Radon on Mt. Etna (Italy): a useful tracer of geodynamic processes and a potential health hazard to populations
- Etna Observatory, National Institute of Geophysics and Volcanology, Catania, Italy
Radon gas and its radioactive daughters have been extensively studied on Mt. Etna, both in local volcanic rocks and in all types of fluid emissions from the volcano (crater gases, fumaroles, mofettes, soil gases, groundwaters). The first measurements date back to 1976 and were carried out both in local volcanic rocks and in the crater plume. Since then, fifty-four scientific articles have been published. The largest majority of them (more than 50%) correlated radon emissions with volcanic activity and/or magma dynamics inside Mt. Etna. Many others were focused on possible correlations between time variations of in-soil radon and tectonic activity. The concentration of radionuclides in Etna volcanic rocks was measured on several occasions in order to set background values of radon parents and to study the dynamics of Etna magmas. Some articles analyzed the concentrations of radon in Etna groundwaters and their temporal changes in relation to volcanic activity. Only a few studies focused on methodological aspects of radon measurements in the laboratory. Finally, in recent years, geoscientists began to analyze the possible negative effects on human health from high concentrations of indoor radon in houses near active faults. The overall results show that, in most cases, it is possible to understand the endogenous mechanisms that cause changes in soil radon release from rocks and its migration to the surface. Several physical models were produced to explain how those changes were correlated with Etna’s volcanic activity, making them potential precursors, especially in the cases of eruptive paroxysms. More complex is the analysis of radon changes in relation to tectonic activity. Indeed, if measurements of radon in soil is now considered a robust methodology for identifying buried faults, radon time variations are not always clearly correlated with seismic activity. This difficulty is likely due to the complex interplay between tectonic stress, magma migration/eruption and gas release through faults. In any case, the potential high hazard for human health due to high concentrations of indoor radon in houses close to faults seems to be a well-established fact, which requires particular attention both from the scientific community and the public health authorities.
1 Introduction
Radon gas has attracted the attention of geoscientists at least in the past 50 years, because of its great potential as tracer of many geodynamic processes such as volcanic eruptions and earthquakes, due to its physical properties (chemical inertness, high density, relatively short half-life, widespread distribution). More recently, it also came under the spotlight because of its severe impact on human health, due to its radioactivity and its capability to diffuse and accumulate in the atmosphere inside buildings.
On Mt. Etna volcano studies on radon and, more generally, on natural radioactive isotopes started in the mid-1970s (Capaldi et al., 1976; Lambert et al., 1976). However, it was only in the last 20 years that radon was extensively and deeply studied in terms of its sources, sinks and mode of transport to the surface. Until today, a remarkable number of data were produced from very different types of samples (rocks, groundwaters, crater gases, fumarole gases, soil gases, air; Figure 1) that have shed more light both on the benefits of using radon gas to understand how this volcano works and on its high potential risk for human health.
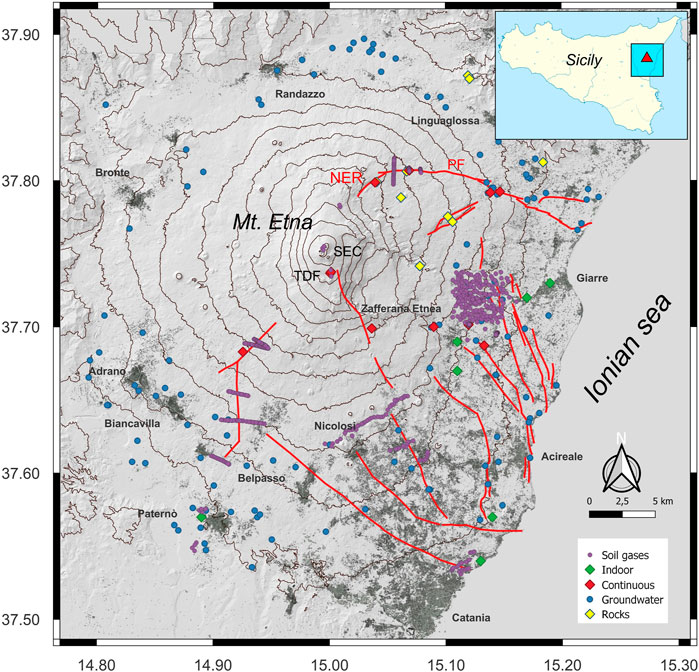
FIGURE 1. Sketch map of the Mt. Etna area, showing the different types of radon measurements carried out so far (see the legend). The red lines show the major tectonic faults on the Volcano. The main towns are also indicated with their names. TDF = Torre del Filosofo; NER = North-East Rift; PF = Pernicana Fault. Elevation contour lines every 250 m.
The World Health Organization (WHO), through the International Agency for Research on Cancer (IARC), has classified radon in Group 1 of carcinogenic substances, which includes substances for which there is sufficient evidence of carcinogenicity on the basis of human epidemiology studies. In particular, exposure to radon indoor increases the risk of contracting lung cancer. Other possible health effects of radon exposure have been studied, such as an increased risk of leukemia, but to date there are no certain conclusions other than the increased risk of lung cancer. The extent of the risk depends both on the concentration of radon to which people are exposed and on how long the exposure lasts. The risk of lung cancer was found to increase by 8.4% per 100 Bq m−3 increase in measured radon (Darby et al., 2005). Given equal conditions of radon exposure, smokers are more at risk (about 25 times more) than non-smokers due to the synergistic effect between radon and cigarette smoke (Darby et al., 2005). In Italy, the Istituto Superiore di Sanità has estimated that about 10% of the 31,000 cases of lung cancer recorded every year is attributable to radon.
This work is intended not only to give the state of the art in the research on radon on Mt. Etna, with categorized lists of all papers published on this subject and a brief description of the main results obtained, but also to provide a comprehensive model of what we have understood about this volcano and its environment based on the radon and radio-isotopes data collected so far and to figure out what possible developments (both scientific and technical) can take place in the near future.
2 Radioactivity in Etna volcanic rocks
Studies on radioactive isotopes in volcanic rocks of Mt. Etna were among the first to be carried out on this volcano (Capaldi et al., 1976), with the aim to imply the dynamics of magma ascent during pre-eruptive periods from the radioactive disequilibria between Ra, Th and U short-lived daughters belonging to the decay chains of 232Th and 238U (all measurements performed with gamma spectrometry). Since the first results, it was found that Ra isotopes were in excess over equilibrium contents, especially regarding the 228Ra/228Th ratio, and this was modeled as a long-term (in the order of tenses to hundreds of years) injection of Ra-rich magma. Conversely, 230Th/238U and 234U/238U ratios were in substantial agreement with equilibrium conditions.
More extensive studies on a larger number of rock samples (Condomines et al., 1995) and a larger number of isotopes (238U, 230Th, 226Ra, 210Pb, 87Sr, and 86Sr) allowed to observe changes related with crystal fractionation over time scales of a couple of centuries, and to estimate both the residence time (max 1,500 years) of magma in the deep reservoir feeding the volcano and to infer a volume of magma in the order of 150–300 km3. Furthermore, it was observed that since 1970 this reservoir was injected with new basaltic magma with isotopic signature suggesting crustal contamination.
A new series of measurements of the same isotopes as those surveyed by Condomines et al. (1995) was performed both in the 2001 and in the 2002–2003 lavas (Clocchiatti et al., 2004). The results fully confirmed those of Condomines et al. (1995) and strengthened the hypothesis that those two eruptions were fed by a volatile-rich magma intrusion independent of the central conduits of the volcano (“eccentric” or “peripheral” intrusion) and composed of new basaltic melt produced from a large-scale metasomatized mantle source.
Some other radioactivity measurements were recently performed in rock samples collected mostly from the SE flank of Mt. Etna (Kozłowska et al., 2016; Kozłowska et al., 2019). Radium isotopes 226Ra and 228Ra were measured by gamma spectroscopy, giving average values of 61.8 and 53.3 Bq/kg, respectively, thus slightly lower than those of the volcanic rocks from other nearby volcanic areas.
The only direct measurements of radon emission from volcanic rocks of Mt. Etna were carried out by Morelli et al. (2011), who performed laboratory measurements of radon exhalation rate from rock samples using the can technique (Grasty, 1997) and CR-39-based (diglycol carbonate) detectors exposed inside each can for 3 months. Both volcanic rocks of different composition and non-volcanic rocks (sedimentary and metamorphic) were analyzed, showing that higher radon exhalation values were found in volcanic rocks, following their higher uranium content, reaching about 700 m Bq m−2 h−1. The results were propaedeutic for developing models of radon transport and release at the surface through soils.
