Exhumation history of the Hengchun Ridge and its implications for Taiwan orogenic processes
- 1Key Laboratory of Submarine Geosciences, Ministry of Natural Resources & Second Institute of Oceanography, Ministry of Natural Resources, Hangzhou, China
- 2Southern Marine Science and Engineering Guangdong Laboratory, Zhuhai, China
- 3School of Oceanography, Shanghai Jiao Tong University, Shanghai, China
The orogenic evolution of Taiwan is thought to have occurred with a steady and southward propagating trend since the Late Miocene. Recent studies suggest a two-stage collision for the Taiwan orogen and that the collision occurred simultaneously along the entirety of the island of Taiwan. To test this hypothesis, we evaluated 270 bathymetry profiles normal to the trench to constrain variations in the width, length, and crest of the Taiwan accretionary prism from the northern Central Ridge to the southern Hengchun Ridge. South of Taiwan Island, a gradual increase in the width and elevation of the accretionary prism of the Hengchun Ridge is noted. Assuming that the uplift of Hengchun Ridge is dominated by the accretion of hyper-stretched continental crust (HSCC), we estimated the uplift rate of the ridge (ca. 0.3 km/Ma) based on a linear regression between the HSCC and the observed depth of the prism crest. Using this uplift rate, we forward modeled the prism crest depth variations from 19.7°N to 23.5°N, and compared these values to observations. The model gives a good match to observations of the Hengchun Ridge, but significantly deeper depths to the north of Hengchun Peninsula. This suggests that the Taiwan orogeny had two stages: the first stage was dominated by structurally underplated HSCC, and the second is a combination of the arrival of the continental shelf and arc–continent collision. In addition to the widely accepted arc–continent collision, our study suggests that both the location and orientation of the continent–ocean boundary play important roles in orogeny.
Introduction
The Taiwan orogeny has been described as an evolving tectonic setting resulting from active and oblique collision between the north-trending Luzon Arc and the northeast-trending Eurasian continental margin (Suppe, 1984). This tectonic setting indicates that the collision started in the north and propagated southward, as suggested by many studies (Liu et al., 2001; Willett et al., 2003; Huang et al., 2006). Both Liu et al. (2001) and Willett et al. (2003) observed a southward propagation of the collision zone (starting at 5–7 Ma) using apatite and zircon fission-track ages based on the analysis of sedimentary rocks and bedrock in the Central Ridge of Taiwan. Based on regional geological settings and records, Huang et al. (2006) proposed a scenario in which continuous southward migration of the collision zone occurred since around 6.5 Ma. Recently, based on interpretations of the detrital thermochronology record of sediment samples in Taiwan, Resentini et al. (2020) observed a southward exhumation process, and Malusa and Fitzgerald (2020) identified a migration of fast erosion rates in the accretionary wedge from the northern to southern Coastal Range since the Late Miocene. Both studies support a southward propagation of the collision zone during oblique arc–continent collision. However, several other studies have challenged the idea of a southward propagation (Mesalles et al., 2014; Lee et al., 2015). According to thermochronological constraints and the timing of rapid subsidence in the eastern Taiwan foreland basin based on recently published zircon fission-track ages, both Lee et al. (2015) and Mesalles et al. (2014) observed an onset of exhumation in both the northern and southern parts of Taiwan Island at ca. 5–7 Ma, suggesting that collision may have occurred simultaneously along the entirety of the island. In addition, slow to moderate uplift of Central Ridge rocks since 6–7 Ma, followed by a rapid uplift after 2–3 Ma (Teng, 1990; Hsu et al., 2016; Lee et al., 2006) may suggest a two-stage collision for the Taiwan orogen, rather than a single southward arc–continent collision process.
Recent geophysical studies have observed that the passive margins of the northern South China Sea (SCS) are characterized by a wide area (>200 km) of extremely thinned hyper-stretched continental crust (HSCC), rather than a narrow transition zone, which is presently subducting along the Manila Trench (Lester et al., 2013; McIntosh et al., 2013; Eakin et al., 2014; Lester et al., 2014; Liu et al., 2018) (Figure 1). In addition, the results of seismic refraction profiles across the Hengchun Ridge show that the uplift of the Hengchun Ridge is primarily caused by the accretion of HSCC (McIntosh et al., 2013). HSCC underthrusting could be the dominant process in the initial stage of mountain building in Taiwan; this is supported by a comparison between crustal structure (McIntosh et al., 2013) and geothermal gradients (Mesalles et al., 2014) on and offshore Taiwan. Because the boundary between the oceanic crust, HSCC, and continental crust at the northern margin of the SCS have different trends than those of the Manlia Trench (Figure 1), the subducted plate along the Hengchun Ridge varies from oceanic crust to HSCC to continental crust moving northward. Thus, the Hengchun Ridge can be used as an analog for studying the evolution of accretionary prisms developed from oceanic crust to HSCC subduction, providing an opportunity to study the early orogenic history of Taiwan Island. Integrating these observations with onshore geology allows us to further construct the entire tectonic evolution of the Taiwan orogeny, which developed from oceanic crust subduction to arc–continental collision.
