- 1Departamento de Ecología y Territorio, Facultad de Estudios Ambientales, Pontificia Universidad Javeriana, Bogotá, Colombia
- 2Instituto de Hidrología, Meteorología y Estudios Ambientales, IDEAM, Bogotá, Colombia
Methane emitted by wetlands accounts for nearly 30% of the global methane emissions and is expected to double by 2,100. Methane fluxes from peatlands are related to m soil temperature, water table, and vegetation. Disturbances, mostly drainage, are considered to have a more limited impact on CH4 fluxes from peatlands. Here, we studied the temporal and spatial patterns of methane emissions from four peatlands across and elevation and disturbance gradient in the northern Andes. Our research seeks to understand the effects of disturbance legacies and soil temperature, flooding patterns, and vegetation on methane emissions in Andean peatlands. Our results indicated a strong control of CH4 emissions by soil temperature with a stronger effect at intermediate elevations. The removal of peat forming vegetation from the surface had the higher effect on CH4 emissions, increasing methane fluxes by an order of magnitude. Methane fluxes from conserved cushion vascular plants were low, but nearby hollows had relatively high emissions. Our research provides evidence of high methane emissions from degraded peatlands in the tropical Andes. However, the role of climate change and disturbance legacies on methane emissions from tropical Andean peatlands remains unknown affecting our ability to predict future emission patterns and our ability to incorporate climate mitigation strategies.
1 Introduction
Peatlands are a major source of atmospheric CH4 emissions accounting for nearly 30% of the global overall inputs (Saunois et al., 2020; Canadell et al., 2021). CH4 concentration in the atmosphere has been increasing since 1980, albeit with a lower rate of increase between 2000 and 2010 (Canadell et al., 2021). CH4 emissions from peatlands are highly dependent on soil temperature (Koffi et al., 2020), with sites at mid and high elevations showing a higher sensitivity (Zhu et al., 2021). Thus, a higher rate of CH4 emissions from peatlands is predicted under expected higher air temperatures, with increases ranging from 50% to 80% the current emission levels by the year 2,100 (Koffi et al., 2020). However, human driven disturbances on peatland ecosystems are far from homogenous and go beyond simple drainage. Changes in land cover including the replacement of peat forming vegetation and the planting of grasses for cattle fed can have large impacts on the decomposition pathways of the organic matter within the peat (Planas-Clarke et al., 2020; Wang et al., 2021).
The northern tropical Andes are a highly degraded ecosystem with less than 30% of their original land cover remanent (Rodríguez Eraso et al., 2013). High elevation alpine ecosystems in the northern Andes (Paramos) have been intensively used for agriculture and low yield cattle grazing, transforming large areas from native paramo vegetation to grasslands dominated by planted exotic grasses or agricultural fields (Ross et al., 2017). Peatlands in the northern Andes are particularly common and are considered areas of low production (Hribljan et al., 2017; Chimner et al., 2019). Thus, peatland areas are usually drained or transformed into productive areas by draining or direct replacement of the vegetation (Marchant et al., 2004; Ross et al., 2017). Changes on the peat forming vegetation of peatlands have important effects on how organic matter is decomposed and on how the gases produced during the decomposition process are transported to the surface (Jones et al., 2019; Bansal et al., 2020).
Peatlands are sensitive to disturbance, particularly mountain peatlands that are usually small and located in specific geomorphic settings (Cooper et al., 2010). Tropical mountain peatlands are minerotrophic, indicating that incoming water comes into contact with the surrounding soil or bedrock before entering the peat and that the surrounding landscape matrix has an effect on the nutrient content and availability (Saarinen et al., 2013; Glina et al., 2019). Human induced disturbances have rapid and drastic effects on the carbon and vegetation dynamics of high elevation peatlands reducing the soil carbon stocks by increasing decomposition rates (Urbina and Benavides, 2015). Small scale disturbances create a positive degradation feedback with increasing decomposition rates on the portions of the peat exposed to the degradation further exposing old peat to changing environmental conditions (Holden, 2005; Urbina and Benavides, 2015). Former land uses can have lasting effects on the carbon accumulation patterns of peatlands, and historic legacies can have an effect even decades after the disturbance have ceased because the active positive feedbacks that started during the active land use (Schimmel et al., 2021).
Methane is produced in peatlands as part of the decomposition process under anaerobic and highly reduced conditions (Wieder et al., 1990; Akhtar et al., 2020). Methane production is inhibited at lower pH mainly due to direct effect on the methanogenesis pathway and at higher pH by the quality of the organic matter (Ye et al., 2012; Girkin et al., 2019). Methane production is mediated by reducing conditions within the soil matrix, the reducing capacity of the soils decreases as different substrates are consumed as electron acceptors in stable anoxic conditions. Thus, a high and stable water table decreases the redox potential of soil until organic carbon is used as electron acceptor producing methane (Lai, 2009). The speed of the process is mediated by temperature that controls the metabolic rate of methanogenic archaea (Sánchez et al., 2017; Zhu et al., 2021).
Tropical Andean and boreal peatlands, to some degree, have similar peat forming species at lower elevations with peatlands dominated by Sphagnum mosses, sedges, true mosses and flood tolerant grasses (Cooper et al., 2010; Benavides, 2013). At higher elevations, a different vegetation type develops dominated by cushion forming plants mostly from the Juncaceae and Plantaginaceae families (Cooper et al., 2010; Planas-Clarke et al., 2020). Rate of methane emission vary with vegetation with low methane emissions from cushion plants (Fritz et al., 2011; Planas-Clarke et al., 2020) and somehow larger emission rates from other vegetation types (Sánchez et al., 2017). The low emission rates are associated to oxygenation in the deeper layers of the peat that transforms stored methane into carbon dioxide before being transported to the surface, this oxygenation is believed to be mostly caused by root-mediated oxygen transport (Jones et al., 2019; Münchberger et al., 2019).
Cushion plants are a unique feature of austral alpine environments (Körner and Kèorner, 1999; Hupp et al., 2017). The roots of the cushion plants have abundant aerenchyma carrying oxygen from the surface to the root tissue up to 50 cm deep and well into the water table (Dullo et al., 2017; Kock et al., 2019; Suárez et al., 2021). The methane that is produced in the deeper anoxic layers has a facilitated transport via the roots of the cushion plants, but the downward oxygen transport oxidizes the methane with almost no methane efflux on the surface (Fritz et al., 2011). However, the peatlands created by the growth of cushion plants have a combination of cushions, hollows, and sites of recent cushion collapse with a spatial variation that is also reflected on the methane fluxes. In our study, methane fluxes in sites with cushion plants were low and similar to what has been reported elsewhere in the Peruvian Andes (Sánchez et al., 2017; Planas-Clarke et al., 2020).