3 222Rn in crater gas emissions and in the air
Determination of radon emission from open conduit degassing at active volcanoes during the last 40 years was mostly performed through the measurement of the last three, long-lived, radon daughters (namely, 210Pb, 210Bi, and 210Po) in volcanic plumes (e.g., Lambert et al., 1976). Studying 210Pb-210Bi-210Po radioactive disequilibria in magmatic gases from open-conduit persistent crater plumes has proved useful to infer degassing dynamics of active volcanoes (Lambert et al., 1976; Gauthier et al., 2000; Le Cloarec & Pennisi, 2001; Allard et al., 2016), where major gases like CO2 and H2O act as carriers for radon and its daughter radionuclides. On Mt. Etna, such measurements were carried out since 1988 using cellulose acetate filters to trap the airborne aerosol particles that carry those radionuclides and then measuring alpha and beta radioactivity in the collected samples (Lambert et al., 1976; Le Cloarec et al., 1988; Marty and Le Cloarec, 1992; Le Cloarec and Pennisi, 2001; Terray et al., 2018).
The basic assumption of all the above studies is that radioactive equilibrium is achieved among all of the 238U daughters (e.g., Lambert et al., 1976), therefore the above long-lived decay products of radon associated with the aerosols collected are reasonably all emitted from magma. One of the first results so far obtained was to infer the degassing time of magma inside Etna’s shallow conduits and to show the different proportions of fresh magma that is involved in convective processes (Le Cloarec et al., 1988). In fact, the measured activities of the above nuclides were actually found to be very different, with a marked enrichment in 210Bi and 210Po relative to 210Pb, because of the higher volatility of the former nuclides with respect to that of the latter. Using the ratios 210Bi/210Pb and 210Po/210Pb both in the volatile phase and in the magma it was possible to model how their radioactive disequilibria in the gas phase vary in time after exsolution, thus allowing for the estimate both of the magma residence time and of the gas transfer time in the volcano conduit, especially if taking 222Rn into account in the degassing models (Terray et al., 2018; Terray et al., 2020a). Other models used the above nuclides together with SO2 crater fluxes to infer the dynamics of magma in the shallow conduits of Mt. Etna, as well as the volume of degassing magma and the proportion of non-erupted degassing magma (Le Cloarec and Pennisi, 2001). Furthermore, using elemental ratios between other magmatic gases (CO2, He) detected in the crater plume emissions and 210Po it was possible to estimate their volcanic fluxes (Marty and Le Cloarec, 1992).
The main results of the above studies were: i) assessment of the role of the South-east Crater as a “secondary” degassing vent (i.e., not directly related with the central conduit of the volcano); ii) assessment of the existence of different magma convective cells within the conduits of the volcano; iii) estimate of the proportion of degassed magma eventually erupted (e.g., 15%–20% during the period 1983–1995); iv) magma residence times within the shallow reservoirs in the order of 500–1,000 days; v) transfer times of magma to the surface shorter than 7 days.
It was only recently that the first direct measurements of in-air radon were carried out, both at the summit craters of Mt. Etna (Terray et al., 2020b) and at different altitudes around the volcano (Vaupotič et al., 2010).
Vaupotič et al. (2010) investigated radon contents in outdoor air both close to the volcano summit and in other areas along its flanks, showing radon concentrations in the range from 3.0 to 19.6 Bq m−3. Higher average values were generally found in the west sector of Mt. Etna, but the highest ones (up to 93 Bq m−3) were measured at high altitude (about 3,000 m asl).
In particular, Terray et al. (2020a) used a network of fixed dosimeters around the rim of the central crater of the volcano to monitor radon emissions for several months. This allowed for a better constraint of radon emission in an open-conduit volcanic plume and also for the assessment of the potential health hazard for the people working or visiting the summit area of Mt. Etna. The results indicated higher radon concentrations downwind and also close to high-flow fumaroles, thus showing substantial emission of this gas both from crater plume degassing and from fumarole emissions around the rim of the central crater of Mt. Etna. In terms of potential radon hazard in air, the radon contents in the high-concentration sites were much higher than the thresholds recommended by the WHO handbook (WHO, 2009) for 8-hour-long indoor exposure (radon values measured in 4 sites out of 41 at 1 m elevation above ground ranged from 704 to 8,827 Bq m−3, compared to a maximum recommended concentration of 300 Bq m−3). However, both the very short periods of exposure (normally << 8 h) and the rapid dispersion of radon in open air due to air/gas motion make this hazard low, if not negligible.
4 222Rn in groundwaters
Despite the growing interest on this subject around the world, relatively few papers (including conference materials) on radon activity in groundwater have been published on Mt. Etna area (D’Alessandro and Vita, 2003; Galli et al., 2000; Quattrocchi et al., 2000; Kozłowska et al., 2009; Kozłowska et al., 2016; Kozłowska et al., 2019; Fiore et al., 2011). This is quite surprising, considering that the groundwaters of Mt. Etna not only represents the main drinking water resource for the local population and are also distributed in the municipal water networks of the surrounding areas, but are also used both for agricultural and for industrial purposes.
Groundwater contamination from radon is a serious global concern: the major health hazard occurs due to toxic indoor air inhalation and consumption of contaminated drinking water supplied from groundwater. It is widely shared the assumption that the radon was found to be directly or indirectly responsible of about 2% of all death from cancer in Europe (Darby et al., 2005) and that indoor radon levels derived from groundwater use in the United States are thought to cause 1%–7% of fatal lung cancers (Cothern et al., 1986). Studies have been conducted in order to find the correlation between the drinking water radon contamination and gastrointestinal malignancies, but the consequences are contradictory (Auvinen et al., 2005). Epidemiological studies on the incidence of cancer in the volcanic area of Etna, cannot draw any conclusion on the association between increased exposure to 222Rn and the risk of thyroid cancer (Pellegriti et al., 2009) The guidance levels for waters intended for human consumption in 24 European Union countries are 100 Bq L−1 (Jobbàgi et al., 2017).
The only extensive survey on Mt. Etna’s groundwater for the specific determination of dissolved radon contents was performed by D’Alessandro and Vita (2003), who measured 222Rn activity in 119 groundwater samples collected throughout the active volcanic area of Mt. Etna. The activity values, measured by means of a portable Lucas-type scintillation chamber, ranged from 1.8 to 52.7 Bq L−1, highlighting that about 40% of the samples exceeded the maximum level of 11 Bq L−1 proposed by the USEPA in 1991. Samples collected from wells showed generally higher radon contents than those collected from springs and drainage galleries, probably because of radon loss at the contact with the atmosphere in the well head-space. Despite the rather uniform composition of the Etnean volcanic rocks, with a very narrow range of uranium content, the 222Rn activity in groundwaters showed an uneven distribution. The highest radon levels were found in the eastern sector of Mt. Etna, the tectonically most active area of the volcano (with the maximum values clustering along the Timpe Fault System). The south-western sector, on the contrary, despite its intense geodynamic activity (high seismicity and strong magmatic degassing at the surface from both focused vents and diffuse degassing areas), displayed lower radon levels. This is probably due to the formation of a free gas phase in the groundwater (due to oversaturation of water in magmatic CO2, which is particularly abundant in this part of the volcano) that strips the radon from the water. The gas emitted from water into the soil, therefore, would be strongly enriched in radon compared to groundwater. Moreover, comparing the data collected at Mt. Etna with those of other volcanic areas of Italy, D’Alessandro and Vita (2003) show that the two main factors controlling radon release to the aquifers are i) the content of parent elements in the aquifer rocks and ii) the temperature of the geothermal systems that interact with the sampled aquifers. The former acts when the leaching of U and other parent nuclides in the host rocks is more effective due to Eh-pH conditions in the groundwaters that favor mineral dissolution (Aiuppa et al., 2000), whereas the latter is explained by the inverse correlation between radon solubility in water and water temperature, so that higher temperature causes greater evolution of radon from water (Wilhelm et al., 1977).
Both Galli et al. (2000) and Quattrocchi et al. (2000) described the design and set-up of automated monitoring stations for the determination of many physical and chemical parameters, including dissolved radon, in Etna’s groundwater. The few radon concentration data indicate background values in the same range as that shown by D’Alessandro and Vita (2003).