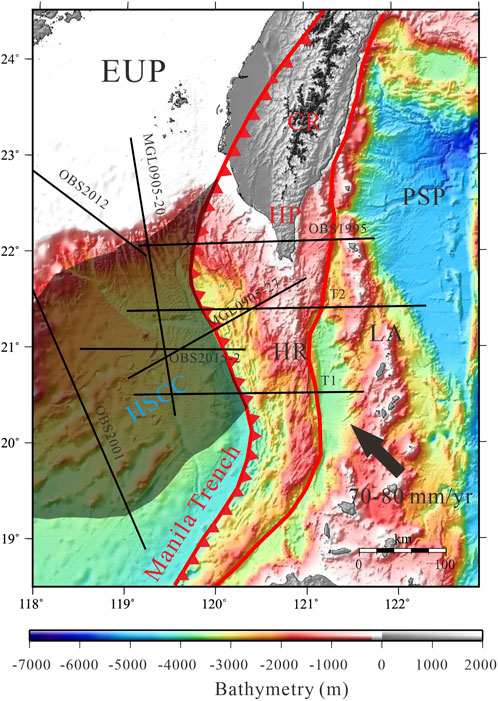
FIGURE 1. Regional features illustrated on a bathymetric map [SRTM15+; (Tozer et al., 2019)]. Solid black lines represent the locations of the regional seismic refraction profiles: OBS 2001 (Wang et al., 2006), T1 and T2 (Eakin et al., 2014), OBS 2015-2 (Liu et al., 2018), OBS 1995 (McIntosh et al., 2005), MGL0905-27 (Lester et al., 2013), MGL0905-20 (Lester et al., 2014), and OBS 2012 (Wan et al., 2017). The area of hyper-stretched continental crust (HSCC), indicated by transparent blue, is constrained by regional seismic refraction profiles and modified from Li et al. (2019). The solid red line indicates the eastern boundary of the accretionary prism. The Luzon Arc is moving northwestward towards the Eurasian continent at 70–80 mm/yr (Yu et al., 1999). CR: Central Ridge, EUP: Euroasian Plate, HR: Hengchun Ridge, HP: Hengchun Peninsula, LA: Luzon Arc, PSP: Philippine Sea Plate.
Tectonic background
Taiwan Island features an active orogenic system resulting from the collision between SE Eurasia and the Luzon Arc west of the Philippine Sea plate (Teng, 1990). It developed by at least 10 Ma during the eastward subduction of the SCS oceanic crust (Liu et al., 2019), which is characterized by a high convergent rate (8 cm/yr) (Yu et al., 1997). The initial stages of the Taiwan collision started with the arrival of Eurasian continental crust along the Manila Trench around 5–7 Ma (Willett et al., 2003; Lee et al., 2015). Following the accretion of continental crust (McIntosh et al., 2005, 2013), the prism collided with the northernmost Luzon volcanic arc of the Philippine Sea Plate, at which time more rapid uplift occurred with the arrival of thicker continental crust intersecting the prism (Mesalles et al., 2014).
Based on present-day geological observations, the tectonic setting of the region changes from north to south along the Taiwan orogen (Figure 1). In the north, the Taiwan orogen is primarily composed of three distinct geomorphic units; from west to east, these are the subducted Eurasian continental margin, the accretionary prism, and the accreted Luzon Arc (Huang et al., 2006) (Figure 1). Morphologically, the Central Ridge of Taiwan extends continuously from Hengchun Peninsula to the offshore Hengchun Ridge (Figure 1) and has a common tectonic origin (Huang et al., 2006; McIntosh et al., 2013). The Central Ridge stretches from 22°N to 25.25°N and has a length of more than 500 km. The Hengchun Peninsula, which is located at the southernmost part of Taiwan Island, is the southern extended segment of the Central Ridge, and marks the northern most part of the accretionary prism in the Manila subduction zone (Zhang et al., 2016). Based on regional tectonostratigraphic records, Huang et al. (2006) suggested that the onset of collision in the Hengchun Peninsula could have started during the early Pliocene. To the south, the submarine Hengchun Ridge stretches more than 350 km from 20.2°N to 22°N, representing a subduction wedge between the Manila Trench and the fore-arc basin of the Luzon Trough. This northward increase in width and elevation results in a significant change in morphology, likely in response to an earlier collision process (McIntosh et al., 2013). Two distinct structural domains (the upper and lower slope) have been observed in the Hengchun Ridge. The upper slope has imbricated thrusts and folds, while the lower slope is characterized by a sharp change in bathymetric relief (Lester et al., 2013; Eakin et al., 2014). The transition from lower to upper slope could be caused by out-of-sequence thrusts (Lin et al., 2008), or structurally underplated subducted crust (Lester et al., 2013). To the west, several seismic studies show that the northern SCS has a broad (>200 km) continental–ocean transition zone that comprises HSCC (stretching factor 3–5) with a thickness between 5 and 15 km, which is presently subducting at the Manila Trench (Wang et al., 2006; Lester et al., 2013; McIntosh et al., 2013; Eakin et al., 2014; Lester et al., 2014; Liu et al., 2018) (Figure 1). Furthermore, seismic studies reveal a high-velocity anomaly suggestive of a HSCC structurally underplated beneath the base of the accretionary prism (width 20 km, height 10 km) (Lester et al., 2013) (MGL0905-27 in Figure 1). This feature was also discovered beneath onshore Taiwan with a thicker (up to 30 km) and slightly wider spatial (ca. 30 km) extent (McIntosh et al., 2005, 2013) (OBS1995 in Figure 1). Based on regional seismic studies and magnetic anomalies, the subduction of oceanic crust at the Manila Trench probably occurs only to the south of 20.2°N (Eakin et al., 2014).
Materials and methods
Because the continental-ocean transition zone of the northern margin of the SCS trends differently than the Manila Trench, the subducted plate along the trench varies from oceanic to HSCC, and northward to continental crust subduction. To understand how the structure of prism growth is related to this process, we estimated changes in the morphology of the accretionary prism northward. The bathymetrical map is derived from Tozer et al. (2019) (SRTM15+) with a resolution of 15 s. For the location of the Manila Trench between 23°N and 23.5°N, we estimated the location based on a frontal thrust fault (Laonung fault) west of the Central Range. This fault was proposed following the proto-Manila trench and acted as the deformation front of the subduction wedge during the arc-continental collision (Huang et al., 1997). Between 21.5°N and 23°N, the trench represents as a deformation front on land connecting the offshore Manila Trench (e.g., Lallemand and Tsien, 1997; Liu et al., 1997; Liu et al., 2004). Here, we estimate the sea–land connection of the deformation front from Liu et al. (1997). Then, we extracted the bathymetry of the Taiwan accretionary prism normal to the trench every 2 km, representing a variation in width, slope, and crest of the accretionary prism from 19.7°N to 23.5°N. This resulted in the obtainment of 270 different profiles normal to the trench. Along the profile, the spacing is 1 km; this retains the main structural characteristics of the prism. The crest of the prism is calculated based on the average depth of the ten shallowest points of the prism. The width of the prism is estimated from the trench point to the western boundary of the prism (solid red line in Figure 1). The slope is estimated based on a standard least-squares linear regression model (y = a + b·x, where the b is the slope) for each accretionary prism using the GMT program “gmtregress” (Wessel and Smith, 1991). Although the slope could have been affected by regional processes (slumps, erosion, and mud volcanoes), a gradual process of slope change should provide a representative mechanism for the growth of the accretionary prism, where greater and lesser slopes indicate that the growth of the prism is dominated by vertical and horizontal accretion, respectively.