The tropical Andes follow a humidity gradient from south to north, with the northern part of the Andes receiving up to 3,000 mm of rain a year (Cleef, 1981). The northern Andes have had a long history of degradation with nearly 30% of their area transformed for agriculture or cattle grazing affecting peatlands or replacing them with different land cover types (IDEAM and CORMAGDALENA, 2008). Our study aims to understand the patterns of methane emission from tropical alpine peatlands in the northern Andes under contrasting disturbance regimes. Our research questions are aimed to better understand how methane emissions vary among the different disturbance regimes on contrasting Andean peatlands and how microtopography, water table depth and soil temperature the rate of methane emission within the peatland. Our research aims to fill a gap on our understanding on the spatial variation of methane emissions in the tropical Andes and to provide bottom-up estimates of emission factors of greenhouse gasses from high elevation peatlands that can be used on the regional and national greenhouse gas inventories.
2 Materials and methods
2.1 Study sites
Our study took place in four high elevation peatlands in Colombia, two of the peatlands were in the Nevados National Park and two in the Chingaza National Park of Colombia (Figure 1). The four peatlands are fed mostly by superficial runoff and to some degree by groundwater recharge; although, several differences on their climate and geographical location are observed (Table 1, Supplementary Figure S1). Disturbance intensity was determined by direct observation of current grazing, drainage or runoff from cropland. Ecological legacies from historical disturbances were also registered using interviews with local farmers. Disturbances were divided in three main categories: grazing by cattle, runoff from cropland, and land use change. Each category was tallied from 1 to 5 and overall disturbance was obtained by summing the scores of each site across the three categories. Sites were organized from the one with the highest score to the lowest since the summed disturbance index was developed from qualitative data. Disturbance history was different among the four sites: The Calostros site (Chingaza National Park) was heavily disturbed with drainage and current cattle grazing, the surrounding matrix is used for low yield cattle production; the dominant vegetation is Sphagnum sancto-josephense with hummocks of Sphagnum magellanicum, Carex pichinchensis and ericaceous shrubs. The Laguna seca site (Chingaza National Park) has a low disturbance intensity with some drainage observed and historic, not current, cattle grazing; the dominant vegetation is true mosses (Straminergon stramineum, Calliergon spp, Drepanocladus, Sphagnum sancto-josephense) in hollows with hummocks of Cortaderia colombiana and scattered cushions of Plantago rigida (Bosman et al., 1993). The San Antonio site (Nevados National Park) has an intermediate disturbance with active cattle and active drainage management; the dominant vegetation is tall individuals of Cortaderia nitida and Hypericum lycopodiodies with true mosses on the ground layer (Drepanocladus, Straminergum). Finally, the El Cisne site (Nevados National Park) has a low historical disturbance; the vegetation is dominated by cushions of Distichia muscoides surrounded by Calamagrostis effussa grasses and the true moss Sarmentypnum exannulatum in the hollows (Table 1).
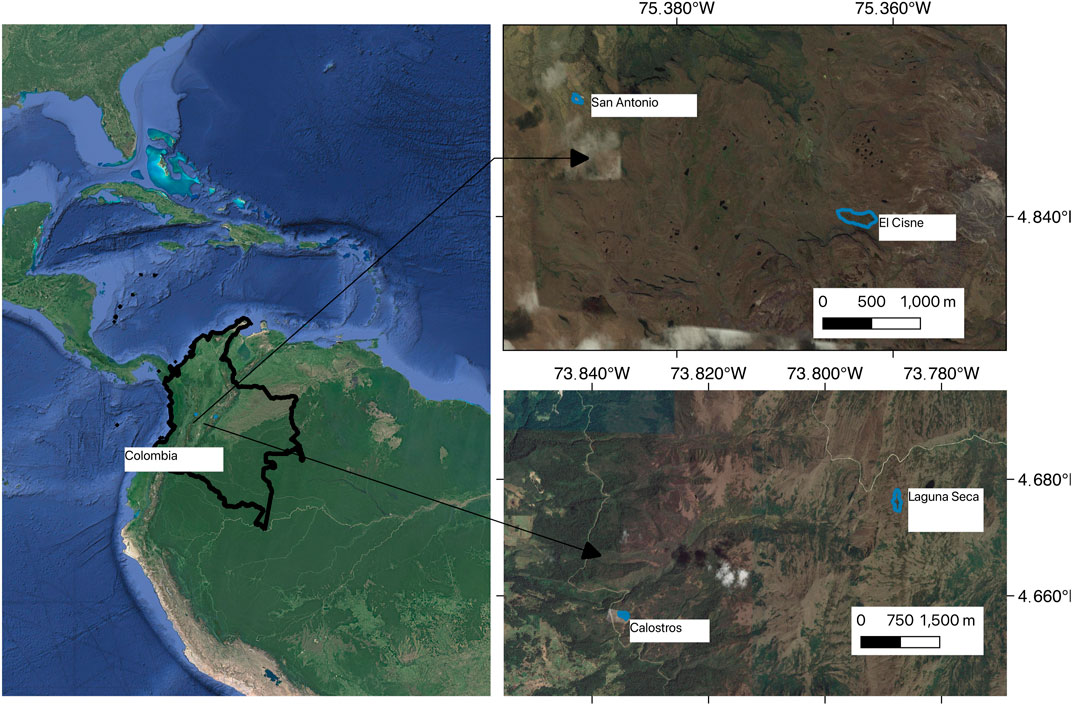
FIGURE 1. Map of the study sites showing the distance between paired sites: Top right: Central Cordillera, San Antonio-intermediate disturbance and El Cisne-Conserved. Bottom right: Eastern cordillera, Calostros-high disturbance, and Laguna Seca-low disturbance.
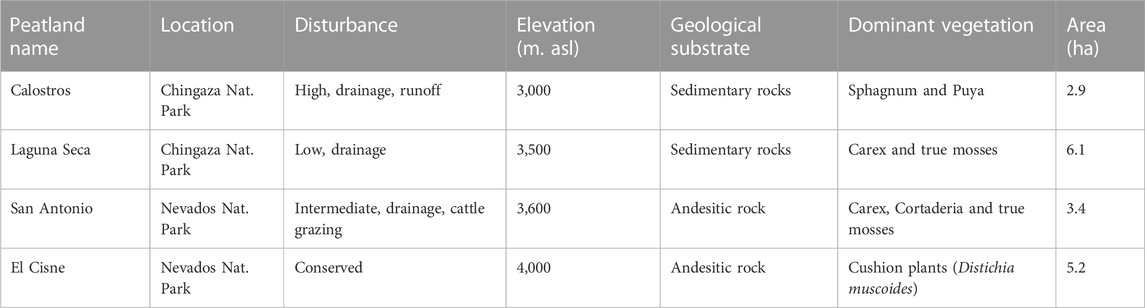
TABLE 1. Dominant vegetation, water chemistry, physical characteristics, and disturbance history of the four selected peatlands evaluated in this study.