Kozłowska et al. (2009) investigated radioactivity in underground waters from Mt. Etna on the basis of 13 water samples. The samples were collected from springs, wells and galleries around the volcano. Water from nine out of thirteen intakes is actually used for human consumption. Activity concentration measurements of 222Rn were performed with a liquid scintillation counter. Radon activity concentration was found within the range from 1 to 13 Bq L−1, hence these waters can be classified as low-radon waters.
Kozłowska et al. (2016) focused on activity concentration of 222Rn measured in 9 water samples from the aquifers of the eastern flank of Mt. Etna volcano. Three samples were from water drainage galleries and six from water wells, all used for human consumption. Sampling was performed three times, in 2011, 2012, and 2013. This area is a good case study because it is characterized by large volumes of groundwater and by strong interactions between groundwater, volcanic rocks, volcanic gases and some hydrothermal fluids. Moreover, it is also the area of Mt. Etna with the highest values of dissolved radon, as already shown by D’Alessandro and Vita (2003). Measurements of radon activity concentration in water were performed with a liquid scintillation technique. All water samples showed Radon activity concentration within the range from 2.91 ± 0.36 to 21.21 ± 1.10 Bq L−1, hence these waters can be classified as low-radon waters.
Finally, on the basis of a radiometric survey carried out on eight rock samples from the eastern flank of Mt. Etna, representative of the average rock type of the aquifers, Kozłowska et al. (2019) made an interesting attempt to determine radionuclide transfer factors from soil to water and to calculate the radiological risk resulting from ingestion of these isotopes contained in drinking water. Based on both the results of this study and those of previous investigations (Kozłowska et al., 2016), the Authors concluded that the magmatic rocks of Mt. Etna show favorable conditions for uranium leaching by water, whereas thorium and radium remain in the host rocks of the aquifer. Furthermore, the effective radiation doses due to radionuclides ingestion via drinking water are significantly below the limit of 100 μSv/year set by WHO (2017) and the limit of 1 mSv/year set by the Italian law, and therefore represents a rather limited risk to human health.
5 222Rn and 220Rn in-soil gas
5.1 General outlines
Measurements of radon (both 222Rn and 220Rn) in soil (Figure 2) can produce different results as a function of many factors, the most important of which are: i) the concentration of parent radionuclides in the different layers of rock in the sub-soil; ii) the grain size (i.e., the porosity) of the surface layers of the soil; iii) the presence of a pre-fractured soil, which increases the permeability of subsurface rocks; iv) the concurrent presence of other soil gases (mostly CO2) in the sub-soil that can act as carrier for radon and thus enhance (or dampen by dilution, in case of excessive gas flow rates) radon emissions; v) the proximity of an active fault plane, which through its fractures increases the local permeability of rocks; vi) the influence of environmental parameters like humidity, wind speed and temperature, the latter both in the air and in the soil atmosphere.
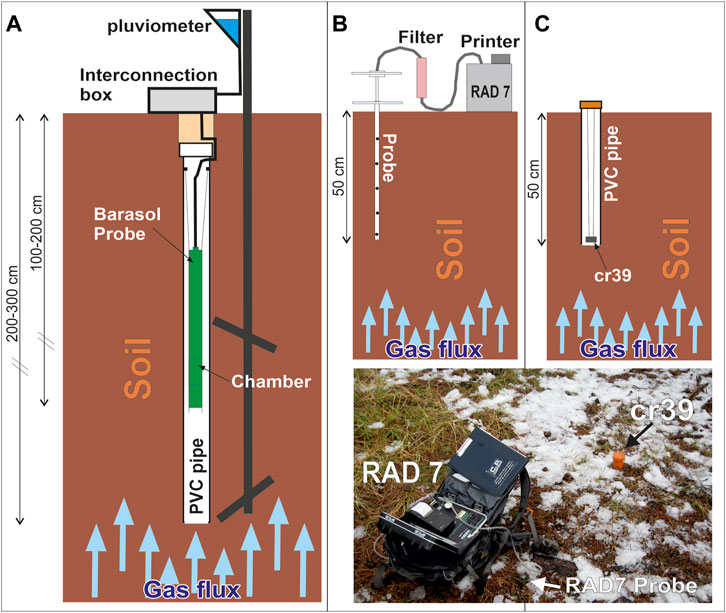
FIGURE 2. Summary of the different methods used to measure in-soil radon on Mt. Etna. Inbox (A) shows the typical setup of the sensors for continuous radon monitoring; (B) shows the field setup for discrete measurement both of 222Rn and 220Rn with Durridge RAD7 portable radonmeter; (C) shows the setup for long-term monitoring of radon using track detectors (SSNTD CR-39 type).
In-soil radon detection can be either discrete, being performed discontinuously in time in the same location or as spot measurements in different locations over large surfaces, or near-continuous, being performed automatically in the same place at time intervals ranging from a few minutes to a few hours.
On Etna, temporal changes of in-soil 222Rn activity were observed simultaneously with changes in volcanic activity (Le Cloarec et al., 1988; Alparone et al., 2005; Neri et al., 2006, 2016; Giammanco et al., 2007, Immè et al., 2005; Immè et al., 2006a; Immè et al., 2006b; Immè et al., 2014; Morelli et al., 2006; La Delfa et al., 2007; Immè and Morelli, 2012; Falsaperla et al., 2014; Falsaperla et al., 2017) and/or during increased tectonic activity in the proximity of fault planes (Burton et al., 2004; Brogna et al., 2007; La Delfa et al., 2007, 2008; Neri et al., 2007, 2011, 2014, 2016, 2019; Giammanco et al., 2009; La Delfa et al., 2010; Siniscalchi et al., 2010; Morelli et al., 2011a; Morellia et al., 2011b; Vizzini and Brai, 2012; Bonforte et al., 2013; Johnova et al., 2014; Woith, 2015). Moreover, some studies were aimed at verifying the correspondence between the strongest in-soil radon emissions and the indoor radon pollution in buildings located in areas with surface faulting, in order to evaluate the possible risks to human health (Brogna et al., 2007; Pellegriti et al., 2009; Neri et al., 2019).
5.2 Continuous radon measurements
The first continuous measurements of in-soil 222Rn activity on Etna were performed from January 1992 to January 1993 using a probe equipped with a solid-state detector (Alphametertm—Alphanuclear Co., Canada), placed at the bottom of a 2 m-deep bore-hole at altitude of about 1,650 m asl, close to a dry fissure that formed during the 1989 flank eruption and that re-activated during the 1991–1993 flank eruption (Badalamenti et al., 1994). Anomalous variations were observed mostly in late January 1992, seemingly correlated with a magmatic intrusion along the 1989 dry fissure. Further radon monitoring was performed some months later using a similar sensor (Barasol Multisensor BMC2, Algade, France) by Pinault and Baubron (1996) and some years later also by Alparone et al. (2005). In both cases, the 222Rn station was located ∼1 km South of SEC, at 2,940 m altitude in a place called “Torre del Filosofo” (see Figures 1, 3). In the former, some large anomalous spikes were observed and they were interpreted as non-periodic, spasmodic 222Rn emissions likely due to volcanic activity. In the latter, the Authors analyzed 222Rn measurements carried out during a period of frequent eruptive episodes that occurred at South-East Crater (SEC) in September-November 1998, using the same type of solid-state sensor device as that used by Badalamenti et al. (1994), placed at the bottom of a 1 m-deep sealed bore-hole. Air temperature and air relative humidity were also measured over 1 h periods by sensors placed in the same location. According to Alparone et al. (2005), conspicuous increases of 222Rn were recorded at least 46 h before each of five episodes of lava fountaining that occurred at SEC during the period of monitoring, thus demonstrating the potential use of 222Rn as a precursor to strong explosive basaltic eruptions (Figure 3C).