To analyze the exhumation history of Hengchun Ridge, it is also necessary to know its uplift rate. We first estimated the amount of subducted HSCC based on a simple geometric relationship between the Manila Trench and HSCC, where the HSCC east of the Manila Trench was simply extended following the trend of the HSCC to the west of the trench (Figure 2). Some uncertainties related to the change of geometry of the Manila Trench were noted because the trench migrated westward during subduction; however, based on reconstructions by Seton et al. (2012), the overall configuration of the northern Manila Trench has experiences little change since the Late Miocene. Moreover, to the east of the trench, the geometry of the HSCC is generally consistent with a map of a subducted thinned continental crust slab, which is unfolded and restored to the Earth surface based on the regional tomography data provided by Wu et al. (2016) and Liu et al. (2018). Using an E–W effective convergence rate of 50 mm/yr for the northern Manila Trench (Tan, 2020), we split the E–W convergence rate normal to the trench and calculated a trench-normal subduction plate velocity along the trench. Then, the duration of the subduction of HSCC could be estimated. If the growth of the Hengchun Ridge was dominated by underplating of HSCC to the accretionary prism, a positive correlation between the duration of the subducted HSCC and the depth of the prism crest should be observed. Subsequently, the uplift rate of the Hengchun Ridge can be estimated by using linear regression to fit the data.
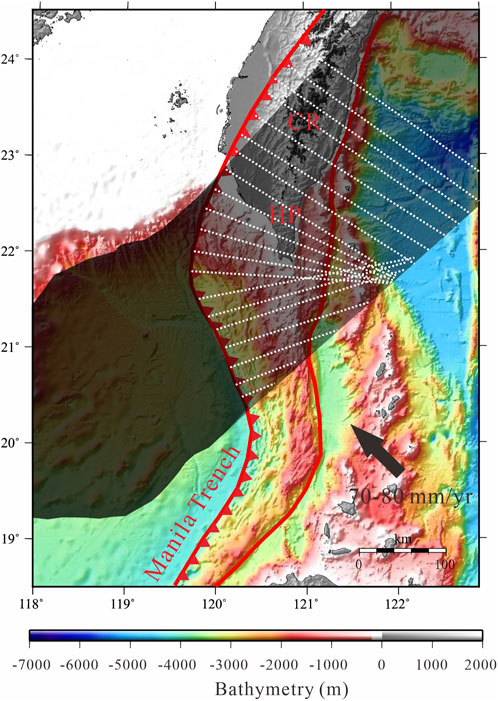
FIGURE 2. Area of the hyper-stretched continental crust (HSCC) in the study region. The distribution of HSCC to the east of the Manila Trench was simply extended following the trend of HSCC west of the trench. HSCC subduction normal to the trench is indicated by white dashed lines. Owing to different trends between the trench and the HSCC, an increasing amount of HSCC subduction occurs from Hengchun Ridge to Hengchun Peninsula. CR: Central Ridge, HR: Hengchun Ridge, HP: Hengchun Peninsula, LA: Luzon Arc.
Results
Hyper-stretched continental crust underplating
The amount and duration of HSCC subduction between 19.7°N and 23.5°N is shown in Figure 3A. The area between 19.7°N and 21.5°N includes the Hengchun Ridge (offshore), while the Hengchun Peninsula covers an area between 21.5°N and 22.5°N. The area north of 22.5°N represents the Central Ridge. The initial stage of HSCC subduction is at 20.2°N. A gradually increasing amount of HSCC subduction is noted between 20.2°N and 21.5°N (0–230 km); this corresponds to the duration of HSCC subduction from 0 to 5 Ma. Between 21.5°N to 23.5°N, the duration and amount of subducted continental crust remain almost unchanged at 5 Ma and 240 km, respectively. The relationship between the duration of HSCC subduction and the observed depth of the prism crest over Hengchun Ridge is shown in Figure 3B. In general, a strong positive correlation is observed between the two, with a correlation coefficient (R) of 0.93. The observed prism crest increases from −2000 to 0 m and is associated with an increase in duration from 0 to 5 Ma. Linear regression determined that the uplift rate of the Hengchun Ridge is 0.296 ±0.011 km/Ma (red circle and solid line in Figure 3B). There are some uncertainties in the uplift rate due to the uncertainties of the convergence rate. Previous studies have shown that the Philippine Sea Plate is moving toward the Eurasian continent at the rate of 60–80 mm/yr in 300°–310°azimuth (Yu et al., 1997; Yu et al., 1999). This results in E–W effective convergence rate at the trench from 45 to 70 mm/yr. Here, we tested the sensitivity of the convergence rate from 45 mm/yr to 70 mm/yr to see how it changes from the uplift rate. The result shows that the changing of the convergence rate still results in a positive correlation between the duration of HSCC subduction and the prism crest, and the uplift rate changes to 0.266 km/Ma and 0.355 km/Ma at a convergence rate of 45 and 70 mm/yr, respectively, (green and blue circles and dashed lines in Figure 3B).