Each peatland was mapped according to the main microtopographic features present: cushions of vascular plants, hummocks with Sphagnum and small shrubs, hollows either with open water, exposed soil or hollow vegetation, and sedge lawns. The maps were developed from high resolution (20 cm) drone aerial composite images taken in 2019. The composite images were used for a detailed classification of the main microtopographic features, using a combination of manual and automatic classifiers on the RGB bands.
2.2 Methane flux measurement
We established 12 PVC collars at each site located across the main hydrological gradient observed. The collars were 40 cm in diameter and 10 cm in height and were driven into the ground until a 3–4 cm section remained above the ground. We placed a cylindrical PVC chamber over the collars, the chamber was 40 cm high and with a matching diameter to the collar. The height of the chamber and depth of the collar were set to capture fluxes from soil and water diffusion and plant mediated transport. The chamber had a volume of 0.038 m3 and a footprint of 0.13 m2 with an internal fan and lateral valve for gas collection. Additionally, the chamber was painted white on the outside to prevent heating from solar radiation. Gas samples were collected four times over a 45-min time span at 15-min intervals. The gas samples were collected using a 500 mL air-proof glass syringe. The gas samples were immediately transferred to gas vials and kept in dark cool containers during the day. Methane concentration was analyzed using a portable gas chromatographer (Photovac MicroFID II ®). The analyzer was continuously calibrated using methane dilutions and pure air. When larger concentrations were observed, a new calibration was performed to include the span of the reading. The change in concentration with time was transformed to a relationship of mass by time by area (g CH4 m−2 h−1) using the law of ideal gasses (PPSystems, 2002; Planas-Clarke et al., 2020). We visited the sites at least three times every year with several measurements during the year including the main annual climatic variations from 2015 to 2020. Not all the collars were used at each visit because of disturbance from local fauna, vegetation growth or subsidence.
We installed a monitoring water well every four collars and recorded the water table level at each measurement using the nearest well. We also recorded during each flux measurement the air and soil temperature at 5 cm belowground, air relative humidity, atmospheric pressure, dew point, and overall climatic conditions (sunny, cloudy, drizzle, and rain), and the condition of the ground within the collar (flooded, saturated, moist, and dry) that usually matched the water table at the nearest well. We recorded the microtopographical position of the collar differentiating hollows, hummocks, cushions and sedge tussocks. The upper part of hard cushions of vascular plantas are different from sedge or sphagnum Hummocks with a unique vegetation type that also creates a unique microhabitat. The sedge microhabitat refers to sedge lawns. We installed seven automatic soil temperature sensors under the different microtopographic features for 1 year (2019–2020), recording the soil temperature at 5 cm deep every hour on the Laguna Seca and El Cisne sites (iButton, Maxim Integrated, Inc.).
2.3 Data analysis
We analyzed the relationship between the methane fluxes and basic environmental variables using simple linear models. The independent variables used where: site, soil and air temperature, water table, and dew point. We also run a mixed linear model using the collar as the random factor and nesting the model in the collars since we measured several times each collar during the 6 years of monitoring. The model was selected from a full model describing methane fluxes including the interactions between site with elevation, microhabitat, water table and soil temperature. Model complexity was successively reduced by removing interaction terms first and individual variables later and the best model was selected using the model with the lowest AIC (Anderson and Burnham, 2004). We calculated an annual mass balance of methane emissions for each site by using meteorological data from the nearest station when available or just using the emissions averages for the different microtopographical features for 1 year. We calculated the overall emissions for each site by identifying the areas covered by the different microtopographic features across the peatland area using high resolution imagery. The images were developed from the orthomosaic feature available in the software Agisoft Metashape. The land cover classification was performed using the spatial analyst tool of ArcMap ® and the maximum likelihood method using known control points. All cover classes had a user’s accuracy above 90%. All the statistical analysis were performed on the R statistical software (R Core Team, 2020) with the packages MASS (Venables and Ripley, 2002) and mle4 (Bates et al., 2002). Methane emissions were estimated for each peatland by using the recorded soil temperature data for the year 2019 and using the slope of the relationship between methane fluxes and soil temperature to estimate hourly fluxes for the different microhabitats (Riutta et al., 2007; Webster et al., 2018). The resulting value was multiplied for the area of the microhabitat that was obtained from the high-resolution imagery for each peatland. Source code and raw data is available at https://github.com/jcbenavides/methane1.
3 Results and discussion
Methane emissions were larger at the most disturbed sites with an average of 254 mg CH4 m−2 h−1 followed by the rate of emission at the intermediate low and conserved site with an average of 12 and 5 mg CH4 m−2 h−1 respectively. Methane emissions were largely different between sites and the site with the highest emission rate is also the most disturbed and the lowest elevation site. Differences in vegetation types can influence methane emissions with similar composition of peat forming vegetation between the high, intermediate, and low disturbance sites with a high dominance of Sphagnum mosses and terrestrial bromeliads (Puya spp). The conserved site had a different vegetation dominated by the cushion plants Distichia muscoides and Plantago rigida and was located at a highest elevation (4,000 masl). Another confounding factor is the location with the high and intermediate disturbance located on the eastern cordillera in the Chingaza region, while the low disturbance and conserved sites are in the central cordillera within the Nevados National Park area (Table 1) (Figure 2).
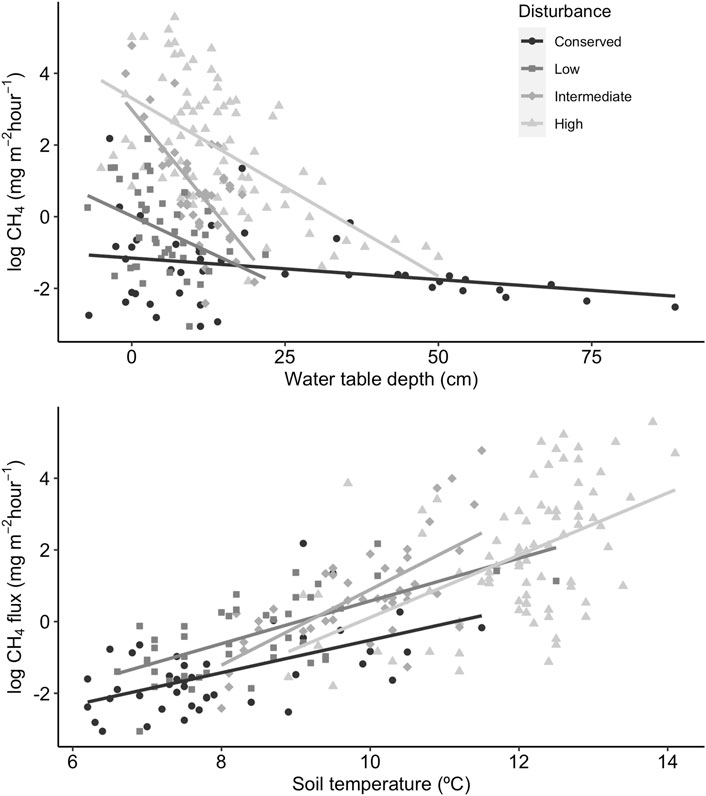
FIGURE 2. Relationship between soil temperature and methane fluxes for the four study sites. Variation of methane flux among the different flooding conditions in the four sites.
