- 1Shanghai Institute of Geological Survey, Shanghai, China
- 2Key Laboratory of Land Subsidence Monitoring and Prevention, MNR, Shanghai, China
Confined groundwater is an indispensable resource for the urban security of Shanghai, China, where multi-layer aquifer structures and human activities create a complex groundwater environment. An understanding of the hydrochemical characteristics and evolutionary mechanisms of groundwater is necessary for its protection and effective utilization and will be explored in this study. A total of 87 groundwater samples were collected from five confined aquifers. Hydrochemistry analysis methods such as Durov diagram, Gibbs model and Saturation index were used to determine the origin and hydrochemical evolution of the confined groundwater. The results show that the samples have two different origins, marine–continental and continental, which have different hydrochemical characteristics. Cl− content of 7.5 meq L−1 was used as a demarcation index for the two origins. The groundwater with a marine–continental-origin is dominated by ancient seawater from which Na+ and Cl− are derived, whereas Ca2+, Mg2+, and HCO3− are derived mainly from carbonate dissolution. Groundwater with a continental-origin is dominated by the effects of water–rock interaction, where major ions are derived mainly from silicate weathering and carbonate dissolution. In both types of groundwater, SO42− is mainly derived from insoluble sulfides that are present in low quantities, whereas SO42− in the few samples with high insoluble sulfide content is derived from human activities. Cation exchange is another controlling factor regarding the hydrochemical composition of groundwater, and water from the two origins have different reaction modes as follows: reverse cation exchange is dominant in marine–continental groundwater, whereas positive cation exchange is more common in continental groundwater. Over the past century, saline water has been flowing into the groundwater funnel region due to human activities, which has resulted in changes in the hydrochemical composition. The recent influx of fresh groundwater and artificial recharge has caused groundwater salinization and mineral re-dissolution.
1 Introduction
Confined groundwater is an important high-quality water resource for domestic and industrial purposes (Zhang et al., 2016), but its over-use causes serious problems. Globally, changes in the hydrochemical composition of groundwater threatens resources in many regions, especially in economically developed delta areas (Gan et al., 2018; Wang et al., 2022). Therefore, an understanding of the hydrochemical origins and controlling factors of groundwater is necessary for its development and protection.
The five confined aquifers in the Shanghai study area developed in an unconsolidated alluvial formation deposited under alternating marine and continental sedimentary environments. There is a weak hydraulic connection between the five aquifers, except where the aquifers are physically connected. Large quantities of high-quality groundwater are confined in Shanghai (Zhang et al., 1999), which has provided an enormous contribution to economic development over recent decades (Jang et al., 2012). However, over-exploitation and artificial recharge have greatly affected the groundwater environment, and its hydrochemical composition has considerably changed in some regions. Increasing attention had been focused on fluctuations in groundwater levels because of the serious land subsidence caused by groundwater exploitation (Huang et al., 2021; Li et al., 2021), but there have been few reports on the hydrochemical characteristics and evolutionary mechanisms of groundwater, and the use and protection of groundwater has received little attention. The origins of groundwater, hydrochemical characteristics, and its classification should be considered in detail to delineate groundwater boundaries and predict saline expansion/contraction (Yang et al., 2018; Li et al., 2022). The Yangtze River Delta is an ideal location to conduct such a study.
The aim of this study was to apply hydrochemical methods in (1) describing groundwater hydrochemical characteristics, 2) identifying sources of groundwater and establishing their demarcation index, 3) identifying the sources of major ions in groundwater, 4) explaining the hydrochemical evolution of the water of different origins, and 5) elucidating the influence of human activities on groundwater. As a result, these methods provide a scientific basis for groundwater utilization and protection planning.
2 Materials and methods
2.1 Study area
The study area is the mainland part of Shanghai in the flat alluvial plain of the Yangtze River Delta at the mouth of the Changjiang River with an area of 5,300 km2 within 120°52′–122°12′E and 30°40′–31°53′N (Figure 1). Quaternary deposits are widely distributed in the area with a thickness of 250–350 m increasing W–E. The aquifers are comprised mainly sand and clay in loose Quaternary sediments and include aphreatic aquifers and five confined aquifers (Figure 2). This study focused on the latter.
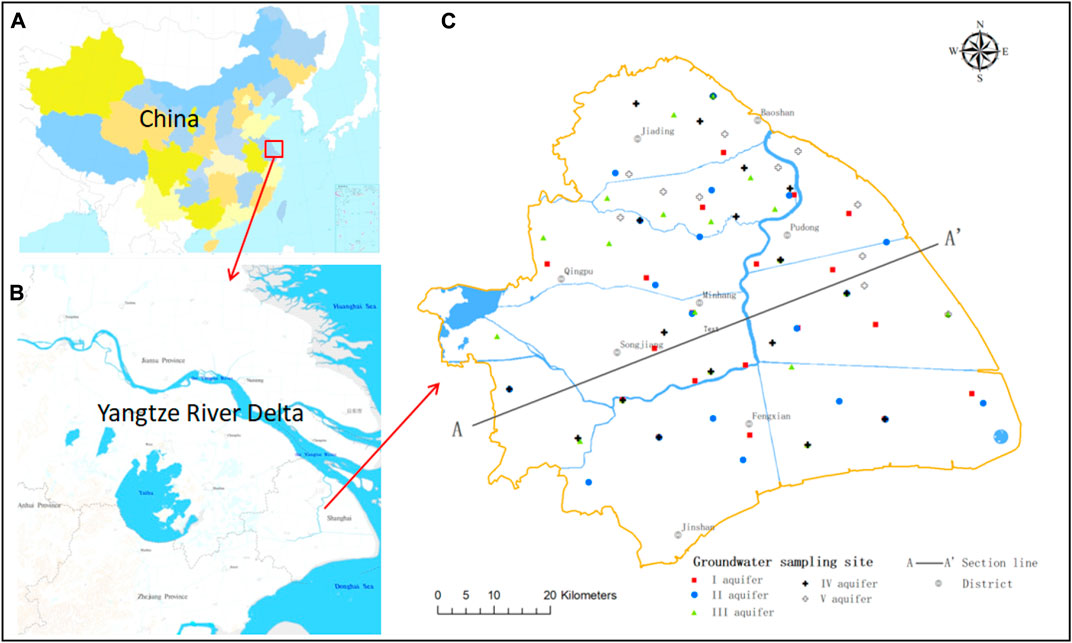
FIGURE 1. Map of the study area showing groundwater sampling points. (A) Map of China. (B) Map of the Yangtze River Delta. (C) Map of Shanghai.