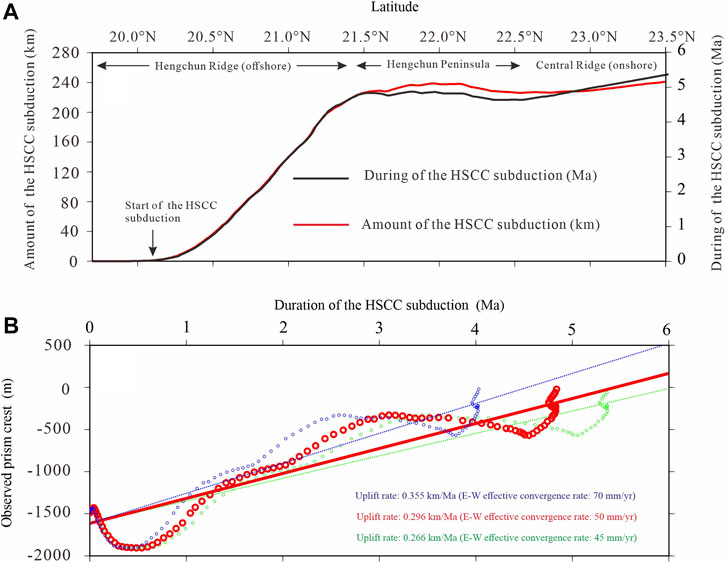
FIGURE 3. (A) Amount and duration of hyper-stretched continental crust (HSCC) subduction. The start of HSCC subduction occurs at 20.2°N. For Hengchun Ridge, the amount and duration of HSCC subduction increases from 0 to 220 km and 0 to 5 Ma, respectively. For the onshore Hengchun Peninsula and Central Ridge, the amount and duration remain constant at 240 km and 5 Ma, respectively. (B) Correlation between the duration of the HSCC subduction and observed crest of the prism over Hengchun Ridge. The red circle shows the correlation based on the E-W effective convergence rate of 50 mm/yr, and a red solid line indicates a best-fit line. The green and blue circles and dashed lines represent the correlation based on the E-W convergence rate of 45 and 70 mm/yr, respectively. The positive correlation suggests that the uplift of Hengchun Ridge is dominated by the accretion of HSCC underthrusting.
Morphology of the taiwan accretionary prism
The morphology of the Taiwan accretionary prism is shown in Figure 4. To the south of 20.2°N, oceanic crust subduction exhibits less variation in width (70–80 km), slope (0.04–0.05), and crest of the prism (−1,500 m to −1,200 m). Between 20.2°N and 21.3°N (the Hengchun Ridge), the crest of the prism gradually increases (−1,500 m to −500 m); this is associated with an increase in width from 70 to 110 km. The northward increase in the size of the prism probably results from the accretion of terrigenous sediments in the SCS continental margin and Taiwan orogen to the north. The prism slope is almost the same (0.04), suggesting that the growth of the accretionary prism can be attributed to both vertical and horizontal accreted. From 21.6°N to 22.2°N, the width increases from 130 to 150 km; this is associated with a minor change in crest depth (from −500 to 0 m). A decreasing slope in this area (from 0.03 to 0.02) suggests that the growth of the accretionary prism is marked by horizontal advection. North of 22.5°N represents the Hengchun Peninsula and Central Ridge. Here the crest of the accretionary prism exhibits significant uplift from 0 to 3,500 m. The width of the accretionary prism is almost constant at 140 km, while the slope dramatically increases from 0.01 to 0.04, reflecting the rapid vertical growth of the accretionary prism. The length and slope of the accretionary prism could be affected by the uncertainties of the location of the Manila Trench just SW of Taiwan Island, as the Manila Trench gradually loses its bathymetric identity north of 21.5°N. There are several possible ways of the connection between the deformation front on land and the Manila Trench offshore in SW Taiwan Island (e.g., Huang et al., 2004; Yu, 2004; Han et al., 2017). Here, we test the sea–land connection proposed by Han et al. (2017), Lacombe et al. (2001)), and Lin et al. (2008) (Figure 4A) to see how it changes the estimated length and slope of the accretionary prism. The test shows that the length of the accretionary prism changes of up to 40 km, while the slope to be changed by generally less than 0.01. In addition, the large amounts of mud diapirs and mud volcanos are active offshore and onshore SW Taiwan Island (e.g., Chen et al., 2014; Doo et al., 2015) could potentially change the height, accordingly the slope of the accretionary prim. Regional seismic reflection profiles show that the height of the mud volcano is generally less than 400 m (Chen et al., 2014), which results in slope changes of up to 0.003.
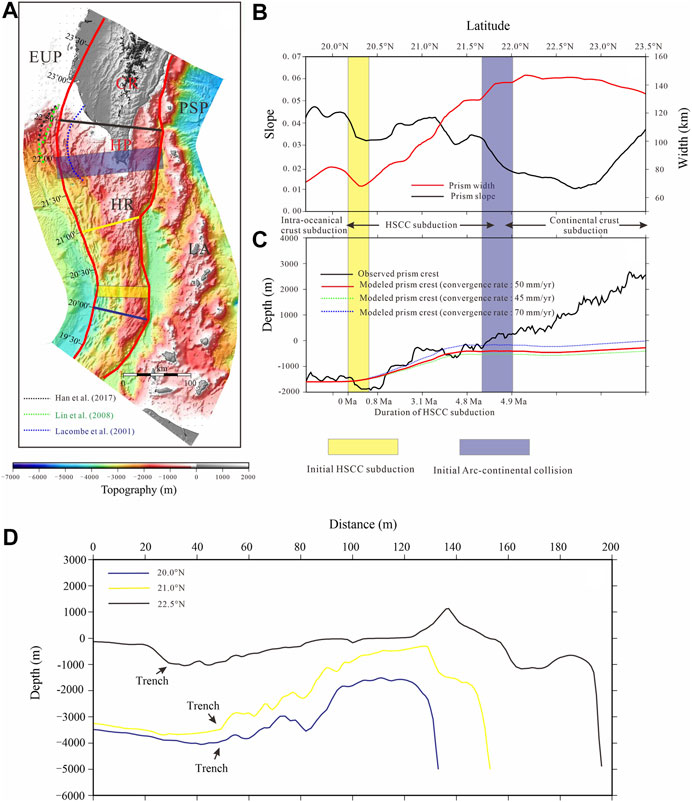
FIGURE 4. (A) Bathymetry of the Taiwan accretionary prism from 19.5°N to 24°N. Solid red lines indicate the western and eastern boundaries of the accretionary prism. Black, green, and blue dotted lines indicate possible trench locations proposed by Han et al. (2017), Lin et al. (2008), and Lacombe et al. (2001) SW of Taiwan Island. (B) Variations in prism width and slope from 19.7°N to 23.5°N. (C) Comparison between the modeled (red solid line) and observed prism crest (black solid line) from 19.7°N to 23.5°N. The modeled duration of the subducted hyperextended continental crust is shown below. The green and blue dash lines show the modeled prism crest using E-W effective convergence rate at 45 and 70 mm/yr, respectively. (D) Profiles across the accretionary prism at 20.0°N, 21.0°N, and 21.5°N, representing a typical cross section of oceanic subduction, hyper-stretched continental crust subduction, and initial arc–continental collision, respectively. CR: Central Ridge, EUP: Eurasian Plate, HR: Hengchun Ridge, HP: Hengchun Peninsula, LA: Luzon Arc, PSP: Philippine Sea Plate.