The linear model was used after checking the log transformed methane flux data for a normal distribution of the residuals and homogeneity of variances. The linear model selected with the lowest AIC considered the interaction between water table and soil temperature and effectively removed the effect of elevation on the log transformed methane flux (adjR2=0.75, RMSE=0.985). Soil temperature varied among sites with lower mean temperature at the higher elevations (F3,207=118.5, p<0.0001). The conserved and low disturbance sites had lower temperatures than the intermediate and high disturbance sites. Soil temperature between the conserved and the low disturbance site was similar even though the sites have a 400 m difference in elevation. The low disturbance site had a lower temperature than the intermediate disturbance site with both sites occurring almost at the same elevation. The high disturbance site had a higher soil temperature than any other site and was also the site at the lowest elevation (Figure 2). Soil and air temperature was relatively constant during the year with some variation was observed among the different microhabitats. Soil temperature and water level were unrelated but had an interaction effect on the fluxes (F1,207=39.95, p < 0.001).
Methane fluxes were related to depth to the water table with higher emissions at higher water levels (F1,207=66.7, p < 0.001). However, the response of methane emissions to the depth to the water table at the conserved site was weaker than in the other locations indicating that perhaps different mechanisms control methane emissions on the conserved site, that conserved site is also the site dominated by the cushion plants and has the largest variation in water table (Figure 2). Methane emissions were related to soil temperature with similar response to the temperature across the different sites (Figure 2). Soil temperature had a direct effect with the rate of methane emissions across all four sites. Soil temperature was related with elevation but variations within each site were large and the within site variability indicates the strength of the response of methane to the daily variation in soil temperature. At the same time, the intermediate disturbance and the conserved sites had no differences in their mean soil temperature.
The type of disturbances in our four study sites were associated to modification on the land use and not drainage was observed. However, the site with the highest fluxes and more disturbed also has a high variation in its water table. Overall, methane fluxes were related to the depth of the water table. The magnitude of the effect was lower on the site dominated by cushion plants that was also the site with the lowest methane flux. A high water table develops a larger anoxic layer near the surface with labile carbon that facilitates the production of methane from the anaerobic decomposition of organic matter (Turetsky et al., 2014; Sánchez et al., 2017). Fluctuations of the water table also influence the production of methane in peatlands, the exposure of old peat to oxygenic conditions on lower water table exposes organic carbon that is later submerged in the anoxic layers and decomposes anaerobically (Turetsky et al., 2008).
The four sites shared several microtopographical features. Hummocks formed by Sphagnum magellanicum, Cortaderia columbiana, Chusquea tesellata, Agrostis breviculmis and Arcytophyllum nitidum were shared between the sites with low, intermediate, and high disturbance. Hollows with no vegetation or with the sedges Carex pichinchensis, and Carex bomplandi, the mosses Sphagnum sancto-josephense, Drepanocladus aduncus, Scorpidium scorpioides or Straminergum stramineum were found in all sites. Hummocks formed by the cushion plants Distichia muscoides and Plantago rigida were found in the low and intermediate disturbance sites and in the conserved site. We classified the main land cover and microtopography categories based on field observations for each site. The classification was done over 50 cm resolution RGB images (50 cm) and a digital elevation model at that same resolution obtained from the joining of the images in the final orthomosaic. The largest peatland was Laguna Seca with 6.1 ha and none of the peatlands have a combination of the four microtopographic characteristics identified in this study (Table 2) (Figure 3).
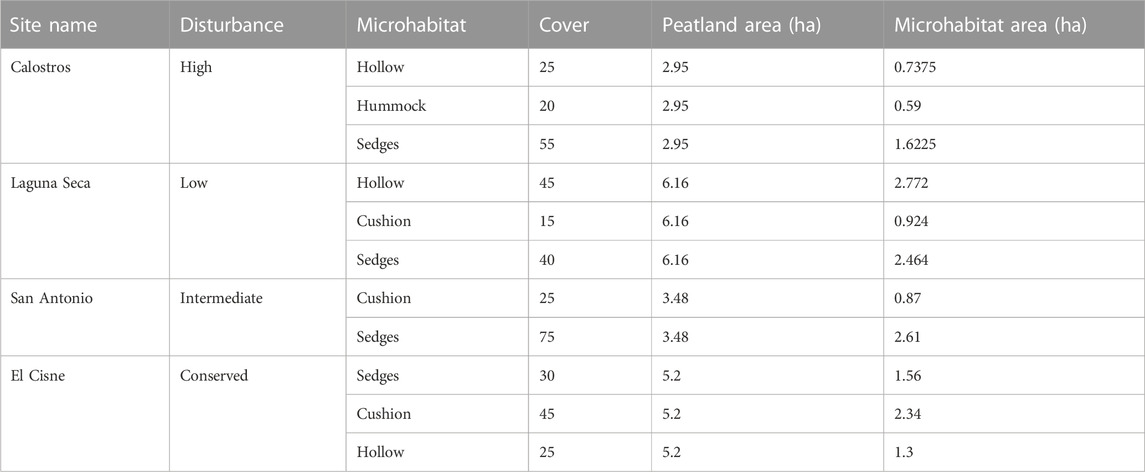
TABLE 2. Distribution of the different microtopographic features in the four Andean peatlands in this study obtained after the classification of high-resolution images.
