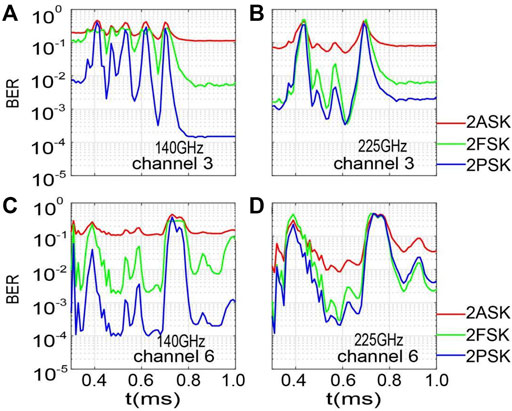
FIGURE 4. The time-varying BER of EHF signals with different modulation methods in the side channels 3 (A,B) and 6 (C,D) of the plasma sheath of a sharp-coned reentry vehicle. Left panels are results of 140 GHz, and right panels are results of 225 GHz.
As can be seen from Figure 4, if 2ASK modulation is adopted, the minimum BER magnitude of channels 3 and 6 is 10−1 when the carrier frequency is 140 GHz. However, the BER of channel 6 reaches the magnitude of 10−1 more time, so the BER performance is relatively better. When the carrier frequency is 225 GHz, the minimum BER magnitude of channel 3 is between 10−1 and 10−2, and the BER of channel 6 can reach the magnitude of 10−2, so the BER performance of channel 6 is obviously better than that of channel 3. When channel 6 and 225 GHz carrier frequencies are selected, BER performance can reach the best.
If 2FSK modulation is adopted, when the central carrier frequency is 140 GHz, the BER of channels 3 and 6 can reach the magnitude between 10−2 and 10−3, but the BER performance of channel 6 is obviously better on the whole. When the central carrier frequency is 225 GHz, the BER of channels 3 and 6 can reach the magnitude between 10−3 and 10−4, and the BER performance of channel 6 is slightly better than that of channel 3. The BER performance is better when channel 6 is adopted and with a central carrier frequency of 225 GHz.
If 2PSK modulation is used, when the carrier frequency is 140 GHz, the BER of channel 3 can reach the magnitude between 10−3 and 10−4, but the BER of channel 6 can reach the magnitude of 10−4, and the performance is relatively better. When the carrier frequency is 225 GHz, the BER of channels 3 and 6 can reach the magnitude between 10−3 and 10−4. However, the time when the BER is less than 10−3 in channel 6 is more, the BER performance is relatively better. In the same channel, the BER performance is better when the carrier frequency is 140 GHz. Better BER performance can be obtained by combining 140 GHz carrier frequency with channel 6.
In a word, 2ASK has the worst BER performance and 2PSK has the best BER performance under the specific channel and carrier frequency. Overall, 2PSK modulation combined with 140 GHz carrier frequency and channel 6 can achieve the best BER performance.
4 Discussion
According to Section 2, the transmission coefficient of EHF signals in the side channel of the plasma sheath of a sharp-coned reentry vehicle increases with carrier frequency, while the gap between different channels at specific carrier frequency decreases with time. In addition, the transmission coefficient of channel 6 near the bottom of the vehicle is the largest in the early stage, while the transmission coefficient of channel 3 in the middle of the vehicle is the largest in the later stage.
In Figure 3, the transmission coefficient at 225 GHz is obviously higher than 140 GHz, particularly for channel 3, yet the BER of channel 3 at 225 GHz in Figure 4 is not significantly higher than 140 GHz. The reason is that the BER performance depends on not only the signal strength or SNR but also the phase shift of the carrier waves. The wavelengths of 225 GHz are much shorter than that of 140 GHz. Hence the phase for the signals at 225 GHz varies more frequently during the signal transmission process than that at 140 GHz. In other words, the phase of signals at 225 GHz could be less stable than that at 140 GHz. Hence, although the signals at 225 GHz suffer less attenuation in the plasma sheath, it is not a sufficient guarantee to achieve better BER performance.