Aquifers I–V were formed during the early to middle–late Pleistocene and their characteristics are shown in Table 1.
The groundwater system in the study area is not a stand-alone system and is part of the Yangtze River Delta system, where confined aquifers are the predominant type. In its natural state, groundwater flows mainly NW–SE. In general, the groundwater is characterized by weak hydrodynamic conditions and a low flow rate. Recharge is mainly through lateral inflow, although artificial recharge has become an important source in recent decades, and discharge is mainly from anthropogenic extraction.
2.2 Sampling and analysis
For sampling, 87 wells were selected to provide a balanced regional distribution. Groundwater samples were collected in September 2018, and 19, 19, 19, 17, and 13 samples were collected from aquifers I–V, respectively (Figure 1).
Prior to filling, all sample bottles were rinsed three times with sample water. Samples for the cation analysis were collected in 500-mL HDPE bottles and then five drops of concentrated nitric acid were added; those for anion analysis were collected in 1000-mL glass bottles without preservatives. All samples were stored on ice in the field and transferred to 4°C storage until ready for analysis.
The concentrations of Na+, K+, Ca2+, Mg2+, Cl−, and SO42− were determined by ion chromatography, HCO3− was determined by acid–base titration, and total dissolved solid (TDS) concentrations were determined using gravimetric analysis (drying at 105 °C). The analysis accuracy was assessed through the ion-balance error of ±5%.
2.3 Durov diagram
The Durov diagram, which is used to study hydrochemical characteristics and facies (An et al., 2014; Gu et al., 2018), comprises two triangles, a central square, and two rectangles (Figure 5). The left and top triangles indicate the concentrations of cations and anions, respectively, the centrals square shows hydrochemical facies, and the right and bottom rectangles indicate TDS concentration and pH, respectively.
2.4 Gibbs model
The Gibbs model is effective in elucidating hydrochemical processes (Gibbs, 1970; Gibbs, 1972). This model is based on groundwater hydrochemical processes being mainly controlled by water–rock interaction, evaporation, and precipitation (Farid et al., 2015; Wang et al., 2022). For this study area, the hydrochemical processes were adjusted to include rock–water interaction, marine-origin, and recharge (including lateral inflow and artificial recharge).
2.5 Saturation index
The saturation index (SI) is used to describe the solubility equilibrium of minerals in water (Rezaei et al., 2005). SI = log(IPA/Ksp), where IPA is the ionic activity product of dissolved mineral constituents and Ksp is the solubility product of the mineral. SI values of 0, >0, and <0 indicate saturated, supersaturated (precipitation may occur), and undersaturated (dissolution may occur) groundwater, respectively.
3 Results and discussion
3.1 Hydrochemical characteristics of confined groundwater
The ionic contents of the studied samples are shown in Table 2. The hydrochemical characteristics of aquifers I and II were generally similar, as were those of aquifers IV and V. The ion contents of aquifer III were the most variable with high standard deviation (SD) values, which indicated that the spatial distribution of groundwater is somewhat variable in terms of hydrochemical composition.
The pH values of groundwater were in the range of 6.99–8.36, 7.06–8.36, 6.92–9.42, 7.30–9.38, and 7.50–9.09 for aquifers I–V, respectively. Aquifers I–III were neutral to slightly alkaline, and aquifers IV–V were slightly alkaline with the pH increasing from aquifers I to V.
TDS concentrations had a range of 246–16522 mg L−1 and decreased from aquifers I to V (Figure 3). All groundwater samples in aquifer I were saline water with TDS >5000 mg L−1 in the west. In aquifer II, 68.4% of the samples were saline, with most in the southwest having TDS >5000 mg L−1. There was a wide range of TDS concentrations in aquifer III, from 250 to 16522 mg L−1, with fresh water predominating in the west and saline water in the east. In aquifers IV and V, most samples were fresh water. There were a few samples with TDS >2000 mg L−1 in the area connecting aquifers III and IV.