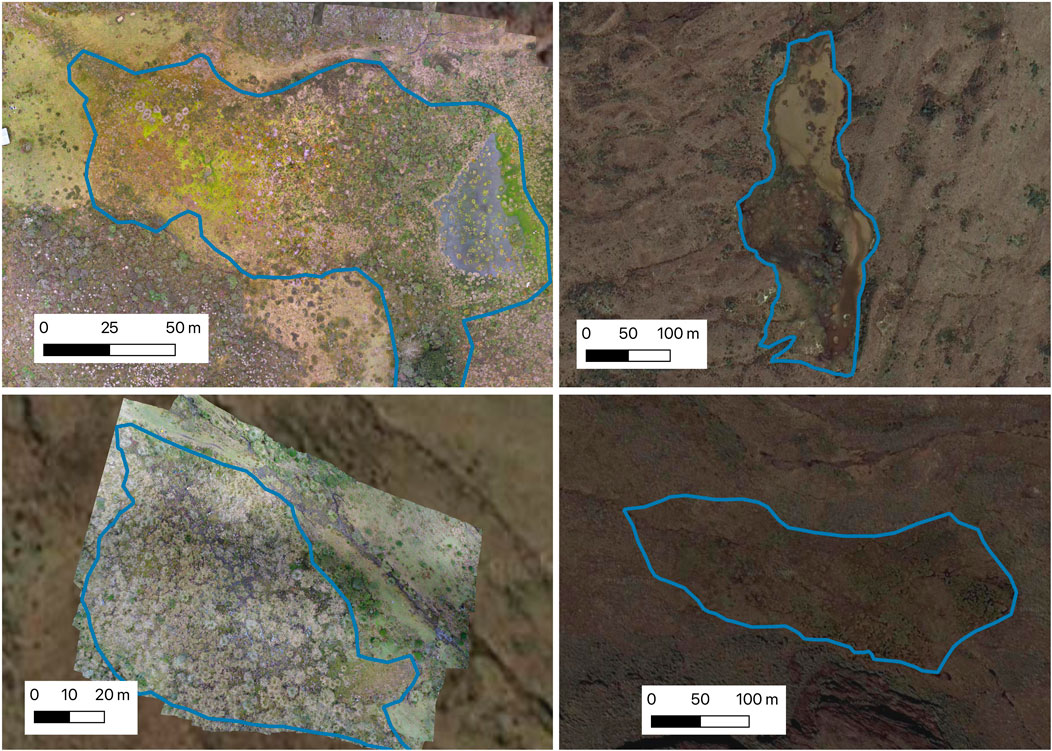
FIGURE 3. High resolution imagery of the four studied peatlands used for the classification of the main microtopographic and vegetation classes. Top left: Calostros-high disturbance, top right Laguna Seca-Low disturbance, bottom left San Antonio Intermediate disturbance, bottom right El Cisne-Conserved.
Our results, for each peatland indicated that although the highly disturbed peatland of Calostros have high emissions, the intermediate disturbance-San Antonio peatland due to its larger area of sedges with a high rate of emissions, had a larger contribution than any of the specific cover types on the disturbed site. The Conserved-El Cisne and low disturbance-Laguna Seca sites have low effluxes of methane on the cushion vegetation. Methane emissions were particularly high in sites dominated by Sphagnum sancto-josephense or open-flooded ground in the hollows. The hummocks dominated by Sphagnum magellanicum and the sedge Carex pichinchensis had lower emissions than the hollows but higher than fluxes from the cushion plants (Figure 4).
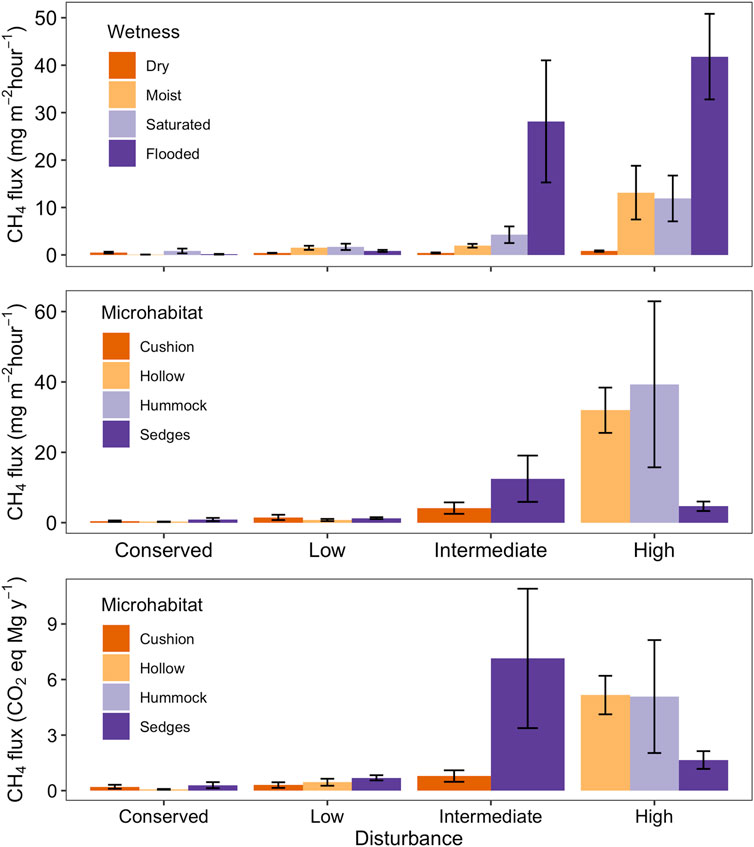
FIGURE 4. Variation of methane emissions from four peatlands across the disturbance gradient in the tropical Andes. Top: CH4 emissions variation related to water table depth categorized in 4 levels, flooded: water table above the ground, saturated: water table between 0 and 5 cm deep, moist: water table level between 5 and 20 cm deep, dry: water table level below 20 cm deep. Center: CH4 emissions relate to microtopography for each site. Bottom: CH4 emissions in CO2 equivalent for the complete area of each peatland detailing the emissions from each microhabitat. Error bars in SD.
The dominant vegetation has a strong influence on methane production and transport to the surface (Strack et al., 2006). Peatlands in the tropical Andes are highly diverse with important variation on plant communities following nutrient, elevation and biogeographical gradients (Benavides and Vitt, 2014; Polk et al., 2019). The higher fluxes were found in sites with sedges either in hollows and hummocks with Sphagnum species. The hummocks formed by cushion plants had very low methane fluxes. Cushion plants are common at higher elevations above 4.000 masl and can transition to Sphagnum or other mosses dominated ecosystems under acidic and low nutrient conditions, transitions to sedge or grass vegetation can also occur. Sedge and Sphagnum dominated peatlands occur at lower elevations and transition from oligotrophic and acidic Sphagnum dominated sites to mesotrophic sites dominated by sedges or grasses (Cooper et al., 2010). Methane emissions on the surface are largely influenced by the transport mechanisms from the anoxic layers to the surface with the root-leaf system of sedges channeling the movement of methane to the surface (Strack et al., 2006). In our study methane production is mainly associated to increased disturbance by grazing where cattle activity promotes decomposition within the anaerobic environment by increasing nutrient availability (Urbina and Benavides, 2015).
Cushion plants in the Andes are a response to adverse climatic conditions at high elevations and in some cases can be considered a response to the camelid herbivory in the Andes (Yager et al., 2019). The distribution of cushion plants is strongly controlled by temperature and elevation with a clear thermal optimum around the line of 4,100 masl in the tropical region that is lower in the southern latitudes (Ruthsatz et al., 2020). Climate change and the associated warmer temperatures have a strong effect on high elevations with temperatures raising faster in the mountains than in the lowlands (Buytaert et al., 2011; Diaz et al., 2014; Fadrique et al., 2018). Plants respond to the changes in temperature by migrating upward and have done it in the past with recent events of Distichia colonization after the middle ages “little ice age” (Benfield et al., 2021). Sedges and other wetland species can colonize sites dominated by Distichia and have done it in the past under warmer conditions. The consequences of a changing vegetation in high elevation wetlands could have larger impacts on the overall emissions of methane gas since sedge dominated peatlands have larger net methane emissions than the current cushion plant vegetation.