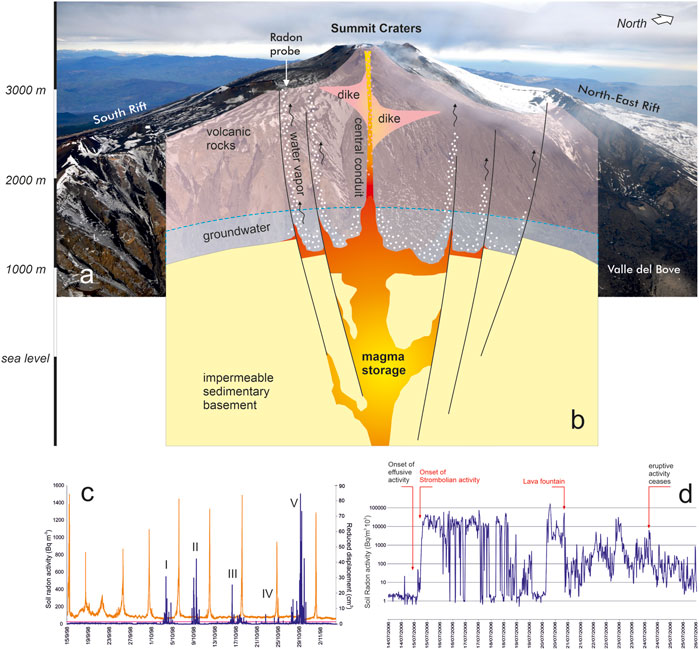
FIGURE 3. (A) Location of the continuous 222Rn monitoring stations used from 1996 to 2013 in the site called “Torre del Filosofo” (TDF in Figure 1, at 2,940 m asl) along the so-called South Rift of Mt. Etna; (B) Schematic volcanologic and structural N-S profile of the summit of Mt. Etna, showing a model of radon degassing from magma and across the groundwater/hydrothermal system towards the radon monitoring station; (C) results of in-soil radon monitoring at TDF during 1998, where the blu lines is the radon signal, the orange line is the reduced displacement associated with volcanic tremor amplitude and roman numbers indicate the radon anomalies associated with lava fountain episodes (modified from Alparone et al., 2005); (D) radon signal at TDF during July 2006, showing the main changes associated with eruptive activity. Radon values were obtained using a BMC2 barasol probe (limit of detection = 50 Bq m−3; range from 0 to 1 GBq m−3) (modified from Neri et al., 2006).
Considering these first positive experiments, a Barasol Multisensor BMC2 (Algade, France) probe was later installed in the same site in July 2005. Since then, the 222Rn probe was constantly active until 2013, when a lava flow buried the monitoring station. During the period of operation, the probe collected data useful for monitoring the volcanic activity at the summit craters of Etna, particularly during the 10-day-long July 2006 Strombolian/effusive eruption (Neri et al., 2006). Both the onset of explosive activity and a lava fountain episode at SEC were preceded by some hours by increases in 222Rn in-soil emissions. Anomalous peaks in 222Rn activity were interpreted as due to micro fracturing of uranium-bearing volcanic rocks. These observations confirmed the hypothesis that in-soil 222Rn measurements collected in the Etna’s summit area can be strongly controlled by the state of volcano-tectonic stress within the volcano and demonstrate the usefulness of high-frequency 222Rn acquisition before and during explosive-effusive eruptions (Figure 3D).
In addition to this, between February and April 2007 the 222Rn probe installed at Torre del Filosofo detected some episodes of volcanic activity that were not evident at the surface, i.e., magma ascent along the central conduit, and did not culminate in an eruption. Falsaperla et al. (2014) described several of these cases, called “failed eruptions”, during inter-eruptive periods between paroxysmal eruptions at the SEC. Failed eruptions were characterized by increases in volcanic tremor amplitude and simultaneous 222Rn anomalies and were explained as ascending magma batches that triggered repeated episodes of gas pulses and rock fracturing, but that were not able to eventually erupt at the surface.
Also in case of long-lasting flank eruptions, the 222Rn probe installed at site Torre del Filosofo demonstrated its usefulness. Falsaperla et al. (2017) analyzed 222Rn data together with other seismic and environmental parameters; the triggering mechanism of 222Rn anomalies was postulated to be either tectonic (promoting rock fracturing) or volcanic (promoting gas pulses) (Figure 4). During the 2008–2009 flank eruption, in particular, numerous episodes of rock fracturing, inferred from seismic swarms, both anticipated and accompanied the beginning of the eruption. Concurrently, vigorous gas pulses causing anomalous peaks in 222Rn emissions testified the arrival of new magma batches at the surface during the same eruption, each one interpreted as a distinct episode of magma re-feeding following deep rock fracturing. Moreover, analyzing the temporal pattern of the anomalous 222Rn emissions, Falsaperla et al. (2017) inferred that the advective ascent speed of the 222Rn gas carrier (mainly water vapor) in the area of Torre del Filosofo was >>94 m/day, hence much faster than diffusive motion of the gas. In this case, the ascent speed was about double that calculated by Neri et al. (2016) in a radon monitoring site located about 7 km away from the summit crater area.
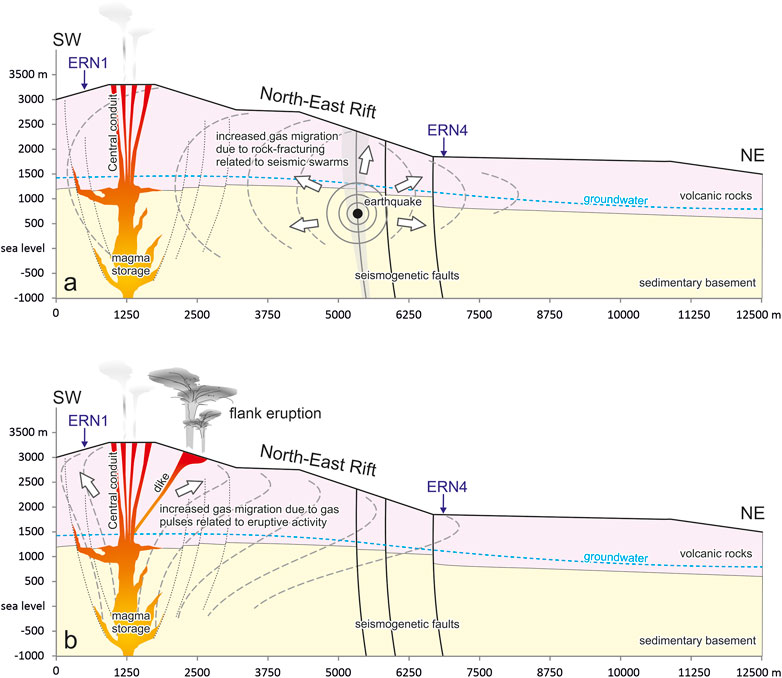
FIGURE 4. Schematic geological sections of the summit area and the NE Rift of Mt. Etna, showing a dynamic conceptual model of radon gas release and migration in case of (A) tectonic strain release due to earthquakes or (B) increase in soil gas velocity due to increased magmatic degassing during dike-fed intrusions (white arrows and dashed grey lines). Dashed red arrows indicate possible directions of magmatic intrusions into the NE rift zone. Modified from Falsaperla et al. (2017).
Other continuous 222Rn measurement stations, equipped with Alphametertm, Barasol or AlphaGUARD (Bertin Technologies SAS, France) monitoring devices, were installed far from the summit craters of Mt. Etna and near active fault planes of the northern and eastern flanks of Etna, that are the areas of the volcano mostly affected by seismic capable faults, at altitudes ranging from ∼450 m to 1800 m asl (Figure 1), in order to monitor mainly the tectonic activity of the volcano (Immè et al., 2005; Immè et al., 2006a; Immè et al., 2006b, 2014; La Delfa et al., 2008; Morelli et al., 2011a, 2011b; Neri et al., 2016; İçhedef et al., 2020). Interesting results were obtained, in particular, by Neri et al. (2016) analyzing the 222Rn data acquired in 2009–2011 by a probe placed in the Piano Provenzana area, on the north-eastern flank, at 1800 m asl (Figure 1). According to the Authors, radon is released mainly from sources at depth <1,400 m, with an ascent speed of >50 m/day. Three periods of anomalous gas release were detected after filtering of the raw radon signal and they were interpreted as having either a tectonic (as in February 2010) or a volcanic (as in January and February 2011) trigger (Figure 5A). This was not a surprise, because the probe was purposely installed in a site that was both near the Pernicana fault and near the North-East Rift of the volcano, that is a volcanic-tectonic structure which intercepts the central volcanic conduit of the volcano (Figure 5B).