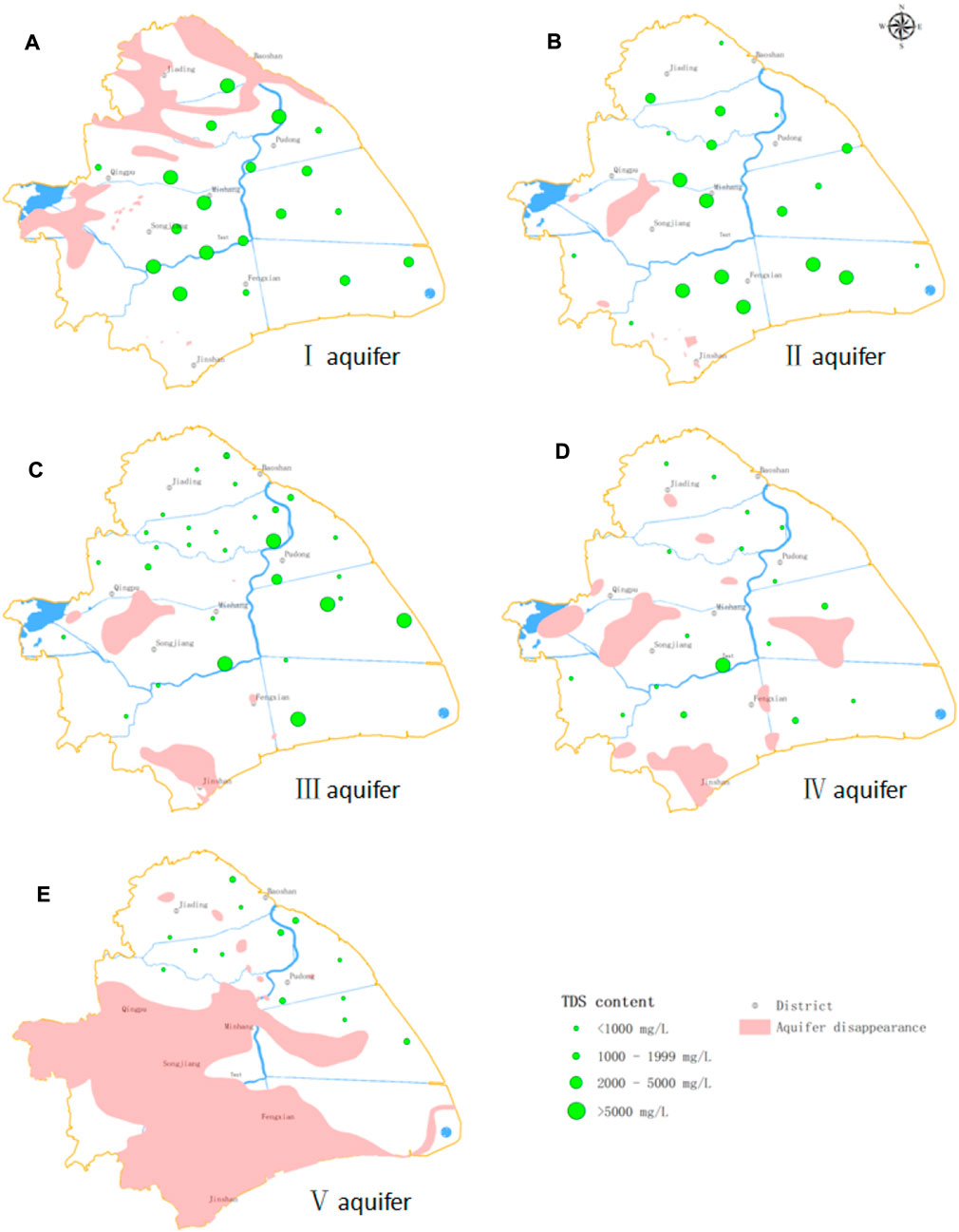
FIGURE 3. Spatial distribution of TDS in the study area. Dot sizes indicate TDS concentrations as follows: the larger the dot, the higher the TDS concentration. (A) TDS concentrations of all samples in aquifer I were >1000 mg L−1 and gradually increase from east to west; saline water was distributed throughout the aquifer. (B) In aquifer II, 68.4% of samples had TDS concentrations >1000 mg L−1; saline water occupies most of the aquifer. (C) In aquifer III, groundwater TDS concentrations were markedly higher in the east than in the west. Additionally, saline water is mainly distributed in the eastern part of the aquifer, whereas fresh water is mainly distributed in the western part of the aquifer. (D) In aquifer IV, most samples have TDS concentrations <1000 mg L−1 with the exception of one sample from the area connecting aquifers III and IV that had TDS = 7010 mg L−1 and another from the same area in aquifer III that had TDS = 7654 mg L−1. We infer that there is a close hydraulic connection between the groundwater of aquifers III and IV in this area. (E) The groundwater of aquifer V is mainly fresh water, but some brackish water with TDS of 1,000–2000 mg L−1 is also present.
The range of concentrations of the major ions are shown in Figure 4. Na+ was the dominant cation with an order of Na+>Ca2+>Mg2+>K+. Of the anions, Cl− had the highest concentrations with clear dominance among all major ions in aquifers I–III. The concentrations of K+, Na+, Ca2+, Mg2+, and Cl− generally decreased from aquifers I to III but had similar concentrations in aquifers IV and V. Most samples had low SO42−and HCO3−concentrations from all five aquifers (Table 2).
3.2 Hydrochemical facies of confined groundwater
The hydrochemical compositions and characteristics of the groundwater samples are indicated by the Durov diagram (Figure 5), where samples from aquifers I and II are concentrated on the Na+ and Cl− sides and those from aquifers III–V are scattered in the upper half of the central square. This indicates that the predominant hydrochemical facies of aquifers I and II are Cl–Na, whereas those of aquifers III–V are variable.
The hydrochemical facies of groundwater can also be described in terms of TDS as follows: for TDS of <1000 mg L−1, hydrochemical facies were variable and included HCO3·Cl–Na, Cl·HCO3–Na, HCO3·Cl–Na·Mg, and Cl·HCO3–Na·Mg, which were mainly distributed in aquifers II–V. For a TDS of 1,000–2000 mg L−1, the hydrochemical facies were Cl–Na, Cl–Na·Ca, and Cl–Na·Mg, and these are distributed mainly in aquifers I, IV, and V. For TDS of 2000–5000 mg L−1, the hydrochemical facies were Cl–Na·Ca and Cl–Na, which were mainly distributed in aquifers I and II. For TDS >5000 mg L−1, the hydrochemical facies were Cl–Na and distributed mainly in aquifers I–III.
3.3 Hydrochemical processes in confined groundwater
The Gibbs diagrams for all groundwater samples are shown in Figure 6. Most samples from aquifers I and II are plotted in the marine-origin domain, whereas those from aquifer III are plotted in both the marine-origin and rock–water-interaction domains, and those from aquifers IV and V are plotted in the rock–water-interaction domain. However, some samples from aquifers IV and V deviate from the Gibbs model (Figure 6A). Therefore, we speculate that other factors may affect the composition of groundwater, which makes Na+ rich and Ca2+ poor. A similar pattern of evolution applies to aquifers I–III as discussed in the following section.
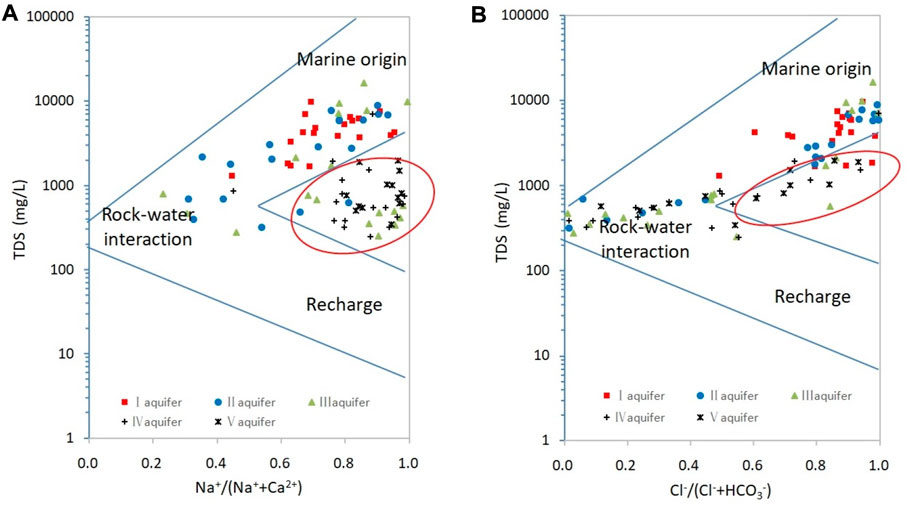
FIGURE 6. Gibbs diagrams for confined groundwater. The red circles indicate samples that deviate from the Gibbs model. (A) Gibbs diagrams of ions. (B) Gibbs diagrams of anions.