4 Conclusion
Wetlands are among the largest biogenic sources of methane (Saunois et al., 2020) due to the prevalence of waterlogged and anoxic conditions (Wieder et al., 1990). The magnitude of the CH4 emissions observed in our study shows a large variation across the disturbance gradient with larger CH4 emissions from disturbed sites. Globally, methane emissions from peatlands vary largely with variations between 0.1 and 358 mg CH4 m−2 day−1 in a boreal system (Waddington and Day, 2007) and between 50 and 300 mg CH4 m−2 day−1 from cushion plants in tropical Andean peatlands in Ecuador (Sánchez et al., 2017; Planas-Clarke et al., 2020). Cushion plants are considered to have low methane emission rates due to different mechanisms including extreme oxygenation on the root aerenchyma of cushions (Fritz et al., 2011), limitation on CH4 production rates by low N availability (Sánchez et al., 2017) or oxygenation of the water saturated area by root transport (Kock et al., 2019; Suárez et al., 2021).
Overall, peatlands are natural sources of methane and although it was considered that Andean peatlands have low emissions those studies were based on measurements from the surface of cushion plants. Our study shows that methane emissions from Andean peatlands are largely affected by human disturbance mostly from changes in hydrology and nutrients in the water (Turetsky et al., 2014). The interaction between warmer climates and warmer soil temperatures induced by climate change, changes in vegetation and methane emissions are still unknown and require further study. Thus, challenges ahead are not only to protect or restore degraded peatlands but to reflect on climate change adaptation mechanisms that will allow for a reduced impact of climate change on the net GHG emissions from peatland ecosystems.
5 Plain language summary
Peatlands are important ecosystems in the global carbon and methane cycles. Previous studies indicated that methane fluxes from mountain peatland in the Andes were low and were mostly associated to cushion plants in the central and southern portions of the Andes. Our study showed that methane emissions are high under disturbances and microtopographical conditions. Methane fluxes were higher when water table was high or above the surface and had a direct relationship with soil temperature. Methane fluxes from our study were higher than in other regions of the Andes and were associated to specific vegetation types and human disturbances. Cushion plants remain as a small source of methane, but higher emissions were observed from the hollows between the cushions, even in undisturbed locations. Sites dominated by sedges and the moss Sphagnum had higher emissions when the water level was high, or the plants were submerged. Our results have implications on the management and restoration of Andean peatlands, since methane fluxes were low even in disturbed peatlands, and peatland restoration involves a rewetting of the peat with subsequent higher methane emissions early in the restoration process. Our study shows that disturbed peatlands are important sources of methane and that restoration activities can reduce methane fluxes on the long term. However, we still lack a clear understanding of the belowground processes associated with methane production and transport on these ecosystems, hampering our ability to better manage them.
Data availability statement
The original contributions presented in the study are included in the article/Supplementary Material, further inquiries can be directed to the corresponding author.
Author contributions
JB designed the experiment and analyzed the data and wrote part of the MS. SR helped writing the MS and building the references database. EB helped with the design of the study and collaborated with the writing and climatological data used in the MS.
Funding
This study was funded by IDEAM—Instituto de Hidrología, Meteorología y Estudios Ambientales that provided funds for field activities and EABT time, The Sustainable Wetlands Adaptation and Mitigation Program (SWAMP).
Acknowledgments
We acknowledge the support of the IDEAM (Instituto de Hidrología, Meteorología y Estudios Ambientales) in Colombia who provided the financial support for this research. We also acknowledge the support of the USFS-SWAMP program who provided the gas chromatographer and calibration gases for this study. We also thank Pontificia Universidad Javeriana for their support and all the students and researchers that participated of the field data collection under the most adverse possible conditions at remote tropical high elevations.
Conflict of interest
The authors declare that the research was conducted in the absence of any commercial or financial relationships that could be construed as a potential conflict of interest.
Publisher’s note
All claims expressed in this article are solely those of the authors and do not necessarily represent those of their affiliated organizations, or those of the publisher, the editors and the reviewers. Any product that may be evaluated in this article, or claim that may be made by its manufacturer, is not guaranteed or endorsed by the publisher.
Supplementary material
The Supplementary Material for this article can be found online at: https://www.frontiersin.org/articles/10.3389/feart.2023.1078830/full#supplementary-material
References
Akhtar, H., Lupascu, M., Sukri, R. S., Smith, T. E., Cobb, A. R., and Swarup, S. (2020). Significant sedge-mediated methane emissions from degraded tropical peatlands. Environ. Res. Lett. 16, 014002. doi:10.1088/1748-9326/abc7dc
Anderson, D., and Burnham, K. (2004). Model selection and multi-model inference. 63. NY: Springer-Verlag, 10.
Bansal, S., Johnson, O. F., Meier, J., and Zhu, X. (2020). Vegetation affects timing and location of wetland methane emissions. J. Geophys. Res. Biogeosciences 125, e2020JG005777. doi:10.1029/2020jg005777
Bates, D., Maechler, M., Bolker, B., and Walker, S. (2002). Fitting linear mixed-effects models using lme4. J. Stat. Softw. 67, 1–48. doi:10.18637/jss.v067.i01
Benavides, J. C. (2013). The changing face of Andean peatlands: The effects of climate and human disturbance on ecosystem structure and function. PhD, South. Ill. UJnviersity.
Benavides, J. C., and Vitt, D. H. (2014). Response curves and the environmental limits for peat-forming species in the northern Andes. Plant Ecol. 215, 937–952. doi:10.1007/s11258-014-0346-7
Benfield, A. J., Yu, Z., and Benavides, J. C. (2021). Environmental controls over Holocene carbon accumulation in Distichia muscoides-dominated peatlands in the eastern Andes of Colombia. Quat. Sci. Rev. 251, 106687. doi:10.1016/j.quascirev.2020.106687
Bosman, A., VAN DER Molen, P., Young, R., and Cleef, A. (1993). Ecology of a paramo cushion mire. J. Veg. Sci. 4, 633–640. doi:10.2307/3236128
Buytaert, W., Cuesta-Camacho, F., and Tobón, C. (2011). Potential impacts of climate change on the environmental services of humid tropical alpine regions. Glob. Ecol. Biogeogr. 20, 19–33. doi:10.1111/j.1466-8238.2010.00585.x
Canadell, J. G., Monteiro, P. M. S., Costa, M. H., Cotrim Da Cunha, L., Cox, P. M., Eliseev, A. V., et al. (2021). “Global carbon and other biogeochemical Cycles and feedbacks,” in MASSON-DELMOTTE. Climate change 2021: The physical Science Basis. Contribution of Working Group I to the Sixth Assessment Report of the Intergovernmental Panel on climate change. Editors P. ZHAI, A. PIRANI, S. L. C. CONNORS, C. PÉAN, S. BERGERet al. (London: Cambridge University Press).