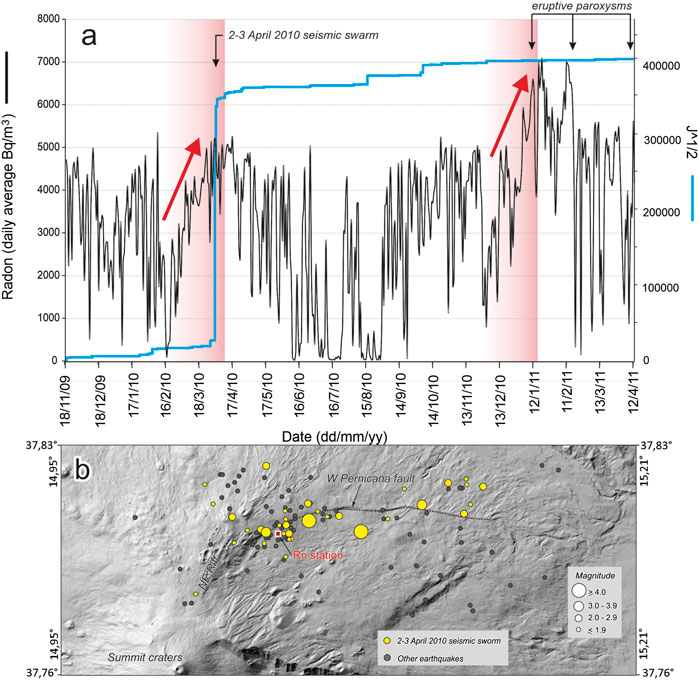
FIGURE 5. (A) Example of in-soil radon signal from the continuous monitoring station located near the Pernicana Fault from November 2009 to April 2011, showing daily average values of radon activity (black line), cumulate strain release during earthquakes (blue line) and summit eruptions of Mt. Etna during the studied period (arrows). 222Rn values were obtained using a BCM2 Barasol probe (Algade, FR). The BCM2 probe integrates and stores the data collected every 20 min (limit of detection = 50 Bq m−3; range from 0 to 1 GBq m−3). Meteorological parameters, such as temperature (0.1°C) and atmospheric pressure (1 hPa), were simultaneously recorded. The two vertical pink bands highlight periods of steady increase in radon emissions (red arrows: each period lasted about 7 weeks) that preceded both major seismic events and summit eruptions; (B) earthquake epicenters distribution from 2 November 2009 to 13 April 2011, projected onto a Digital Elevation Model of the NE flank of Mt. Etna. Modified from Neri et al. (2016).
More recent studies (İçhedef et al., 2020) have focused on how to filter out all the periodic cycles from the radon signals recorded at different stations around the volcano using digital band-stop filters after applying the Fast Fourier Transform algorithm, assuming these were caused by non-volcanic influences (environmental parameters, Earth tides, etc.). This kind of approach is intended to avoid recording environmental parameters that are then used to filter the raw radon time series based on linear regression models. The alternative filtering procedure of İçhedef et al., 2020 helped reveal aperiodic radon changes that were interpreted and modeled in terms of gas-pressure waves that propagated away from the central conduits of the volcano during rapid pre-eruptive magma ascent episodes.
5.3 Discrete radon measurements
It is known that the measurement of in-soil radon (222Rn) and thoron (220Rn) emissions are greater in areas characterized by high permeability of the rocks richer in uranium and in the presence of significant fluxes of carrier gases (mostly water vapor and CO2). These conditions often occur in areas with surface soil faulting, i.e., in the immediate proximity (from tens to hundreds of meters) to active faults. On Etna, these conditions are mainly found along the eastern and southern slopes of the volcano, where numerous and important active and capable fault systems occur (Branca et al., 2011; Barreca et al., 2013, and references therein) and dry fissures have often opened during important flank eruptions. However, these volcano-tectonic structures, or parts of them, are often buried by recent and historical lava flows, due to the high frequency of Etna eruptions and the large volumes of volcanic materials (especially tephra) emitted. In these cases, one can only guess the presence of such faults because of linear morphological flexures of the topographic surface. However, in recent decades a valid support to the identification of buried active faults has been provided by discrete surveys of in-soil radon performed with portable instruments. This approach was first tested in the field with measurements across already known tectonic systems (Chiodini et al., 1989; Badalamenti et al., 1994; Burton et al., 2004; Brogna et al., 2007; Giammanco et al., 2009; Siniscalchi et al., 2010), which provided objective evidence about the correspondence between radon anomalies and faults. After obtaining positive results with this method, in-soil radon surveys were carried out in areas apparently without evidence of tectonic dislocations on the surface, but marked by the presence of linear morphological flexures and/or characterized by numerous earthquake epicenters aligned along well-defined spatial directions, which suggested the presence of buried seismogenic faults (Neri et al., 2007, 2011, 2014; Morelli et al., 2011b; Bonforte et al., 2013; Johnová et al., 2014).
Two different types of in-soil radon measurements were used for discrete surveys: passive and spot (Figure 2). Passive measurements (only for 222Rn) were performed using track detectors (SSNTD CR-39 type), while spot measurements (both for 222Rn and 220Rn) were made using an active portable device (model RAD7, Durridge COMPANY Inc., Bedford, United States). Moreover, in some cases (i.e., Giammanco et al., 2009; Bonforte et al., 2013) soil CO2 effluxes were measured at the same sampling points of radon using the accumulation chamber method (Farrar et al., 1995; Chiodini et al., 1998). An example of an in-soil radon measurement profile is shown in Figure 6, that shows both 222Rn and 220Rn measurements performed along two profiles ∼1,000–2000 m long: radon anomalies were found in correspondence of fault planes and they were always associated both with other soil gas anomalies (mostly CO2) and with ground deformation observed from satellite (In-SAR) data. Also, the different 220Rn/222Rn ratios in soil gases would highlight different stress regimes in the surveyed faults driving the gas to the surface. Actually, faults characterized mostly if not exclusively by creep motion, thus producing constant fracturing in the shallow rocks, are associated with higher 220Rn emissions compared to 222Rn, due to the shorter half-life and hence shallower production source of the former (Burton et al., 2004).
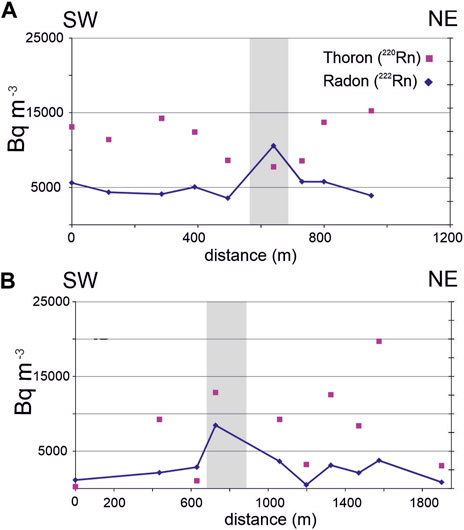
FIGURE 6. Example of profiles of in-soil 222Rn and 220Rn activities measured across faults (grey bands) on the southern flank of Mt. Etna: (A) seismogenic fault with emission of “deep” 222Rn only; (B) shallower fault with creep motion and marked emission of “shallow” 220Rn. The Durridge RAD7 instrument was used to measure radon and thoron concentrations in soils (Measurement = +/−5% absolute accuracy). According to the method suggested by Burton et al. (2004), one 222Rn and 220Rn measurement consists of hammering a hollow metal tube into the soil to a depth of 50 cm, inserting a plastic tube connected to the inlet of the RAD7 instrument and performing three measurements of radon and thoron activity each with 5-min integration time. The result was determined by taking the average of the second and third integrations. When the difference between these two was greater than 30% we repeated the measurement until the difference was reduced. Modified from Bonforte et al. (2013).
Therefore, the radon surveys conducted on Etna showed that in-soil measurements can be a valid method to identify buried active and capable faults and to evaluate the state of stress of the tectonic structures under investigation (Burton et al., 2004; Neri et al., 2007; Neri et al., 2011; Neri et al., 2014; Bonforte et al., 2013; Johnová et al., 2014). Moreover, according to Burton et al. (2004), a high spatial resolution of radon sampling (sampling step of the order of some tenses of meters) is required to correctly map radon anomalies. The ability to identify and localize active faults using radon surveys at high spatial resolution has a strong impact on the assessment of the local risk posed by volcano-tectonic earthquakes (Brogna et al., 2007; La Delfa et al., 2008; Pellegriti et al., 2009; Neri et al., 2016).
Monitoring 220Rn/222Rn ratios in time along faults, if associated with concurrent soil CO2 effluxes in the same sites, was found to be a proxy for the velocity of gas in the subsoil, which in turn is a function of the gas pressure in the volcanic system (Giammanco et al., 2007). Starting from the above assumptions, the temporal changes of a new parameter, named Soil Gas Disequilibrium Index, that incorporates the combination between CO2 efflux and 220Rn/222Rn ratio, proved effective in detecting changes in the degassing regime inside Mt. Etna before eruptions (Giammanco and Sims, 2022).