Quaternary strata studies (Qiu and Li., 2007) indicate that there were seven marine transgressions in Shanghai during the Quaternary period with aquifers I–III formed during transgression periods. The presence of groundwater of marine–continental- and continental-origin in the study area was verified. The distribution of the former may account for most of aquifers I and II, and the eastern portion of aquifer III. The latter applies to the remaining aquifers, including small portions of aquifers II and III and most of aquifers IV and V. Further evidence is provided in the following section based on an ion-ratio analysis.
3.4 Sources of ions in confined groundwater
The Quaternary sediments in the study area are comprised sand and clay. X-ray diffraction and electron microscopy data for these sediments (Qiu and Li., 2007) indicate that the diagenetic minerals in the aquifers are predominantly silicate (quartz, feldspar, kaolinite, and illite) and carbonate (calcite and dolomite) rocks. Rock weathering and dissolution are naturally controlled by the hydrochemical composition of groundwater (Bau et al., 2004; Kim, 2010) through typical reactions as follows:
Silicate dissolution:
Carbonate dissolution:
Halite dissolution:
Ancient seawater is an important source of Na+ and Cl− in the groundwater of marine–continental-origin. The Na+/Cl− equivalence ratio in seawater is 0.87 (Kunwar and Kawamura, 2014). A ratio of <1 indicates that the groundwater is affected mainly by seawater, whereas ratios of >1 indicate that silicate dissolution is more important (Eq. 1) and a ratio of 1 indicates that halite dissolution is the primary source of these ions (Eqs 4, 5; Gianguzza et al., 2004; Panno et al., 2006).
Bivariate plots for Na+ and Cl− are shown in Figures 7A–B, which shows two groups of samples separated by the Cl− = 7.5 meq L−1 line (Figure 7B). Samples from aquifers I and II are distributed mainly below the 0.87:1 line with Cl− >7.5 meq L−1, and samples from aquifers IV and V are distributed mainly above the 1:1 line with Cl− <7.5 meq L−1. Therefore, the Cl− = 7.5 meq L−1 line acts as a demarcation index to identify groundwater origins in the study area. Some samples from the aquifer V plot near the 1:1 line with Cl− >7.5 meq L−1 is due to halite dissolution rather than seawater. Using the demarcation index of Cl− = 7.5 meq L−1, it was determined that the proportions of continental-origin groundwater samples in aquifers I–V were 0%, 26.3%, 52.6%, 70.6%, and 84.6%, respectively.
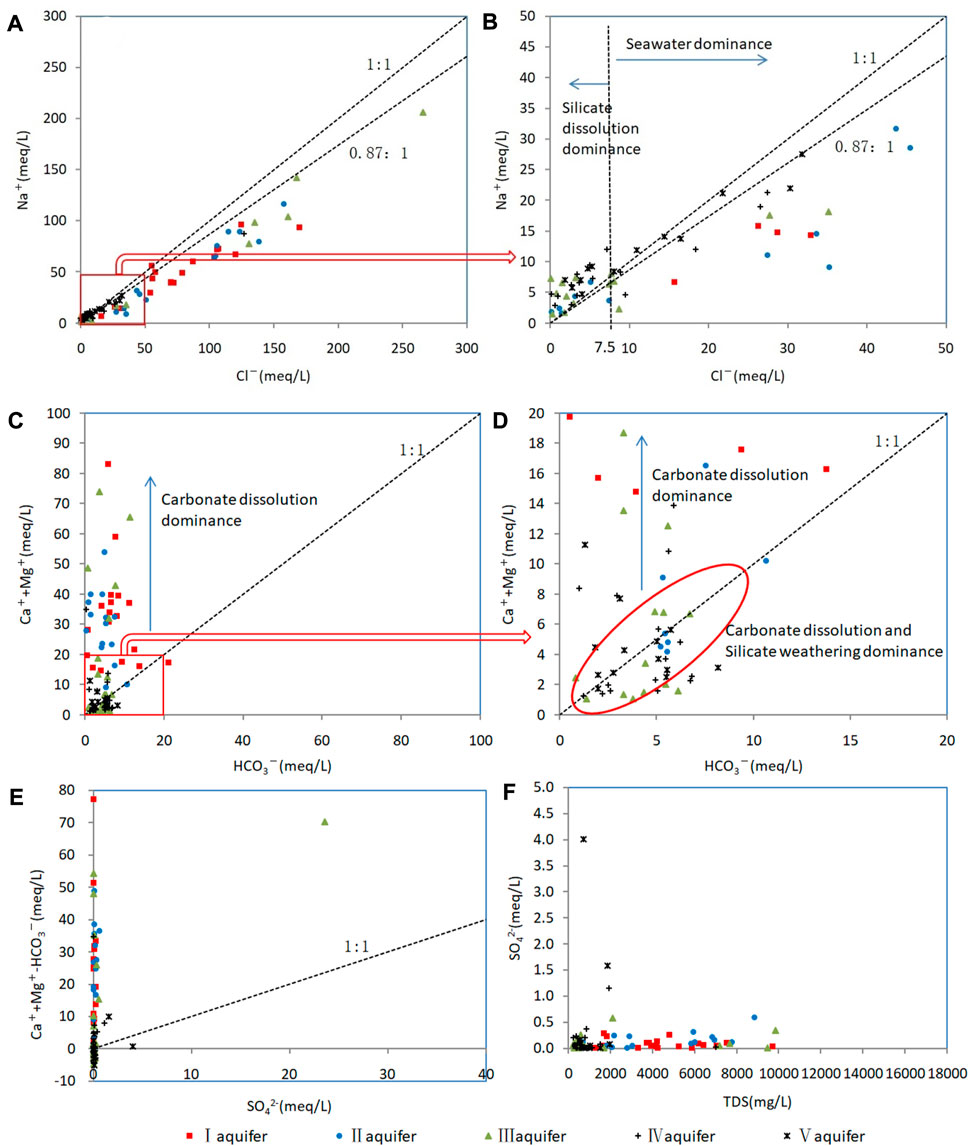
FIGURE 7. Relationships between ion contents of confined groundwater samples. (A) Relationship between Na+ and Cl− for all samples. (B) Relationship between Na+ and Cl− for samples with Na+ and Cl− concentration of <50 meq L−1. (C) Relationship between (Mg2++Ca2+) and HCO3− for all samples. (D) Relationship between (Mg2++Ca2+) and HCO3− for samples with (Mg2++Ca2+) and HCO3− concentration of <20 meq L−1. (E) Relationship between (Mg2++Ca2+–HCO3−) and SO42− for all samples. (F) Relationship between SO42− and TDS for all samples.