Chimner, R. A., Bourgeau-Chavez, L., Grelik, S., Hribljan, J. A., Clarke, A. M. P., Polk, M. H., et al. (2019). Mapping mountain peatlands and wet meadows using multi-date, multi-sensor remote sensing in the Cordillera Blanca, Peru. Wetlands 39, 1057–1067. doi:10.1007/s13157-019-01134-1
Cleef, A. M. (1981). The vegetation of the páramos of the Colombian Cordillera Oriental. Amsterdam, Netherlands.
Cooper, D. J., Wolf, E. C., Colson, C., Vering, W., Granda, A., and Meyer, M. (2010). Alpine peatlands of the Andes, Cajamarca, Peru. Arct. Antarct. Alp. Res. 42, 19–33. doi:10.1657/1938-4246-42.1.19
Diaz, H. F., Bradley, R. S., and Ning, L. (2014). Climatic changes in mountain regions of the American cordillera and the tropics: Historical changes and future outlook. Arct. Antarct. Alp. Res. 46, 735–743. doi:10.1657/1938-4246-46.4.735
Dullo, B., Grootjans, A., Roelofs, J., Senbeta, A., Fritz, C., and Lamers, L. (2017). Radial oxygen loss by the cushion plant Eriocaulon schimperi prevents methane emissions from an East-African mountain mire. Plant Biol. 19, 736–741. doi:10.1111/plb.12586
Fadrique, B., Báez, S., Duque, Á., Malizia, A., Blundo, C., Carilla, J., et al. (2018). Widespread but heterogeneous responses of Andean forests to climate change. Nature 564, 207–212. doi:10.1038/s41586-018-0715-9
Fritz, C., Pancotto, V. A., Elzenga, J. T., Visser, E. J., Grootjans, A. P., Pol, A., et al. (2011). Zero methane emission bogs: Extreme rhizosphere oxygenation by cushion plants in Patagonia. New Phytol. 190, 398–408. doi:10.1111/j.1469-8137.2010.03604.x
Girkin, N., Vane, C., Cooper, H., Moss-Hayes, V., Craigon, J., Turner, B., et al. (2019). Spatial variability of organic matter properties determines methane fluxes in a tropical forested peatland. Biogeochemistry 142, 231–245. doi:10.1007/s10533-018-0531-1
Glina, B., Piernik, A., Hulisz, P., Mendyk, Ł., Tomaszewska, K., Podlaska, M., et al. (2019). Water or soil—what is the dominant driver controlling the vegetation pattern of degraded shallow mountain peatlands? Land Degrad. Dev. 30, 1437–1448. doi:10.1002/ldr.3329
Holden, J. (2005). Peatland hydrology and carbon release: Why small-scale process matters. Philosophical Trans. R. Soc. A Math. Phys. Eng. Sci. 363, 2891–2913. doi:10.1098/rsta.2005.1671
Hribljan, J. A., Suarez, E., Bourgeau-Chavez, L., Endres, S., Lilleskov, E. A., Chimbolema, S., et al. (2017). Multidate, multisensor remote sensing reveals high density of carbon-rich mountain peatlands in the páramo of Ecuador. Glob. change Biol. 23, 5412–5425. doi:10.1111/gcb.13807
Hupp, N., Llambí, L. D., Ramírez, L., and Callaway, R. M. (2017). Alpine cushion plants have species–specific effects on microhabitat and community structure in the tropical Andes. J. Veg. Sci. 28, 928–938. doi:10.1111/jvs.12553
Ideam, I. G. A. C., and Cormagdalena, (2008). Mapa de Cobertura de la Tierra Cuenca Magdalena-Cauca: Metodología CORINE Land Cover adaptada para Colombia a escala 1:100.000, Bogotá, Colombia. Bogota: Instituto de Hidrología, Meteorología y Estudios Ambientales, Instituto Geográfico Agustín Codazzi y Corporación Autónoma Regional del río Grande de La Magdalena.
Jones, S. P., Diem, T., Arn Teh, Y., Salinas, N., Reay, D. S., and Meir, P. (2019). Methane emissions from a Grassland-wetland Complex in the southern Peruvian Andes. Soil Syst. 3, 2. doi:10.3390/soilsystems3010002
Kock, S. T., Schittek, K., Wissel, H., Vos, H., Ohlendorf, C., Schäbitz, F., et al. (2019). Stable oxygen isotope records (δ18O) of a high-Andean cushion peatland in NW Argentina (24 S) imply South American Summer Monsoon related moisture changes during the Late Holocene. Front. Earth Sci. 7, 45. doi:10.3389/feart.2019.00045
Koffi, E. N., Bergamaschi, P., Alkama, R., and Cescatti, A. (2020). An observation-constrained assessment of the climate sensitivity and future trajectories of wetland methane emissions. Sci. Adv. 6, eaay4444. doi:10.1126/sciadv.aay4444
Körner, C., and Kèorner, C. (1999). Alpine plant life: Functional plant ecology of high mountain ecosystems.
Lai, D. Y. F. (2009). Methane dynamics in northern peatlands: A review. Pedosphere 19, 409–421. doi:10.1016/s1002-0160(09)00003-4
Marchant, R., Behling, H., Berrio, J. C., Hooghiemstra, H., VAN Geel, B., VAN DER Hammen, T., et al. (2004). Vegetation disturbance and human population in Colombia–a regional reconstruction. Antiquity 78, 828–838. doi:10.1017/s0003598x0011347x
Münchberger, W., Knorr, K.-H., Blodau, C., Pancotto, V. A., and Kleinebecker, T. (2019). Zero to moderate methane emissions in a densely rooted, pristine Patagonian bog–biogeochemical controls as revealed from isotopic evidence. Biogeosciences 16, 541–559. doi:10.5194/bg-16-541-2019
Planas-Clarke, A. M., Chimner, R., Hribljan, J., Lilleskov, E., and Fuentealba, B. (2020). The effect of water table levels and short-term ditch restoration on mountain peatland carbon cycling in the Cordillera Blanca, Peru. Wetl. Ecol. Manag. 28, 51–69. doi:10.1007/s11273-019-09694-z
Polk, M. H., Young, K., Cano, A., and León, B. (2019). Vegetation of Andean wetlands (bofedales) in Huascarán national Park, 24. Peru: Mires & Peat.
PPSYSTEMS (2002). “SRC-1 soil Respiration system,” in Operators manual for CIRAS-2 (Haverhill, MA: PP Systems Inc). Version 4.01.