In many cases, analysis of radionuclides concentration in rock samples was also undertaken in the radon survey areas (Seidel and Monnin, 1984; Condomines et al., 1995; Giammanco et al., 2009; Morelli et al., 2011a,b; Johnová et al., 2014; Catalano et al., 2015a,b; Neri et al., 2016) and in one case it was carried out in the same measuring points as the in-soil radon content, showing similar patterns of all parameters along the surveyed profiles (Johnová et al., 2014).
5.4 222Rn indoor
Since late 2007, continuous long-term (up to ∼3 years) indoor radon monitoring was performed inside several buildings located in the Etna area at various altitudes (Figure 1; Brogna et al., 2007; Neri et al., 2019), in order to evaluate the potential risk from radon pollution for the inhabitants, since radon is among the main causes of cancer of the respiratory system (Baxter, 1990; Muirhead, 1994; Baxter et al., 1999; Pellegriti et al., 2009; Rodríguez-Martínez et al., 2018). Recent studies (Darby et al., 2005; Health Protection Agency, 2009; WHO, 2009) suggest radon thresholds above which the probability of lung cancer incidence increases significantly. These thresholds correspond to 100 Bq m−3 (attention threshold) and to 300 Bq m−3 (maximum threshold recommended), both considered at an exposure time of 8 h. The starting hypothesis was that buildings placed near or on active faults are more exposed to indoor radon pollution, given the higher radon emission from faults as evidenced from in-soil radon surveys. Radon can spread from the fractured ground and diffuse into buildings mainly through their foundations, where they are in direct contact with the ground, being trapped inside the houses due to its high density.
A first study of indoor radon concentration was performed by Brogna et al. (2007), using an ionization chamber and carbon cylinders, making spot measurements in 150 sites of inhabited areas of the south-eastern slope of Mt. Etna. Higher 222Rn concentrations were generally found in basements and ground floors, but the highest values were measured especially in houses built near or on faults, demonstrating that faulted areas are preferential pathways for radon migration through the ground and its emission at the surface, which in turn can cause indoor pollution.
La Delfa et al. (2012) monitored indoor radon in the east flank of Mt. Etna, near an active tectonic fault, and they found correlations between radon accumulation into buildings, type of construction materials and also Etna volcanic activity.
Neri et al. (2019) used a digital radon-monitor to measure alpha emissions with an Rn-accumulative method and their results confirmed those by Brogna et al. (2007). In particular, it was demonstrated that: i) the problem of indoor radon pollution in Etna buildings exists (indoor radon concentrations up to 3,549 Bq m−³ were found); ii) the most polluted buildings were those located near (from tens of meters to a few hundred meters) active and capable faults; iii) the distance of monitoring sites from faults was a key parameter in determining the accumulation of radon indoor (Figure 7), and iv) the construction features of the houses seemed to play an important role in the mitigation of indoor radon accumulation, even in the presence of intensely degassing soils.
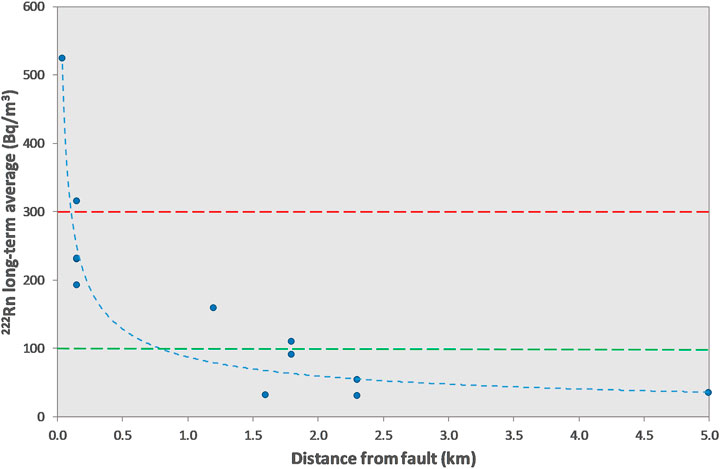
FIGURE 7. Spatial relationship between average radon indoor concentrations in houses of the southern and eastern flanks of Mt. Etna and distance from faults. The best fit model to the data (dashed blue line) follows a negative power law (y = 3.928 x−0.551; R2 = 0.8). The dashed red line indicates the threshold value (300 Bq m−3) recommended by WHO (2009), whereas the dashed green line indicates the attention threshold (100 Bq m−3) suggested by WHO (2009). Modified from Neri et al. (2019).
The data acquired both by Brogna et al. (2007), La Delfa et al. (2012) and Neri et al. (2019) clearly show that it is necessary to deepen this type of study, extending the measurements of indoor radon to other, larger urban areas of Etna volcano, in order to evaluate in detail and monitor the health risk over the time for the population living on the volcano (about one million people).
6 Discussion and conclusive remarks
From the studies carried out so far on Mt. Etna, several important considerations can be outlined, which we have used to produce a comprehensive model that takes into account both the mechanisms of radon release from magma and its surrounding rocks and those of its transport to and accumulation at the surface (Figure 8).
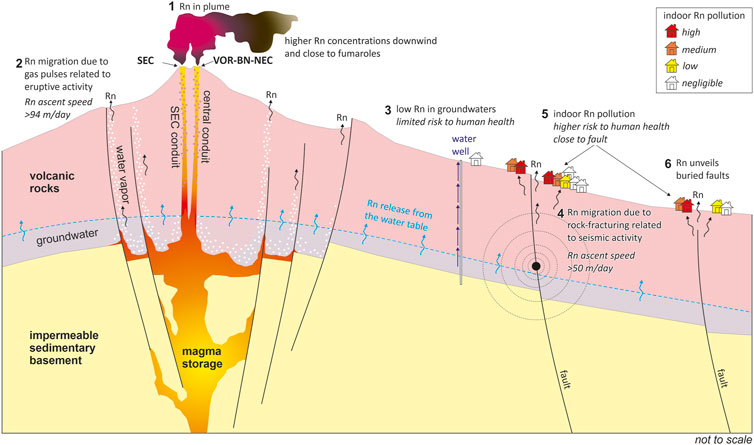
FIGURE 8. Comprehensive model of radon sources, mechanisms of transport and discharge at the surface and potential accumulation indoor at Mt. Etna.
First of all, radon parents in volcanic rocks are useful to reveal processes and evolutionary trends in the magmatic source underneath Mt. Etna; they also define the background radioactive levels that serve as the basis for the models of radon gas emission at the surface. In these senses, periodic analysis of radon and radionuclides contents in Etna lavas will certainly help monitor possible long-term changes in the physical and chemical processes affecting the deep source of magma that feeds the volcano and/or to better understand shallower degassing processes the may occur within the conduits of Etna just before eruptions.
The radon emitted into the plume by the summit craters of Mt. Etna comes mainly from the outgassing of the magma residing both in the shallow magma chamber and in the overlying conduits that reach the topographic surface (“1” in Figure 8). High Rn concentrations in the air are found along the crater rims, especially near fumaroles and in downwind conditions. This produces a potential, albeit limited, risk to the health of people who constantly visit those areas and stand there for a long time (up to several hours), such as scientists, technicians working for the maintenance of volcanological equipment, volcanological and alpine guides who accompany tourists. Radon and its parents and daughters in summit crater gases help define the possible direct link of a specific crater conduit to the deep source of magma beneath the volcano and they allow for the determination of transfer times of gas from the magma reservoir to the surface, thus supporting volcano monitoring and surveillance techniques. To date, there is no continuous and prolonged measurement over time (many months, years) of radon concentration in the crater plume. It would, therefore, be useful to extend such measurements to long periods of time, so as to include more significant eruptive events than simple passive degassing. This requires equipping the measurement stations with real-time transmission systems, in order to give greater robustness to what has been observed and inferred up to now.