Carbonate dissolution and silicate weathering are the primary sources of Ca2+, Mg2+, and HCO3− as indicated by the (Mg2++Ca2+)/HCO3− ratio (Kenoyer and Bowser, 1992; Kim, 2010): a ratio of <1 indicates that Ca2+ and Mg2+ were derived primarily from silicate weathering, and ratios of ≤1 indicate they were derived primarily from carbonate dissolution.
Most samples from aquifers I and II and the east part of aquifer III have (Mg2++Ca2+)/HCO3− ratios of >1 (Figure 7C), which suggests carbonate dissolution as a source of Ca2+ and Mg2+. Most samples from aquifers IV and V and the west part of aquifer III have ratios of ≤1, which reflects the predominant contributions of silicate weathering and carbonate dissolution. Calcite and dolomite reach saturation or oversaturation in most samples (SI >−0.5; Figures 10C, D), which indicates that carbonate dissolution occurred throughout geological history. In summary, carbonate dissolution made the greatest contribution to marine–continental-origin groundwater, whereas both silicate weathering and carbonate dissolution contributed to continental-origin groundwater.
In the absence of anthropogenic sources, SO42− is derived mainly from evaporite deposits, such as gypsum, through hydrochemical reactions as follows:
The SO42− content of most samples was low (Figure 7F), and the correlations between (Mg2++Ca2+−HCO3−) and SO42− were poor (Figure 7E). Furthermore, most samples were undersaturated in gypsum (SI <−2; Figure 10A). Confined aquifers in the study area have excellent sealing properties, low mobility, and long retention times, so evaporite deposits such as gypsum are at low levels and SO42− is mainly derived from insoluble sulfides (Lang et al., 2011), although the high SO42− contents of some samples are attributable to anthropogenic activity.
3.5 Cation exchange
In terms of hydrochemical processes and ion sources, Ca2+, Mg2, and Na+ deviated slightly from the standard model, which implied that the groundwater was affected by other factors. Cation exchange commonly influences the evolution of groundwater composition (Tournassat et al., 2009; Zheng et al., 2021) through typical reactions as follows:
The reactions on the right are positive cation exchange, and those to the left are reverse cation exchange. The relationship between (Mg2++Ca2+−HCO3−−SO42−) and (Na++K+−Cl−) indicates the mechanism of cation exchange (Figure 8).
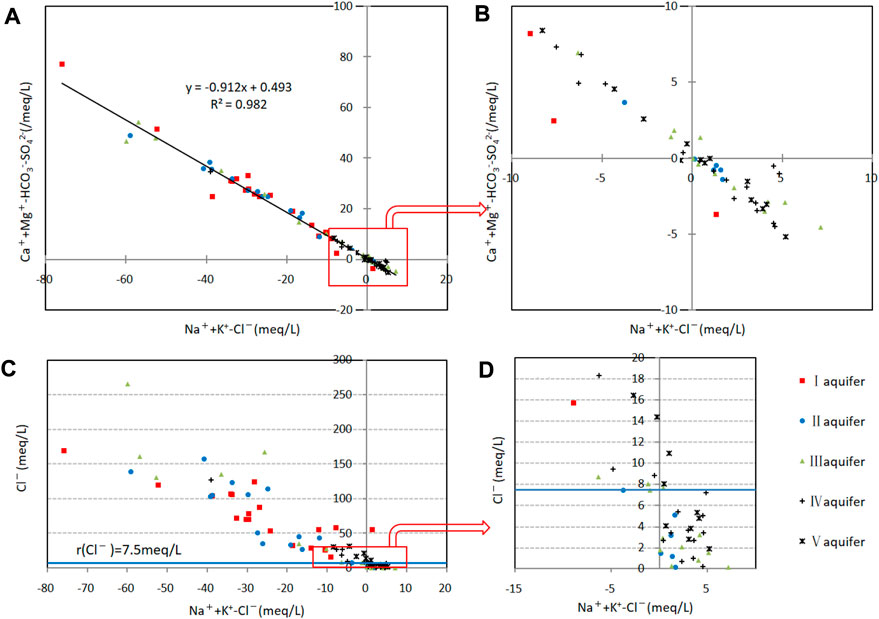
FIGURE 8. Cation-exchange analysis diagram of confined groundwater. (A) Relationship between (Mg2++Ca2+–HCO3−–SO42−) and (Na++K+–Cl−) for all samples. (B) Relationship between (Mg2++Ca2+–HCO3−–SO42−) and (Na++K+–Cl−) for samples with low concentrations. (C) Relationship between Cl− and (Na++K+–Cl−) for all samples. (D) Relationship between Cl− and (Na++K+–Cl−) for samples with low concentrations.
Changes in (Mg2++Ca2+−HCO3−−SO42−) and (Na++K+−Cl−) for the samples were opposite with a correlation coefficient of 0.98, which indicated a strong negative correlation. In general, the anion changes (Cl−, HCO3−, SO42−) in the groundwater were not significant, so this negative correlation mainly reflects (Ca2+, Mg2+) and (Na+, K+). A clear relationship between (Ca2+, Mg2+) and (Na+, K+) is thus noted with cation exchange playing a major role in controlling the hydrochemical compositions of groundwater.
The direction of cation exchange depends on the ionic adsorption energy and concentration. The energy decreases in the order of Ca2+>Mg2+>K+>Na+, so positive cation exchange is more common and leads to an increase in Na+ and K+ and a decrease in Ca2+ and Mg2+ concentrations in groundwater. However, 61% of the samples in the study area exhibited reverse cation exchange with negative (Na++K+−Cl−) and positive (Mg2++Ca2+−HCO3−−SO42−) values.