Discussion
A range of interpretations have been proposed for the evolution of the Taiwan orogen. Based on regional tectonic settings, subduction of SCS crust results in an oblique arc–continent collision starting at approximately 6.5 Ma in northern Taiwan and propagating southward (Huang et al., 2006). Using stratigraphic evidence, low-temperature thermochronological analysis, and regional seismic data, several studies have proposed a two-stage collision for the Taiwan orogen (Lee et al., 2006; Mesalles et al., 2014; Hsu et al., 2016). The first stage started around 6–8 Ma (Teng, 1990; Liu et al., 2001; Lee et al., 2006) with a southward propagation of arc–continent collision (Liu et al., 2001; Willett et al., 2003; Malusa and Fitzgerald, 2020; Resentini et al., 2020) or a simultaneous mountain building along the Taiwan orogenic belt (Lee et al., 2006; Mesalles et al., 2014; Lee et al., 2015; Hsu et al., 2016). The simultaneous hypothesis is supported by the fact that both the Luzon Arc and continental margin exhibit subparallel northward trends during the Late Miocene (Lee et al., 2015). The uplift of the accretionary wedge can be caused by arc–continent collision (Lee et al., 2015; Resentini et al., 2020), subduction of extended continental crust (Lee et al., 2006; Mesalles et al., 2014), HSCC structural underplating at the base of the accretionary prism (Lester et al., 2013; McIntosh et al., 2013), and a combination of crustal accretion, subducting continental crust, and southward propagation of the arc collision (Willett et al., 2003). During this stage, the exhumation rate was slow to moderate (<0.5 km/Ma) (Lee et al., 2006; Hsu et al., 2016). The second stage recorded an additional period of shortening and exhumation. The rate of exhumation significantly accelerated to 2–10 km/Ma at ca. 1–3 Ma (Teng, 1990; Willett et al., 2003; Lee et al., 2006; Mesalles et al., 2014; Hsu et al., 2016). A consistent acceleration in exhumation rate is observed along the entirety of the Taiwan orogen, but the exhumation rate at south is lower than that to the north, suggesting a longer collision history in the north (Lee et al., 2006; Hsu et al., 2016). The acceleration of the exhumation rate could be caused by progressive underthrusting of thicker crust (Lee et al., 2015; Hsu et al., 2016) and shortening of the prism associated with Luzon Arc collision (Lee et al., 2006; Mesalles et al., 2014).
Our data show that the width and crest of the Hengchun accretionary prism gradually increase northward (Figure 4). Assuming prism growth from only the subducted HSCC, we used the duration of the HSCC and the uplift rate of the Hengchun Ridge (0.296 km/Ma) to forward model how much of the accretionary prism has been uplifted north of 19.7°N (Figure 4C). The result shows that our modeled prism crest (red solid line in Figure 4C) fits reasonably well with observations south of 22°N. Considering the uncertainties of the convergence rate, changing the E-W convergence rate to 45 mm/yr and 70 mm/yr (green and blue dashed line in Figure 4C) still result in a reasonable fit between the observed and modeled prism crest. The estimated uplift rate of the Hengchun Ridge (0.266–0.355 km/Ma) is in agreement with the exhumation rate (<0.5 km/Ma) characterizing the early stages of mountain formation in Taiwan (Lee et al., 2006; Hsu et al., 2016). In addition, our model shows that the initial age of HSCC subduction at Hengchun Peninsula (22°N) is 4–5 Ma (Figure 4C). This is comparable to the regional sedimentary record, which indicates that the onset of the submarine collision in the Hengchun Peninsula could have started during the early Pliocene (Huang et al., 2006). The slope of the Hengchun accretionary prism (21°N to 22°N) gradually decreases from 0.04 to 0.02. Considering an uncertainty of the slope by up to 0.01, it still shows a decreasing trend, suggesting that the growth of the Hengchun Ridge is dominated by horizontal advection. This is comparable to the first stages of Taiwan orogenic formation, where the horizontal transport of materials from the prism was more important than its vertical uplift (Willett et al., 2003). In conclusion, the similarity of the exhumation rate, duration, and mechanism of submarine collision both onshore and offshore Taiwan suggest a common tectonic origin between the two. It is likely that the first stage of the Taiwan orogen was dominated by HSCC subduction. The subparallel trend between the trench and the continental margin supports simultaneous mountain building along the Taiwan orogenic belt during the first stage of collision (Figure 5).