Magmatic outgassing in the summit area of Mt. Etna outside its open craters (>2,700 m a. s. l.) occurs mostly in diffuse form through the rocks where they are permeable to gas by porosity and/or by cracking. Radon laden magmatic gas thus reaches the surface mainly through structural discontinuities that intercept the magma ascent conduits. Such discontinuities on Mt. Etna are represented by eruptive fissures, faults, craters and caldera rims and they may also show intense fumarolic activity (“2” in Figure 8). Steam fumaroles could also occur due to the presence of a boiling shallow aquifer contained in the pile of volcanic rocks overlying the impermeable sedimentary basement. Groundwater is heated and partly vaporized near the magmatic conduits, triggering the rapid ascent of water vapor and other gases, mainly CO2, that carry radon. By combining the 220Rn/222Rn ratio with the soil CO2 efflux it is also possible to get information on the velocity/pressure of the gas system inside the volcano. The temporal monitoring of these parameters proved useful in revealing anomalies correlated with volcanic activity. However, although both radon and environmental data have been acquired for very long periods (several years, since 2005), until today the installed stations have not been capable of transmitting the data, a fact which limits the ability to fully exploit the potential of the acquired signals for monitoring and surveillance purposes.
In-soil radon monitoring stations located in the summit area can record the variations of the volcano’s magmatic activity, and in particular those preceding explosive eruptions, more easily than elsewhere, as numerous studies have shown (Alparone et al., 2005; Neri et al., 2006; Neri et al., 2016; Giammanco et al., 2007; Falsaperla et al., 2014; Falsaperla et al., 2017). However, it has also been noted that in some cases significant increases in in-soil radon emissions occurred in the absence of visible volcanic activity on the surface, leading the phenomenon to variations in the level of magma in the central conduit of the volcano (Falsaperla et al., 2014), or to an interaction between seismic activity and movement of volcanic gases in the summit area (Falsaperla et al., 2017). In any case, it seems certain that a more rigorous understanding of radon variations can only be obtained through comparison of radon data with other types of signals acquired by seismic, deformation and gas monitoring networks. As stated above, an evident limit of the measurements carried out so far on Mt. Etna consists in the absence of automatic transmission systems of the data acquired by the radon stations installed. This allows only for an “a posteriori” data analysis, thus nullifying the potential advantages deriving from following the signal variation trends and therefore the phenomena before and during their occurrence. Furthermore, for future monitoring, it will be essential to associate both radon and environmental sensors (temperature and atmospheric pressure) with the seismic and deformation stations installed in the summit area of the volcano, providing them with data transmission in order to allow for a real-time comparative analysis of all the signals acquired by the monitoring networks.
Radon in groundwaters is generally low, in contrast with the high uranium content in the volcanic host rocks, probably because of water thermalism that allows for a larger release of this gas from the water table, due to the inverse correlation between radon solubility and water temperature (“3” in Figure 8). This would also explain the high radon emissions in the subsurface soil and at the surface, because this radon would be produced both directly from the shallow rocks and also from the groundwater degassing.
Near-continuous monitoring of in-soil radon gas in stations located in the proximity (from tens to a few hundred meters) of active faults can anticipate the tectonic activity of those faults from a few weeks to a few hours, albeit with some limitations and uncertainties in the interpretation of the data (“4” in Figure 8). Indeed, in many cases the radon variations were recorded in the absence of subsequent or simultaneous seismic activity, demonstrating the current unreliability of the data in terms of seismic precursor. This unreliability decreases, however, when radon is analyzed in multi-parameter monitoring stations, i.e., when the radon signal can be directly compared with other geochemical and physical signals, which allows for a better understanding of the monitored phenomena. Therefore, also in this case the hope is to transform at least a part of the current seismic/geophysical stations installed on Mt. Etna into multi-parameter stations (in order to acquire also geochemical and environmental signals) capable of transmitting all signals in real-time.
In-soil radon surveys are extremely useful to find hidden or buried capable faults. In particular, the combined measurement both of 220Rn and of 222Rn allows to distinguish between deep-rooted faults, whose strain release works periodically with earthquakes, and shallow faults that are characterized by a more constant and weaker strain release through creeping (”5, 6” in Figure 8). The systematic application of in-soil radon measurements could, therefore, prove very useful when drawing up highly detailed geological maps, especially in places where recent lava flows hide surface evidence of tectonic structures, such as morphological steps or ground fractures.
Finally, indoor radon monitoring in the Mt. Etna area revealed several cases of houses with strong radon accumulation, well above the safety limits set according to the EU recommendations and regulations. Higher indoor radon levels were found generally in buildings located closer to faults and, for a specific building, during the winter period (”5, 6” in Figure 8). This means that, in future perspective, it would be useful to carry out preliminary in-soil radon degassing measurements both during general territorial planning studies and at a more detailed scale of each individual house or other building intended for human use (such as hospitals, schools, offices, etc.), in order to mitigate the risk of indoor radon pollution. The reduction of the health risk can be obtained through the implementation of adequate construction techniques, such as forced ventilation of the rooms, crawl spaces for ventilation of the foundations, laying of radon-proof coatings and sealing of cracks. The latter in particular is extremely necessary in the case of buildings located in areas with a high release of radon from the soil. Application and implementation of radon dispersion models both indoor and in the atmosphere, which is still to be done on Etna, will help assess the health hazard posed by potential accumulation of this gas into buildings and at the summit areas of the volcano (Josse et al., 2004; Zhang et al., 2021; Mancini et al., 2022).
Author contributions
SG and MN conceived the work and wrote the paper. PB described data on radon in groundwater, collected the literature cited and realized Figure 1. MN elaborated all the other Figures and the conceptual models. All authors listed have made a substantial, direct, and intellectual contribution to the work and approved it for publication.
Acknowledgments
The authors wish to thank SM and FD for their useful comments and suggestions.
Conflict of interest
The authors declare that the research was conducted in the absence of any commercial or financial relationships that could be construed as a potential conflict of interest.
Publisher’s note
All claims expressed in this article are solely those of the authors and do not necessarily represent those of their affiliated organizations, or those of the publisher, the editors and the reviewers. Any product that may be evaluated in this article, or claim that may be made by its manufacturer, is not guaranteed or endorsed by the publisher.
References
Aiuppa, A., Allard, P., D'Alessandro, W., Michel, A., Parello, F., Treuil, M., et al. (2000). Mobility and fluxes of major, minor and trace metals during basalt weathering and groundwater transport at Mt. Etna volcano (Sicily). Geochim. Cosmochim. Acta 64, 1827–1841. doi:10.1016/S0016-7037(00)00345-8
Allard, P., Aiuppa, A., Bani, P., Métrich, N., Bertagnini, A., Gauthier, P. .-J., et al. (2016). Prodigious emission rates and magma degassing budget of major, trace and radioactive volatile species from Ambrym basaltic volcano, Vanuatu island Arc. J. Volcanol. Geotherm. Res. 322, 119–143. doi:10.1016/j.jvolgeores.2015.10.004
Alparone, S., Behncke, B., Giammanco, S., Neri, M., and Privitera, E. (2005). Paroxysmal summit activity at Mt. Etna (Italy) monitored through continuous soil radon measurements. Geophys. Res. Lett. 32, L16307. doi:10.1029/2005GL023352
Auvinen, A., Salonen, L., Pekkanen, J., Pukkala, E., Ilus, T., and Kurttio, P. (2005). Radon and other natural radionuclides in drinking water and risk of stomach cancer: A case-cohort study in Finland. Int. J. Cancer 114, 109–113. doi:10.1002/ijc.20680
Badalamenti, B., Capasso, G., Carapezza, M. L., D’Alessandro, W., Di Ganci, F., Diliberto, I. S., et al. (1994). Soil gases investigations during the 1991–92 Etna eruption. Acta Vulcanol. 4, 135–141.