According to the numerical simulation in Section 3, the 2PSK modulation with a carrier frequency of 140 GHz and channel 6 can achieve the best BER performance. Under specific channel and carrier frequency, the BER performance of 2ASK is the worst and that of 2PSK is the best.
Figure 5 shows the BER of the EHF signals at different decision thresholds in the side channel 6 of the plasma sheath of the sharp-coned reentry vehicle at a specific time (t = 1 ms), and the curves of the maximum electron density of the side channels 1–6 of the plasma sheath of the sharp-coned reentry vehicle.
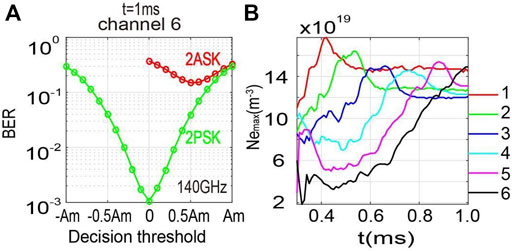
FIGURE 5. (A) The BER of the EHF signals at different decision thresholds in the side channel 6 of the plasma sheath of sharp-coned reentry vehicle at a specific time (t = 1 ms), where Am represents the amplitude of the signal; (B) The time-varying curves of the maximum electron density of the side channels (1–6).
As can be seen from Figure 5A, the optimal decision threshold of the 2PSK system is 0. The optimal threshold of 2PSK is independent of the amplitude, but the optimal threshold of 2ASK is half of the amplitude. Therefore, in the time-varying fading channel, the BER of 2ASK is relatively high, while the BER of 2PSK is relatively low.
It can be seen from Figure 5B that the maximum electron density of the side channels 1–6 of the plasma sheath of the sharp-coned reentry vehicle varies with time, so the transmission coefficient and phase shift of the EHF signals are time-varying. Furthermore, the BER is affected by the transmission coefficient and the phase shift is also time-varying.
In general, the maximum electron density of the side channel of the plasma sheath of the sharp-coned reentry vehicle becomes lower from channel 1 to channel 6. The maximum electron density of channel 1 is always in the magnitude of 1020 m−3, while the maximum electron density of channel 6 is the lowest in a long time, and the variation range is the largest, from about 2 × 1019 m−3 to 1.5 × 1020 m−3. At the end of evolution, the maximum electron density of channel 3 is the lowest. As electron density is a key parameter affecting the transmission coefficient in plasma sheath, the transmission coefficient decreases with the electron density increasing. Therefore, in the early stage, the transmission coefficient of channel 6 is the largest and the BER is relatively smaller in channel 6, and in the later stage of evolution, the transmission coefficient of channel 3 is the largest and the BER is relatively smaller in channel 3.
According to Figure 3, in channel 6, the transmission coefficient is basically greater than 0.8 when the carrier frequency is 140 GHz. Once the carrier frequency is 225 GHz, the transmission coefficient is basically above 0.9. The transmission coefficients at the two carrier frequencies have little difference in channel 6. According to Figure 4, when the 2PSK modulation is adopted, the BER of 140 GHz is smaller than that of 225 GHz. It should be concerned that signals at 225 GHz suffer greater attenuation in a neutral atmosphere than that at 140 GHz. Thus, 140 GHz is recommended to be the operating frequency for the EHF communication system serving sharp-coned reentry vehicles.
5 Conclusion
In this article, the effects of spatiotemporal heterogeneity of plasma sheath of the sharp-coned reentry vehicle on the transmission coefficient and phase shift of EHF signals are studied. It is found that the transmission coefficient of EHF signal in the plasma sheath side channels of sharp-coned reentry vehicle increases with the increase of carrier frequency, the transmission coefficient gap between different channels decreases with time at a specific carrier frequency, and the phase shift tends to be stable with the increase of carrier frequency. The BER performance of EHF signals modulated by 2ASK, 2FSK, and 2PSK in the plasma sheath of a sharp-coned reentry vehicle is numerically studied. The influence of the decision threshold on BER is discussed, and the reason for the time-varying of BER is discussed according to the time-varying curves of the maximum electron density of the six channels. The electron density distribution of different channels affects the transmission coefficient and phase shift and further affects the BER. The study found that the EHF communication system with 2PSK modulation, 140 GHz carrier, and antenna installed on the side wall and close to the bottom of the vehicle can reduce the BER for sharp-coned reentry vehicles. This study reveals the propagation characteristics and BER performance of EHF signals in the plasma sheath of a sharp-coned reentry vehicle, which can provide a valuable reference for the design of EHF communication systems for sharp-coned reentry vehicles.