Reverse cation exchange is more common in water with Cl− content of >7.5 meq L−1 (Figures 8C, D), whereas positive cation exchange is predominant with Cl− <7.5 meq L−1. This is consistent with the demarcation index for groundwater origins (Section 3.4). Reverse cation exchange is more significant in marine–continental-origin groundwater, and positive cation exchange is more significant in continental-origin groundwater with cation exchange explaining the deviation of some samples from the Gibbs model and why the Na+/Cl− ratio is below the 0.87:1 line.
3.6 Anthropogenic activity and hydrochemical evolution
As a drainage area of the Yangtze River Delta, aquifers in the study area have received freshwater recharge throughout geological history through lateral inflow, which compresses saline water within a certain range and limits its expansion and forms stable boundaries. However, boundaries and hydrochemical characteristics have changed over the past century due to groundwater exploitation.
Annual groundwater use exceeded 200 million m3 in 1963, but it is currently decreasing each year. During the heavy-use periods, groundwater funnels develop due to excessive use, and saline water flows into the fresh water. Thus, continental-origin groundwater becomes marine–continental-origin groundwater (Figure 9). For example, saline water from the east flowed into a groundwater funnel to the northwest of Qingpu in aquifer III.
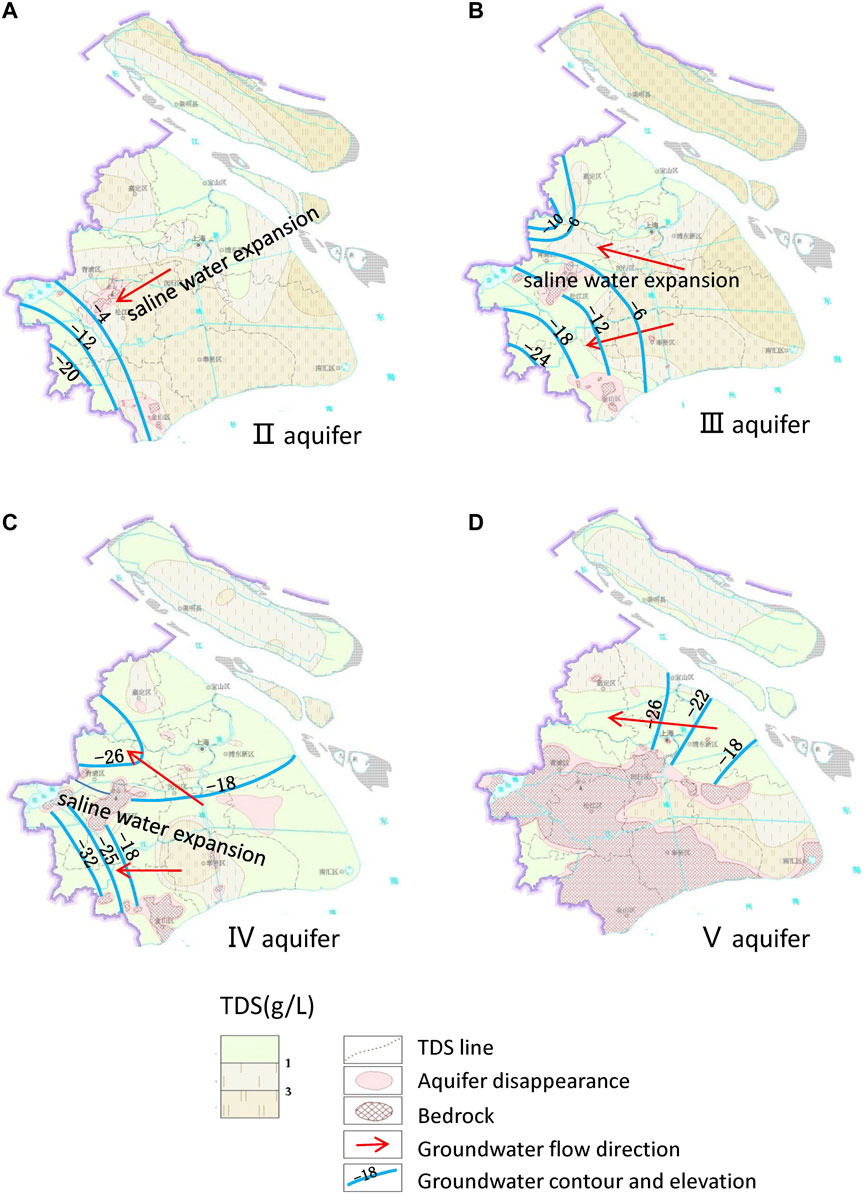
FIGURE 9. TDS and groundwater levels in the study area in 2009. TDS data are derived from Wei et al. (2010). Groundwater levels and flow directions are derived from Gong, (2009). (A) Groundwater funnels in aquifer II were mainly located in Songjiang in the southwest of the study area. (B) Groundwater funnels in aquifer III were mainly located in Songjiang and Qinpu in the western part of the study area. (C) Groundwater funnels in aquifer IV are similar to those in aquifer III. Saline recharge from aquifer III to aquifer IV in the area connecting the aquifers has been exacerbated by a decline in groundwater levels in aquifer IV. (D) Groundwater generally flowed from east to west due to the decline in groundwater levels in the west part of aquifer V.
The expansion of saline water due to human activities over the past century is widespread and has serious implications for freshwater resources. Fortunately, Shanghai has adopted strict controls on groundwater exploitation with annual use limited to 2 million m3. Artificial recharge has also been implemented. During the 13th Five Year Plan period, the annual artificial recharge was ∼20 million m3 and through this the groundwater level rises each year. However, artificial recharge has caused other problems, notably, desalination and mineral re-dissolution.
Saturation indices were calculated with respect to gypsum, aragonite, calcite, and dolomite in groundwater and are plotted in Figure 10. It is generally considered that groundwater is saturated at SI values of −0.5 to +0.5 (Liu, 2019). Most samples were supersaturated or saturated in aragonite, calcite, and dolomite (SI >−0.5; Figures 10B–D), which is consistent with weak hydrodynamic conditions in the study area. However, a few samples had SI values of <−0.5, which is possibly because of the influx of fresh groundwater and artificial recharge and resulted in the breakdown of hydrochemical equilibrium and renewed mineral dissolution (Kanagaraj and Elango, 2019).