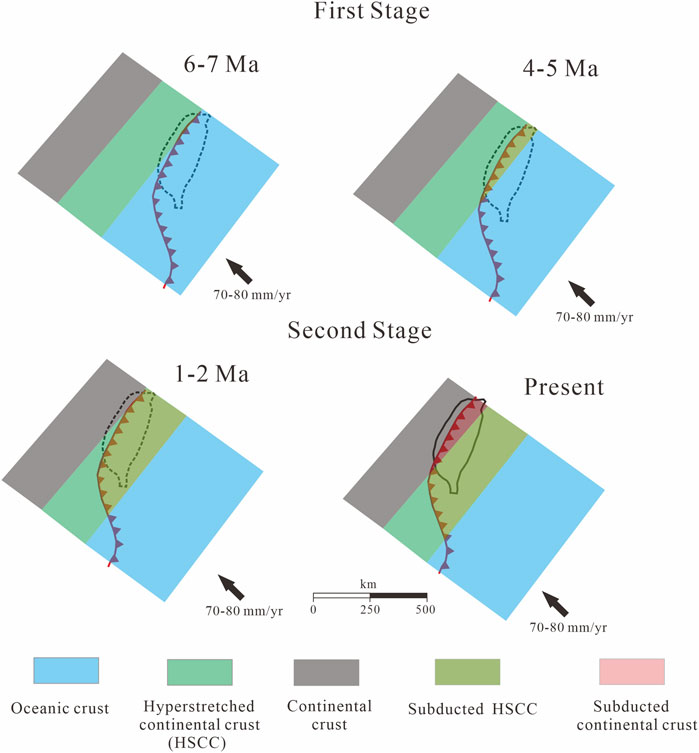
FIGURE 5. Evolution of the Taiwan accretionary prim since the Late Miocene. The location of the trench is derived from Deng et al. (2020) and the area of the HSCC is modified from Li et al. (2019). Owing to differences in orientation between the Manila Trench and the hyper-stretched continental crust (HSCC) of the northern South China Sea, the amount of HSCC subduction changes from south to north and has varied through time. The subparallel trend between the trench and the continental margin supports simultaneous mountain building along the Taiwan orogenic belt during the first stage of the Taiwan orogeny.
North of 22°N, the observed prism crest experienced a dramatic uplift. This region also features a significantly higher (2,000–3,000 m) prism crest than modeled values, indicating that the growth of the prism is not dominated by HSCC subduction, evidencing another stage of collision evolution. During this stage, a significant increase in prism slope (from 0.01 to 0.04) indicates the dominance of vertical uplifting over horizontal advection. This is in agreement with more rapid uplift; indeed, the exhumation rate increases to 2–10 km/Ma during the second stage of the Taiwan orogen (Lee et al., 2006; Hsu et al., 2016). In addition, considering the onset of the first stage Taiwan collision at 6–7 Ma (Lee et al., 2006) and the duration of HSCC subduction (5 Ma; Figure 4), the estimated duration of the second collision stage is 1–2 Ma. This is in agreement with several onshore studies suggesting that the second stage of the Taiwan orogen began at around 1–3 Ma (Teng, 1990; Willett et al., 2003; Lee et al., 2006; Mesalles et al., 2014). Our model shows that to the north of the Hengchun Peninsula, thick continental crust subduction caused significant uplift the accretionary prim (Figure 5). In addition, a gradual increase in both prism crest and slope northward suggests that the north experienced rapid uplift (Figure 4C). This is consistent with lower exhumation rates recorded in the Hengchun Peninsula than the Central Ridge (Lee et al., 2006). This exhumation rate could be related to the combination of the oblique collision between the continental crust and north-trending Luzon Arc result in shortening of continental margin section, and the southward-increasing erosion rate at Taiwan Island (Malusa and Fitzgerald, 2020).
Conclusion
Based on a combination of the exhumation history of the Hengchun accretionary prism and the onshore geology of Taiwan Island, our study supports a two-stage Taiwan orogen. Owing to the difference in orientation between the Manila Trench and the HSCC of the northern SCS, the amount of HSCC subduction over the Hengchun Ridge has changed from south to north (Figure 5). We use this as an analog to analyze the first stage of the Taiwan orogeny. During this stage, the uplift rate of Hengchun Ridge is estimated based on correlations between the duration of HSCC subduction and the observed depth of the prism crest, and uses linear regression to perform the fit. The initial HSCC subduction is coeval with the start of the Taiwan orogen at 6–7 Ma. In addition, the uplift of Hengchun Ridge is dominated by an accretion of structurally underplated HSCC, which is dominated by horizontal advection rather than vertical uplift. The exhumation rate is moderate to low (ca. 0.3 km/Ma) and is comparable to the exhumation rate (<0.5 km/Ma) of the first stage of Taiwan mountain formation. The subparallel trend between the trench and continental margin supports simultaneous mountain accretion along the entire Taiwan orogen. After subduction of the entire HSCC, the second stage of the Taiwan orogeny occurred. During this stage, the exhumation rate of the prism accelerated significantly. This higher rate was most likely caused by the arrival of a thick continental shelf intersecting the prism, together with continent–arc collision. Compared to the widely accepted arc–continent collision model, our study suggests that both the location and orientation of the continental–ocean boundary can also play important roles in orogeny.
Data availability statement
The datasets presented in this study can be found in online repositories. The names of the repository/repositories and accession number(s) can be found below: https://figshare.com/s/dc28a50234beeb5d128d.
Author contributions
PT and WD designed the study. PT wrote the manuscript. WD and JL helped to improve the manuscript with some suggestions. All authors contributed to the article and approved the submitted version.
Funding
This study is supported by the Natural Science Foundation of China (41890811, 42006072), Scientific Research Fund of the Second Institute of Oceanography, MNR, grant no HYGG 2001, Qianjiang talent QJD2002033, and the Innovation Group Project of Southern Marine Science and Engineering Guangdong Laboratory (Zhuhai) (No.311020018).
Acknowledgments
We are grateful to Chuanyang Wang for his detail suggestions to improve the quality of the manuscript.
Conflict of interest
The authors declare that the research was conducted in the absence of any commercial or financial relationships that could be construed as a potential conflict of interest.
Publisher’s note
All claims expressed in this article are solely those of the authors and do not necessarily represent those of their affiliated organizations, or those of the publisher, the editors and the reviewers. Any product that may be evaluated in this article, or claim that may be made by its manufacturer, is not guaranteed or endorsed by the publisher.