Data Availability Statement
The raw data supporting the conclusion of this article will be made available by the authors, without undue reservation.
Author Contributions
All authors listed have made a substantial, direct, and intellectual contribution to the work and approved it for publication.
Conflict of Interest
The authors declare that the research was conducted in the absence of any commercial or financial relationships that could be construed as a potential conflict of interest.
Publisher’s Note
All claims expressed in this article are solely those of the authors and do not necessarily represent those of their affiliated organizations, or those of the publisher, the editors, and the reviewers. Any product that may be evaluated in this article, or claim that may be made by its manufacturer, is not guaranteed or endorsed by the publisher.
Acknowledgments
The authors acknowledge the support from the National Natural Science Foundation of China (Grant No. 61861031).
References
Chen, J., Yuan, K., Shen, L., Deng, X., Hong, L., and Yao, M. (2016). Studies of Terahertz Wave Propagation in Realistic Reentry Plasma Sheath. Pier 157, 21–29. doi:10.2528/pier16061202
Gillman, E. D., Foster, J. E., and Blankson, I. M. (2010). Review of Leading Approaches for Mitigating Hypersonic Vehicle Communications Blackout and a Method of Ceramic Particulate Injection via Cathode Spot Arcs for Blackout Mitigation. NASA/TM-2010-216220. Washington, DC: NASA.
He, G., Zhan, Y., Ge, N., Pei, Y., Wu, B., and Zhao, Y. (2014). Channel Characterization and Finite-State Markov Channel Modeling for Time-Varying Plasma Sheath Surrounding Hypersonic Vehicles. Pier 145, 299–308. doi:10.2528/pier14031104
Hu, B. J., Wei, G., and Lai, S. L. (1999). Smm Analysis of Reflection, Absorption, and Transmission from Nonuniform Magnetized Plasma Slab. IEEE Trans. Plasma Sci. 27, 1131–1136. doi:10.1109/27.782293
Liu, J.-F., Ma, H.-Y., Jiao, Z.-H., Bai, G.-H., Fang, Y., Yi, Y.-M., et al. (2020). Effects of Dynamic Plasma Sheath on Electromagnetic Wave Propagation and Bit Error Rate under External Magnetic Field. IEEE Trans. Plasma Sci. 48, 2706–2714. doi:10.1109/tps.2020.3006955
Ouyang, W., Deng, W., and Wu, Z. (2020). Impact of Half-Angles on the Transmission of Terahertz Wave in Inhomogeneous Plasma Sheath. IEEE Trans. Plasma Sci. 48, 4029–4036. doi:10.1109/tps.2020.3029837
Ouyang, W., Jin, T., Wu, Z., and Deng, W. (2021). Study of Terahertz Wave Propagation in Realistic Plasma Sheath for the Whole Reentry Process. IEEE Trans. Plasma Sci. 49, 460–465. doi:10.1109/tps.2020.3042220
Rao, Q., Xu, G., Wang, P., and Zheng, Z. (2021). Study on the Propagation Characteristics of Terahertz Waves in Dusty Plasma with a Ceramic Substrate by the Scattering Matrix Method. Sensors 21, 263. doi:10.3390/s21010263
Shi, L., Guo, B., Liu, Y., and Li, J. (2012). Characteristic of Plasma Sheath Channel and its Effect on Communication. Pier 123, 321–336. doi:10.2528/pier11110201
Starkey, R. (2003). “EM Wave/magnetoactive Plasma Sheath Interaction for Hypersonic Vehicle Telemetry Blackout Analysis,” in 34th AIAA Plasmadynamics and Lasers Conference, Orlando, United States, June 23–26, 4167.