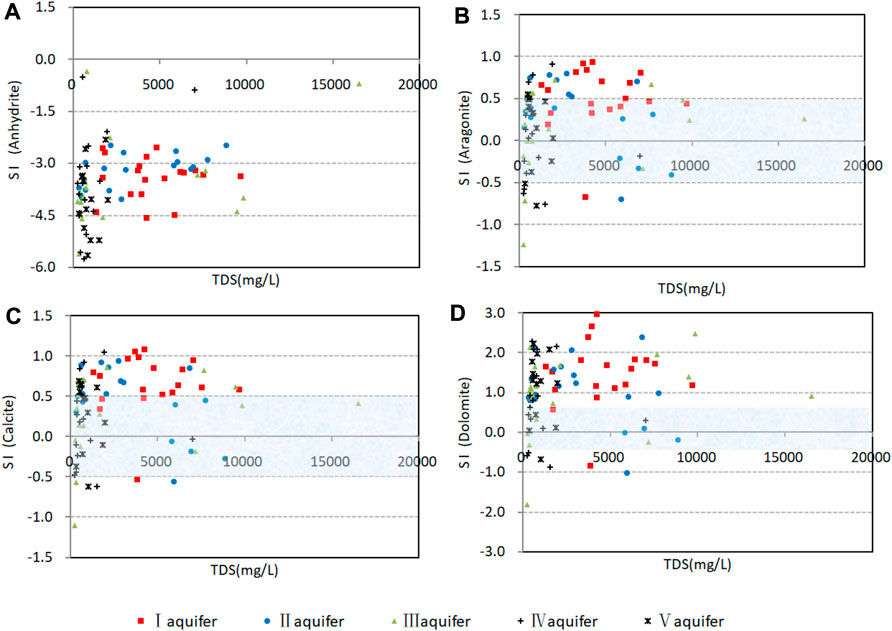
FIGURE 10. Saturation indices of confined groundwater in the study area. (A) Saturation indices values for anhydrite. (B) Saturation indices values for aragonite. (C) Saturation indices values for calcite. (D) Saturation indices values for dolomite.
4 Conclusion
The hydrochemical characteristics of groundwater were studied and trends in the study area were identified. These findings are valuable for planning groundwater protection and utilization in Shanghai.
Hydrochemical characteristics are dominated by the origin of groundwater. Two groundwater origins were identified: marine–continental- and continental-origin. For marine–continental-origin groundwater, major ions are primarily derived from ancient seawater and carbonate dissolution, and reverse cation exchange is common due to high concentrations of Na. Silicate weathering, carbonate dissolution, and positive cation exchange predominantly contribute to the hydrochemical composition of continental-origin groundwater.
TDS contents of 1,000 mg L−1 and hydrochemical facies do not accurately identify the origins of groundwater, but Cl− content of 7.5 meq L−1 acts as a demarcation index for the two origins. This index can be used to determine the boundaries between the groundwater of the two origins and allows accurate monitoring and prediction of the advance/retreat of saline water.
We mainly focused on the relationship between groundwater and human activities, which is unavoidable in Shanghai. The study demonstrates that geogenic processes are not the only mechanisms controlling groundwater chemistry; anthropogenic activities also affect groundwater chemistry, and once the evolution of groundwater is disturbed, then recovery is difficult.
Data availability statement
The original contributions presented in this study are included in the article/Supplementary Material, and further inquiries can be directed to the corresponding author.
Author contributions
All authors listed have made a substantial, direct, and intellectual contribution to the work and approved it for publication.
Funding
This research was supported by a special project on Asian cooperation (Comparative study of geo-environment and geohazards in the Yangtze River Delta and Red River Delta) and a study of three-dimensional geological models and environmental physical fields of middle–shallow underground space.
Conflict of interest
The authors declare that the research was conducted in the absence of any commercial or financial relationships that could be construed as a potential conflict of interest.
Publisher’s note
All claims expressed in this article are solely those of the authors and do not necessarily represent those of their affiliated organizations, or those of the publisher, the editors, and the reviewers. Any product that may be evaluated in this article, or claim that may be made by its manufacturer, is not guaranteed or endorsed by the publisher.
References
An, T. D., Tsujimura, M., Phu, V. L., Kawachi, A., and Ha, D. T. (2014). Chemical characteristics of surface water and groundwater in coastal watershed, mekong delta, vietnam. Environ. Sci. 20, 10. doi:10.1016/j.proenv.2014.03.085
Bau, M., Alexander, B., Chesley, J. T., Dulski, P., and Brantley, S. L. (2004). Mineral dissolution in the cape cod aquifer, Massachusetts, USA: I. Reaction stoichiometry and impact of accessory feldspar and glauconite on strontium isotopes, solute concentrations, and REY distribution. Geochimica Cosmochimica Acta 68 (6), 1199–1216. doi:10.1016/j.gca.2003.08.015
Farid, I., Zouari, K., Rigane, A., and Beji, R. (2015). Origin of the groundwater salinity and geochemical processes in detrital and carbonate aquifers: Case of chougafiya basin (central Tunisia). J. Hydrology 530, 508–532. doi:10.1016/j.jhydrol.2015.10.009
Gibbs, R. J. (1970). Mechanisms controlling world water chemistry. Science 170 (3962), 1088–1090. doi:10.1126/science.170.3962.1088
Gibbs, R. J. (1972). Water chemistry of the amazon river. Geochimica Cosmochimica Acta 36 (9), 1061–1066. doi:10.1016/0016-7037(72)90021-x
Gan, Y., Zhao, K., Deng, Y., Liang, X., Ma, T., and Wang, Y. (2018). Groundwater flow and hydrogeochemical evolution in the jianghan plain, central China. Hydrogeology J. 26 (5), 1609–1623. doi:10.1007/s10040-018-1778-2
Gianguzza, A., Milea, D., Millero, F. J., and Sammartano, S. (2004). Hydrolysis and chemical speciation of dioxouranium(vi) ion in aqueous media simulating the major ion composition of seawater. Mar. Chem. 85 (3-4), 103–124. doi:10.1016/j.marchem.2003.10.002
Gong, S. L. (2009). Change of groundwater seepage field and its influence on the development of land subsidence in shanghai. Scispace 20 (3), 1–5. doi:10.3969/j.issn.1007-1903.2009.01.001
Gu, X., Xiao, Y., Yin, S., Hao, Q., Liu, H., Hao, Z., et al. (2018). Hydrogeochemical characterization and quality assessment of groundwater in a long-term reclaimed water irrigation area, north China plain. Water 10 (9), 1209. doi:10.3390/w10091209
Huang, S., Yin, Y., Sun, R., and Tan, X. (2021). In situ anaerobic bioremediation of petroleum hydrocarbons in groundwater of typical contaminated site in shanghai, China: A pilot study. Environ. Eng. Sci. 38, 1052–1064. doi:10.1089/ees.2020.0534