R CORE TEAM (2020). R: A language and environment for statistical computing. Vienna, Austria. URL Available at: https://www.R-project.org/. R Foundation for Statistical Computing.
Riutta, T., Laine, J., Aurela, M., Rinne, J., Vesala, T., Laurila, T., et al. (2007). Spatial variation in plant community functions regulates carbon gas dynamics in a boreal fen ecosystem. Tellus B Chem. Phys. Meteorology 59, 838–852. doi:10.1111/j.1600-0889.2007.00302.x
Rodríguez Eraso, N., Armenteras-Pascual, D., and Alumbreros, J. R. (2013). Land use and land cover change in the Colombian Andes: Dynamics and future scenarios. J. Land Use Sci. 8, 154–174. doi:10.1080/1747423x.2011.650228
Ross, C., Fildes, S., and Millington, A. (2017). Land-use and land-cover change in the Páramo of south-Central Ecuador, 1979–2014. Land 6, 46. doi:10.3390/land6030046
Ruthsatz, B., Schittek, K., and Backes, B. (2020). The vegetation of cushion peatlands in the Argentine Andes and changes in their floristic composition across a latitudinal gradient from 39 S to 22 S. Phytocoenologia 50, 249–278. doi:10.1127/phyto/2020/0374
Saarinen, T., Mohämmädighävam, S., Marttila, H., and Kløve, B. (2013). Impact of peatland forestry on runoff water quality in areas with sulphide-bearing sediments; how to prevent acid surges. For. Ecol. Manag. 293, 17–28. doi:10.1016/j.foreco.2012.12.029
Sánchez, M., Chimner, R., Hribljan, J. A., Lilleskov, E., and Suárez, E. 2017. Carbon dioxide and methane fluxes in grazed and undisturbed mountain peatlands in the Ecuadorian Andes. Mires Peat. 19: art. 20. 18 p., 19.
Saunois, M., Stavert, A. R., Poulter, B., Bousquet, P., Canadell, J. G., Jackson, R. B., et al. (2020). The global methane budget 2000–2017. Earth Syst. Sci. data 12, 1561–1623. doi:10.5194/essd-12-1561-2020
Schimmel, H., Braun, M., Subke, J.-A., Amelung, W., and Bol, R. (2021). Carbon stability in a Scottish lowland raised bog: Potential legacy effects of historical land use and implications for global change. Soil Biol. Biochem. 154, 108124. doi:10.1016/j.soilbio.2020.108124
Strack, M., Waller, M., and Waddington, J. (2006). Sedge succession and peatland methane dynamics: A potential feedback to climate change. Ecosystems 9, 278–287. doi:10.1007/s10021-005-0070-1
Suárez, E., Chimbolema, S., Chimner, R. A., and Lilleskov, E. A. (2021). Root biomass and production by two cushion plant species of tropical high-elevation peatlands in the Andean páramo. Mires Peat 27, 1–9.
Turetsky, M. R., Kotowska, A., Bubier, J., Dise, N. B., Crill, P., Hornibrook, E. R., et al. (2014). A synthesis of methane emissions from 71 northern, temperate, and subtropical wetlands. Glob. change Biol. 20, 2183–2197. doi:10.1111/gcb.12580
Turetsky, M., Treat, C., Waldrop, M., Waddington, J., Harden, J., and Mcguire, A. (2008). Short-term response of methane fluxes and methanogen activity to water table and soil warming manipulations in an Alaskan peatland. J. Geophys. Res. Biogeosciences 113, G00A10. doi:10.1029/2007jg000496
Urbina, J. C., and Benavides, J. C. (2015). Simulated small scale disturbances increase decomposition rates and facilitates Invasive species Encroachment in a high elevation tropical Andean peatland. Biotropica 47, 143–151. doi:10.1111/btp.12191
Venables, W. N., and Ripley, B. D. (2002). Modern Applied Statistics with S. Fourth Edition. New York: Springer.
Waddington, J., and Day, S. (2007). Methane emissions from a peatland following restoration. J. Geophys. Res. Biogeosciences 112. doi:10.1029/2007jg000400
Wang, Y., Paul, S. M., Jocher, M., Espic, C., Alewell, C., Szidat, S., et al. (2021). Soil carbon loss from drained agricultural peatland after coverage with mineral soil. Science of The Total Environment, 149498.
Webster, K., Bhatti, J., Thompson, D., Nelson, S., Shaw, C., Bona, K., et al. (2018). Spatially-integrated estimates of net ecosystem exchange and methane fluxes from Canadian peatlands. Carbon balance Manag. 13, 16–21. doi:10.1186/s13021-018-0105-5
Wieder, R. K., Yavitt, J. B., and Lang, G. E. (1990). Methane production and sulfate reduction in two Appalachian peatlands. Biogeochemistry 10, 81–104. doi:10.1007/bf00002225
Yager, K., Valdivia, C., Slayback, D., Jimenez, E., Meneses, R. I., Palabral, A., et al. (2019). Socio-ecological dimensions of Andean pastoral landscape change: Bridging traditional ecological knowledge and satellite image analysis in Sajama national Park, Bolivia. Reg. Environ. Change 19, 1353–1369. doi:10.1007/s10113-019-01466-y
Ye, R., Jin, Q., Bohannan, B., Keller, J. K., Mcallister, S. A., and Bridgham, S. D. (2012). pH controls over anaerobic carbon mineralization, the efficiency of methane production, and methanogenic pathways in peatlands across an ombrotrophic–minerotrophic gradient. Soil Biol. Biochem. 54, 36–47. doi:10.1016/j.soilbio.2012.05.015
Keywords: Andes (South America), peatlands ecology, methane, disturbance, Sphagnum, Distichia muscoides, sedge
Citation: Benavides JC, Rocha S and Blanco EA (2023) Spatial and temporal patterns of methane emissions from mountain peatlands in the northern Andes across a disturbance gradient. Front. Earth Sci. 11:1078830. doi: 10.3389/feart.2023.1078830
Received: 24 October 2022; Accepted: 18 April 2023;
Published: 09 May 2023.
Edited by:
Jianghua Wu, Memorial University of Newfoundland, CanadaReviewed by:
Ronny Lauerwald, Université Paris-Saclay, FrancePolina Lemenkova, Université libre de Bruxelles, Belgium
Copyright © 2023 Benavides, Rocha and Blanco. This is an open-access article distributed under the terms of the Creative Commons Attribution License (CC BY). The use, distribution or reproduction in other forums is permitted, provided the original author(s) and the copyright owner(s) are credited and that the original publication in this journal is cited, in accordance with accepted academic practice. No use, distribution or reproduction is permitted which does not comply with these terms.
*Correspondence: J. C. Benavides, anViZW5hdmlkZXNAamF2ZXJpYW5hLmVkdS5jbw==