References
Chen, S.-C., Hsu, S.-K., Wang, Y., Chung, S.-H., Chen, P.-C., Tsai, C.-H., et al. (2014). Distribution and characters of the mud diapirs and mud volcanoes off southwest Taiwan. J. Asian Earth Sci. 92, 201–214. doi:10.1016/j.jseaes.2013.10.009
Deng, H., Ren, J., Pang, X., Rey, P. F., McClay, K. R., Watkinson, I. M., et al. (2020). South China Sea documents the transition from wide continental rift to continental break up. Nat. Commun. 11 (1), 4583. doi:10.1038/s41467-020-18448-y
Doo, W.-B., Hsu, S.-K., Lo, C.-L., Chen, S.-C., Tsai, C.-H., Lin, J.-Y., et al. (2015). Gravity anomalies of the active mud diapirs off southwest Taiwan. Geophys. J. Int. 203 (3), 2089–2098. doi:10.1093/gji/ggv430
Eakin, D. H., McIntosh, K. D., Van Avendonk, H. J. A., Lavier, L., Lester, R., Liu, C., et al. (2014). Crustal-scale seismic profiles across the Manila subduction zone: The transition from intraoceanic subduction to incipient collision. J. Geophys. Res. Solid Earth 119 (1), 1–17. doi:10.1002/2013jb010395
Han, W.-C., Liu, C.-S., Chi, W.-C., Chen, L., Lin, C.-C., Chen, S.-C., et al. (2017). Westward advance of the deformation front and evolution of submarine canyons offshore of southwestern Taiwan. J. Asian Earth Sci. 149, 6–19. doi:10.1016/j.jseaes.2017.07.001
Hsu, W., Byrne, T. B., Ouimet, W. B., Lee, Y., Chen, Y., Soest, M. V., et al. (2016). Pleistocene onset of rapid, punctuated exhumation in the eastern Central Range of the Taiwan orogenic belt. Geology 44 (9), 719–722. doi:10.1130/g37914.1
Huang, C, -Y., Wu, W, -Y., Change, C, -P., Tsao, S., Yuan, P. B., Lin, C, -W., et al. (1997). Tectonic evolution of accretionary prism in the arc-continent collision terrane of Taiwan. Tectonophysics 281, 31–51. doi:10.1016/s0040-1951(97)00157-1
Huang, C. -Y., Yuan, P. B., and Tsao, S. -J. (2006). Temporal and spatial records of active arc-continent collision in taiwan: A synthesis. Geol. Soc. Am. Bull. 118 (3-4), 274–288. doi:10.1130/b25527.1
Huang, S. T., Yang, K. M., Hung, J. H., Wu, J. C., Ting, H. H., Mei, W. W., et al. (2004). Deformation front development at the northeast margin of the tainan basin, tainan–kaohsiung area, taiwan. Mar. Geophys. Res. 25 (1), 139–156. doi:10.1007/s11001-005-0739-z
Lacombe, O., Mouthereau, F., Angelier, J., and Deffontaines, B. (2001). Structural, geodetic and seismological evidence for tectonic escape in SW Taiwan. Tectonophysics 333, 323–345. doi:10.1016/s0040-1951(00)00281-x
Lallemand, S., and Tsien, H.-H. (1997). An introduction to active collision in Taiwan. Tectonophysics 274 (1), 1–4. doi:10.1016/s0040-1951(96)00294-6
Lee, Y., Byrne, T., Wang, W., Lo, W., Rau, R., Lu, H., et al. (2015). Simultaneous mountain building in the Taiwan orogenic belt. Geology 43 (5), 451–454. doi:10.1130/g36373.1
Lee, Y., Chen, C., Liu, T., Ho, H., Lu, H., Lo, W., et al. (2006). Mountain building mechanisms in the southern central range of the taiwan orogenic belt—from accretionary wedge deformation to arc–continental collision. Earth Planet. Sci. Lett. 252 (3-4), 413–422. doi:10.1016/j.epsl.2006.09.047
Lester, R., McIntosh, K. D., Van Avendonk, H. J. A., Lavier, L. L., Liu, C. -S., Wang, T. K., et al. (2013). Crustal accretion in the Manila Trench accretionary wedge at the transition from subduction to mountain-building in Taiwan. Earth Planet. Sci. Lett. 375, 430–440. doi:10.1016/j.epsl.2013.06.007
Lester, R., Van Avendonk, H. J. A., McIntosh, K., Lavier, L., Liu, C. -S., Wang, T. K., et al. (2014). Rifting and magmatism in the northeastern South China Sea from wide-angle tomography and seismic reflection imaging. J. Geophys. Res. Solid Earth 119 (3), 2305–2323. doi:10.1002/2013jb010639
Li, F., Sun, Z., Pang, X., Liao, J., Yang, H., Xie, H., et al. (2019). Low-viscosity crustal layer controls the crustal architecture and thermal distribution at hyperextended margins: Modeling insight and application to the northern South China sea margin. Geochem. Geophys. Geosyst. 20 (7), 3248–3267. doi:10.1029/2019gc008200
Lin, A. T., Liu, C. S., Lin, C. C., Schnurle, P., Chen, G. Y., Liao, W. Z., et al. (2008). Tectonic features associated with the overriding of an accretionary wedge on top of a rifted continental margin: An example from taiwan. Mar. Geol. 255 (4), 186–203. doi:10.1016/j.margeo.2008.10.002
Liu, C.-S., Deffontaines, B., Lu, C.-Y., and Lallemand, S. (2004). Deformation patterns of an accretionary wedge in the transition zone from subduction to collision offshore southwestern Taiwan. Mar. Geophys. Res. 25 (1-2), 123–137. doi:10.1007/s11001-005-0738-0
Liu, C.-S., Huang, I. L., and Teng, L. S. (1997). Structural features off southwestern Taiwan. Mar. Geol. 137 (3), 305–319. doi:10.1016/s0025-3227(96)00093-x
Liu, H., Yumul, P., Dimalanta, C. B., Queano, K., Xia, X. P., Peng, T. P., et al. (2019). Western northern Luzon isotopic evidence of transition from proto-south China sea to south China sea fossil ridge subduction. Tectonics 39, 1–21. doi:10.1029/2019tc005639
Liu, S., Zhao, M., Sibuet, J. C., Qiu, X., Wu, J., Zhang, J., et al. (2018). Geophysical constraints on the lithospheric structure in the northeastern South China Sea and its implications for the South China Sea geodynamics. Tectonophysics 742-743, 101–119. doi:10.1016/j.tecto.2018.06.002