Jang, C. S., Liu, C. W., and Chou, Y. L. (2012). Assessment of groundwater emergency utilization in taipei basin during drought. J. Hydrology 414, 405–412. doi:10.1016/j.jhydrol.2011.11.016
Kanagaraj, G., and Elango, L. (2019). Chromium and fluoride contamination in groundwater around leather tanning industries in southern India: Implications from stable isotopic ratio δ 53 cr/δ 52 cr, geochemical and geostatistical modelling. Chemosphere 220, 943–953. doi:10.1016/j.chemosphere.2018.12.105
Kenoyer, G. J., and Bowser, C. J. (1992). Groundwater chemical evolution in a sandy silicate aquifer in northern Wisconsin: 1. Patterns and rates of change. Water Resour. Res. 28 (2), 579–589. doi:10.1029/91WR02302
Kim, K. (2010). Plagioclase weathering in the groundwater system of a sandy, silicate aquifer. Hydrol. Process. 16 (9), 1793–1806. doi:10.1002/hyp.1081
Kunwar, B., and Kawamura, K. (2014). One-year observations of carbonaceous and nitrogenous components and major ions in the aerosols from subtropical okinawa island, an outflow region of Asian dusts. Atmos. Chem. Phys. 14 (4), 1819–1836. doi:10.5194/acpd-13-22059-2013
Lang, Y. C., Liu, C. Q., Li, S. L., Zhao, Z. Q., and Zhou, Z. H. (2011). Tracing natural and anthropogenic sources of dissolved sulfate in a karst region by using major ion chemistry and stable sulfur isotopes. Appl. Geochem. 26, S202–S205. doi:10.1016/j.apgeochem.2011.03.104
Li, C., Men, B-H., and Yin, S-Y. (2022). Analysis of groundwater chemical characteristics and spatiotemporal evolution trends of influencing factors in southern beijing plain. Front. Environ. Sci. 10, 913542. doi:10.3389/fenvs.2022.913542
Li, M. G., Chen, J. J., Xu, Y. S., Tong, D. G., and Shi, Y. J. (2021). Effects of groundwater exploitation and recharge on land subsidence and infrastructure settlement patterns in shanghai. Eng. Geol. 282, 105995. doi:10.1016/j.enggeo.2021.105995
Liu, C. W., and Wu, M. Z. (2019). Geochemical, mineralogical and statistical characteristics of arsenic in groundwater of the lanyang plain, taiwan. J. Hydrology 577, 123975. doi:10.1016/j.jhydrol.2019.123975
Panno, S. V., Hackley, K. C., Hwang, H. H., Greenberg, S., Krapac, I., Landsberger, S., et al. (2006). Characterization and identification of na-cl sources in ground water. Ground water 44 (2), 176–187. doi:10.1111/j.1745-6584.2005.00127.x
Qiu, J. B., and Li, X. (2007). Quaternary Strata and sedimentary Environment in Shanghai. Shanghai Chian: Shanghai Scientific and TechnicalPublishers.
Rezaei, M., Sanz, E., Raeisi, E., Ayora, C., Vazquez-Sune, E., and Carrera, J. (2005). Reactive transport modeling of calcite dissolution in the fresh-salt water mixing zone. J. Hydrology 311 (1-4), 282–298. doi:10.1016/j.jhydrol.2004.12.017
Tournassat, C., Gailhanou, H., Crouzet, C., Braibant, G., Gautier, A., and Gaucher, E. C. (2009). Cation exchange selectivity coefficient values on smectite and mixed-layer illite/smectite minerals. Soil Sci. Soc. Am. J. 73 (3), 928–942. doi:10.2136/sssaj2008.0285
Wang, S., Xie, Z., Wang, F., Zhang, Y., Wang, W., Liu, K., et al. (2022). Geochemical characteristics and quality appraisal of groundwater from Huatugou of the Qaidam Basin on the Tibetan plateau. Front. Earth Sci. 10, 874881. doi:10.3389/feart.2022.874881
Wei, Z. X., Zai, G. Y., and Yan, X. X. (2010). Atlas of Shanghai urban Geology. China: Geological Publishing House.
Yang, N., Wang, G., Shi, Z., Zhao, D., Jiang, W., Guo, L., et al. (2018). Application of multiple approaches to investigate the hydrochemistry evolution of groundwater in an arid region: Nomhon, northwestern China. Water 10 (11), 1667. doi:10.3390/w10111667
Zhang, X., Hui, Q., Wu, H., Chen, J., and Qiao, L. (2016). Multivariate analysis of confined groundwater hydrochemistry of a long-exploited sedimentary basin in northwest China. J. Chem. 2016, 1–15. doi:10.1155/2016/3812125
Zhangh, W., Qin, X., and Yi, L. (1999). Groundwater system and ITS characteristics in Shanghai region. Carsologica Sin. 1999(4), 343–351. doi:10.3969/j.issn.1001-4810.1999.04.007
Keywords: hydrochemistry, groundwater origin, Shanghai, water–rock interaction, ion source
Citation: Zhan G, Li J, Wang H, Wen X and Gu H (2023) Origin and hydrochemical evolution of confined groundwater in Shanghai, China. Front. Earth Sci. 11:1117132. doi: 10.3389/feart.2023.1117132
Received: 06 December 2022; Accepted: 13 March 2023;
Published: 31 March 2023.
Edited by:
Jian Liu, Qingdao Institute of Marine Geology (QIMG), ChinaReviewed by:
Maosheng Gao, Qingdao Institute of Marine Geology (QIMG), ChinaTianyuan Zheng, Ocean University of China, China
Copyright © 2023 Zhan, Li, Wang, Wen and Gu. This is an open-access article distributed under the terms of the Creative Commons Attribution License (CC BY). The use, distribution or reproduction in other forums is permitted, provided the original author(s) and the copyright owner(s) are credited and that the original publication in this journal is cited, in accordance with accepted academic practice. No use, distribution or reproduction is permitted which does not comply with these terms.
*Correspondence: Guanghui Zhan, emhhbmdoMjAwN0AxMjYuY29t