Starkey, R. P. (2015). Hypersonic Vehicle Telemetry Blackout Analysis. J. Spacecr. Rockets 52, 426–438. doi:10.2514/1.a32051
Tang, R., Mao, M., Yuan, K., Wang, Y., and Deng, X. (2019). A Terahertz Signal Propagation Model in Hypersonic Plasma Sheath with Different Flight Speed. Phys. Plasmas 26, 043509. doi:10.1063/1.5091676
Tang, R., Xiong, Z., Yuan, K., Mao, M., Wang, Y., and Deng, X. (2021). Ehf Wave Propagation in the Plasma Sheath Enveloping Sharp-Coned Hypersonic Vehicle. Antennas Wirel. Propag. Lett. 20, 978–982. doi:10.1109/lawp.2021.3068575
Wei, H., Liu, Y., Shi, L., Yao, B., and Li, X. (2019). Bit Error Rate and Channel Capacity Performance of Telemetry Modulation Methods under Typical Reentry Plasma Sheath Channel. IEEE Trans. Plasma Sci. 47, 4950–4960. doi:10.1109/TPS.2019.2942606
Yang, X., Yuan, K., Wang, Y., Liu, Y., and Xiong, J. (2022a). Propagation Characteristics of Modulated Ehf Signal in the Wake Region of Plasma Sheath. Aerospace 9, 194. doi:10.3390/aerospace9040194
Yang, X., Yuan, K., Wang, Y., and Mao, M. (2022b). Numerical Modeling on the Bit Error Rate of Ehf Communication in Time-Varying Hypersonic Plasma Sheath. AIP Adv. 12, 045318. doi:10.1063/5.0087974
Yuan, K., Wang, Y., Shen, L., Yao, M., Deng, X., Zhou, F., et al. (2017). Sub-thz Signals’propagation Model in Hypersonic Plasma Sheath under Different Atmospheric Conditions. Sci. China Inf. Sci. 60, 1–11. doi:10.1007/s11432-017-9232-8
Yuan, K., Chen, J., Shen, L., Deng, X., Yao, M., and Hong, L. (2018a). Impact of Reentry Speed on the Transmission of Obliquely Incident Thz Waves in Realistic Plasma Sheaths. IEEE Trans. Plasma Sci. 46, 373–378. doi:10.1109/TPS.2017.2788201
Yuan, K., Shen, L., Yao, M., Deng, X., Chen, Z., and Hong, L. (2018b). Studies on the Transmission of Sub-thz Waves in Magnetized Inhomogeneous Plasma Sheath. Phys. Plasmas 25, 013302. doi:10.1063/1.5021363
Zhao, Z., Bai, B., Yuan, K., Tang, R., Xiong, J., and Wang, K. (2022a). Effect of Terahertz Antenna Radiation in Hypersonic Plasma Sheaths with Different Vehicle Shapes. Appl. Sci. 12, 1811. doi:10.3390/app12041811
Keywords: extremely high frequency (EHF), plasma sheath, sharp-coned vehicle, reentry, BER
Citation: Yang X, Yuan K, Wang Y and Liu Y (2022) Evaluation of BER for the EHF Communication System Serving Sharp-Coned Reentry Vehicles. Front. Earth Sci. 10:933083. doi: 10.3389/feart.2022.933083
Received: 30 April 2022; Accepted: 07 June 2022;
Published: 13 July 2022.
Edited by:
Chao Xiong, Wuhan University, ChinaReviewed by:
Weihua Luo, South-Central University for Nationalities, ChinaYuhua Zou, Guilin University of Electronic Technology, China
Copyright © 2022 Yang, Yuan, Wang and Liu. This is an open-access article distributed under the terms of the Creative Commons Attribution License (CC BY). The use, distribution or reproduction in other forums is permitted, provided the original author(s) and the copyright owner(s) are credited and that the original publication in this journal is cited, in accordance with accepted academic practice. No use, distribution or reproduction is permitted which does not comply with these terms.
*Correspondence: Yuhao Wang, wangyuhao@ncu.edu.cn