Liu, T., Hsieh, S., Chen, Y., and Chen, W. (2001). Thermo-kinematic evolution of the Taiwan oblique-collision mountain belt as revealed by zircon fission track dating. Earth Planet. Sci. Lett. 186 (1), 45–56. doi:10.1016/s0012-821x(01)00232-1
Malusa, M. G., and Fitzgerald, P. G. (2020). The geologic interpretation of the detrital thermochronology record within a stratigraphic framework, with examples from the European Alps, Taiwan and the Himalayas. Earth. Sci. Rev. 201 (103), 103074. doi:10.1016/j.earscirev.2019.103074
McIntosh, K. D., Nakamura, Y., Wang, T. -K., Shih, R. -C., Chen, A., Liu, C. -S., et al. (2005). Crustal-scale seismic profiles across taiwan and the Western Philippine Sea. Tectonophysics 401 (1-2), 23–54. doi:10.1016/j.tecto.2005.02.015
McIntosh, K. D., Van Avendonk, H., Lavier, L. L., Lester, W. R., Eakin, D. H., Wu, F. T., et al. (2013). Inversion of a hyper-extended rifted margin in the southern Central Range of Taiwan. Geology 41 (8), 871–874. doi:10.1130/g34402.1
Mesalles, L., Mouthereau, F., Bernet, M., Chang, C., Tien-Shun Lin, A., Fillon, C., et al. (2014). From submarine continental accretion to arc-continent orogenic evolution: The thermal record in southern taiwan. Geology 42 (10), 907–910. doi:10.1130/g35854.1
Resentini, A., Malusa, M. G., and Garzanti, E. (2020). Ongoing exhumation of the Taiwan orogenic wedge revealed by detrital apatite thermochronology: The impact of effective mineral fertility and zero-track grains. Earth Planet. Sci. Lett. 544, 116374. doi:10.1016/j.epsl.2020.116374
Seton, M., Muller, R. D., Zahirovic, S., Gaina, C., Torsvik, T., Shephard, G., et al. (2012). Global continental and ocean basin reconstructions since 200 Ma. Earth. Sci. Rev. 113 (3-4), 212–270. doi:10.1016/j.earscirev.2012.03.002
Suppe, J. (1984). Kinematics of arc-continent collision, flipping of subudction, and backarc spreading near Taiwan. Mem. Geol. Soc. China. 6, 23–56.
Tan, E. (2020). Subduction of transitional crust at the Manila Trench and its geophysical implications. J. Asian Earth Sci. 187 (104), 104100. doi:10.1016/j.jseaes.2019.104100
Teng, L. S. (1990). Geotectonic evolution of late cenozoic arc-continent collision in taiwan. Tectonophysics 183 (1-4), 57–76. doi:10.1016/0040-1951(90)90188-e
Tozer, B., Sandwell, D. T., Smith, W. H. F., Olson, C., Beale, J. R., Wessel, P., et al. (2019). Global bathymetry and topography at 15 arc sec: SRTM15+. Earth Space Sci. 6 (10), 1847–1864. doi:10.1029/2019ea000658
Wan, K., Xia, S., Cao, J., Sun, J., and Xu, H. (2017). Deep seismic structure of the northeastern south China sea: Origin of a high-velocity layer in the lower crust. J. Geophys. Res. Solid Earth 122 (4), 2831–2858. doi:10.1002/2016jb013481
Wang, T. K., Chen, M., Lee, C., and Xia, K. (2006). Seismic imaging of the transitional crust across the northeastern margin of the South China Sea. Tectonophysics 412 (3-4), 237–254. doi:10.1016/j.tecto.2005.10.039
Wessel, P., and Smith, W. H. F. (1991). Free software helps map and display data. Eos Trans. AGU. 72 (441), 441. doi:10.1029/90eo00319
Willett, S. D., Fisher, D., Fuller, C., En-Chao, Y., and Chia-Yu, L. (2003). Erosion rates and orogenic-wedge kinematics in Taiwan inferred from fission-track thermochronometry. Geol. 31 (11), 945. doi:10.1130/g19702.1
Wu, J., Suppe, J., Lu, R., and Kanda, R. (2016). Philippine Sea and East Asian plate tectonics since 52 Ma constrained by new subducted slab reconstruction methods. J. Geophys. Res. Solid Earth 121, 4670–4741. doi:10.1002/2016jb012923
Yu, H.-S. (2004). Nature and distribution of the deformation front in the Luzon Arc-Chinese continental margin collision zone at Taiwan. Mar. Geophys. Res. 25 (1), 109–122. doi:10.1007/s11001-005-0737-1
Yu, S.-B., Kuo, L.-C., Punongbayan, R. S., and Ramos, E. G. (1999). GPS observation of crustal deformation in the Taiwan-Luzon Region. Geophys. Res. Lett. 26 (7), 923–926. doi:10.1029/1999gl900148
Yu, S. B., Chen, H. Y., and Kuo, L. C. (1997). Velocity field of GPS stations in the Taiwan area. Tectonophysics 274 (1-3), 41–59. doi:10.1016/s0040-1951(96)00297-1
Keywords: hengchun ridge, taiwan orogenic, manila subduction, hyper-stretched continental crust, south China sea
Citation: Tan P, Ding W and Li J (2022) Exhumation history of the Hengchun Ridge and its implications for Taiwan orogenic processes. Front. Earth Sci. 10:941040. doi: 10.3389/feart.2022.941040
Received: 11 May 2022; Accepted: 12 July 2022;
Published: 24 August 2022.
Edited by:
Gang Rao, Southwest Petroleum University, ChinaReviewed by:
Huiping Zhang, China Earthquake Administration, ChinaWen-Nan Wu, National Central University, Taiwan
Copyright © 2022 Tan, Ding and Li. This is an open-access article distributed under the terms of the Creative Commons Attribution License (CC BY). The use, distribution or reproduction in other forums is permitted, provided the original author(s) and the copyright owner(s) are credited and that the original publication in this journal is cited, in accordance with accepted academic practice. No use, distribution or reproduction is permitted which does not comply with these terms.
*Correspondence: Pingchuan Tan, tanpc@sio.org.cn