Barreca, G., Bonforte, A., and Neri, M. (2013). A pilot gis database of active faults of Mt. Etna (sicily): A tool for integrated hazard evaluation. J. Volcanol. Geotherm. Res. 251, 170–186. doi:10.1016/j.jvolgeores.2012.08.013
Baxter, P. J., Baudron, J. C., and Coutinho, R. (1999). Health hazards and disaster potential of ground gas emissions at Furnas volcano, Sao Miguel, Azores. J. Volcanol. Geotherm. Res. 92, 95–106. doi:10.1016/S0377-0273(99)00070-0
Baxter, P. J. (1990). Medical effects of volcanic eruptions: 1. Main causes of death and injury. Bull. Volcanol. 52, 532–544. doi:10.1007/BF00301534
Bonforte, A., Federico, C., Giammanco, S., Guglielmino, F., Liuzzo, M., and Neri, M. (2013). Soil gases and SAR measurements reveal hidden faults on the sliding flank of Mt. Etna (Italy). J. Volcanol. Geotherm. Res. 251, 27–40. doi:10.1016/j.jvolgeores.2012.08.010
Branca, S., Coltelli, M., and Groppelli, G. (2011). Geological evolution of a complex basaltic stratovolcano: Mount Etna. Italy. Ital. J. Geosci. 130. doi:10.3301/IJG.2011.13
Brogna, A., La Delfa, S., La Monaca, V., Lo Nigro, S., Morelli, D., Patane’, G., et al. (2007). Measurements of indoor radon concentration on the south-eastern flank of Mount Etna volcano (Southern Italy). J. Volcanol. Geotherm. Res. 165, 71–75. doi:10.1016/j.jvolgeores.2007.04.012
Burton, M., Neri, M., and Condarelli, D. (2004). High spatial resolution radon measurements reveal hidden active faults on Mt. Etna: Radon Measurements Reveal Faults on Etna. Mt. Etna. Geophys. Res. Lett. 31. doi:10.1029/2003GL019181
Capaldi, G., Cortini, M., Gasparini, P., and Pece, R. (1976). Short-lived radioactive disequilibria in freshly erupted volcanic rocks and their implications for the preeruption history of a magma. J. Geophys. Res. 81, 350–358. doi:10.1029/jb081i002p00350
Catalano, R., Immé, G., Mangano, G., Morelli, D., Aranzulla, M., Giammanco, S., et al. (2015b). In situ and laboratory measurements for radon transport process study. J. Radioanal. Nucl. Chem. 306, 673–684. doi:10.1007/s10967-015-4336-6
Catalano, R., Immé, G., Mangano, G., Morelli, D., and Aranzulla, M. (2015a). Radon transport: Laboratory and model study. Radiat. Prot. Dosim. 164, 575–581. doi:10.1093/rpd/ncv314
Chiodini, G., Cioni, R., Guidi, M., Raco, B., and Marini, L. (1998). Soil CO2 flux measurements in volcanic and geothermal areas. Appl. Geochem. 13, 543–552. doi:10.1016/S0883-2927(97)00076-0
Chiodini, G., Cioni, R., Pescia, A., Ponziani, F., Raco, B., and Taddeucci, G. (1989). Eruzione dell’Etna nell’autunno 1989: H2, CO e 222Rn nei gas del suolo in prossimità della frattura apertasi sul versante meridionale del vulcano. Boll. del Grup. Naz. Vulcanol. 1, 177–187.
Clocchiatti, R., Condomines, M., Guénot, N., and Tanguy, J. C. (2004). Magma changes at mount Etna: The 2001 and 2002-2003 eruptions. Earth Planet. Sci. Lett. 226, 397–414. doi:10.1016/j.epsl.2004.07.039
Condomines, M., Tanguy, J. C., and Michaud, V. (1995). Magma dynamics at Mt Etna: Constraints from U-Th-Ra-Pb radioactive disequilibria and Sr isotopes in historical lavas. Earth Planet. Sci. Lett. 132, 25–41. doi:10.1016/0012-821X(95)00052-E
Cothern, C. R., Lappenbusch, W. L., and Michel, J. (1986). Drinking-water contribution to natural background radiation. Health Phys. 50, 33–47. doi:10.1097/00004032-198601000-00002
D’Alessandro, W., and Vita, F. (2003). Groundwater radon measurements in the Mt. Etna area. J. Environ. Radioact. 65, 187–201. doi:10.1016/S0265-931X(02)00096-6
Darby, S., Hill, D., Auvinen, A., Barros-Dios, J. M., Baysson, H., Bochicchio, F., et al. (2005). Radon in homes and risk of lung cancer: Collaborative analysis of individual data from 13 European case-control studies. Br. Med. J. 330, 223. doi:10.1136/bmj.38308.477650.63
Falsaperla, S., Behncke, B., Langer, H., Neri, M., Salerno, G. G., Giammanco, S., et al. (2014). Failed” eruptions revealed by pattern classification analysis of gas emission and volcanic tremor data at Mt. Etna, Italy. Int. J. Earth Sci. 103, 297–313. doi:10.1007/s00531-013-0964-7
Falsaperla, S., Neri, M., Di Grazia, G., Langer, H., and Spampinato, S. (2017). What happens to in-soil radon activity during a long-lasting eruption? Insights from Etna by multidisciplinary data analysis. Geochem. Geophys. Geosystems 18, 2162–2176. doi:10.1002/2017GC006825
Farrar, C. D., Sorey, M. L., Evans, W. C., Howle, J. F., Kerr, B. D., Kennedy, B. M., et al. (1995). Forest-killing diffuse CO2 emission at Mammoth Mountain as a sign of magmatic unrest. Nature 376, 675–678. doi:10.1038/376675a0
Fiore, M., Ledda, C., Conti, G. O., Fallico, R., Sciacca, S., and Ferrante, M. (2011). Radon monitoring in groundwater from the Mt. Etna area. ISEE Conf. Abstr. 2011. doi:10.1289/isee.2011.01783
Galli, G., Mancini, C., and Quattrocchi, F. (2000). Groundwater, radon continuous monitoring system (α -scintillation counting) for natural hazard surveillance. Pure Appl. Geophys. 157, 407–433. doi:10.1007/s000240050006
Gauthier, P. J., Le Cloarec, M. F., and Condomines, M. (2000). Degassing processes at Stromboli volcano inferred from short-lived disequilibria (210Pb-210Bi-210Po) in volcanic gases. J. Volcanol. Geotherm. Res. 102, 1–19. doi:10.1016/S0377-0273(00)00179-7
Giammanco, S., Immè, G., Mangano, G., Morelli, D., and Neri, M. (2009). Comparison between different methodologies for detecting radon in soil along an active fault: The case of the Pernicana fault system, Mt. Etna (Italy). Appl. Radiat. Isot. 67, 178–185. doi:10.1016/j.apradiso.2008.09.007
Giammanco, S., and Sims, K. W. W. (2022). “Monitoring volcanic activity through combined measurements of CO2 efflux and (222Rn) and (220Rn) in soil gas,” in Isotopic constraints on earth system processes. Editors K. W. W. Sims, K. Maher, and D. P. Schrag (United States: Wiley), 167–202. doi:10.1002/9781119595007.ch7
Giammanco, S., Sims, K. W. W., and Neri, M. (2007). Measurements of 220Rn and 222Rn and CO2 emissions in soil and fumarole gases on Mt. Etna volcano (Italy): Implications for gas transport and shallow ground fracture. Geochem. Geophys. Geosystems 8. doi:10.1029/2007GC001644
Grasty, R. (1997). Radon emanation and soil moisture effects on airborne gamma-ray measurements. Geophys 62, 1379–1385. doi:10.1190/1.1444242
Health Protection Agency (2009). Radon and public health—report of the independent advisory Group on ionizing radiation. England: Documents of the Health Protection Agency.
İçhedef, M., Giammanco, S., Neri, M., Catalano, R., Immé, G., Morelli, D., et al. (2020). In soil radon anomalies and volcanic activity on Mt. Etna (Italy). J. Environ. Radioact. 218, 106267. doi:10.1016/j.jenvrad.2020.106267
Immè, G., Catalano, R., Mangano, G., and Morelli, D. (2014). Radon exhalation measurements for environmental and geophysics study. Radiat. Phys. Chem. 95, 349–351. doi:10.1016/j.radphyschem.2013.02.033
Immè, G., La Delfa, S., Lo Nigro, S., Morelli, D., and Patanè, G. (2005). Gas radon emission related to geodynamic activity on. Mt. Etna. Ann. Geophys. 48. doi:10.4401/ag-3180
Immè, G., La Delfa, S., Lo Nigro, S., Morelli, D., and Patanè, G. (2006a). Soil radon concentration and volcanic activity of Mt. Etna before and after the 2002 eruption. Radiat. Meas. 41, 241–245. doi:10.1016/j.radmeas.2005.06.008
Immè, G., La Delfa, S., Lo Nigro, S., Morelli, D., and Patanè, G. (2006b). Soil radon monitoring in the NE flank of Mt. Etna (Sicily). Appl. Radiat. Isot. 64, 624–629. doi:10.1016/j.apradiso.2005.12.007
Immè, G., and Morelli, D. (2012). “Radon survey in active volcanoes,” in Handbook of radon: Properties, applications and health. Editors Z. Li, and C. Feng (New York: Nova Science Publishers Inc.), 405–416.
Jobbágy, V., Altzitzoglou, T., Malo, P., Tanner, V., and Hult, M. (2017). A brief overview on radon measurements in drinking water. J. Environ. Radioact. 173, 18–24. doi:10.1016/j.jenvrad.2016.09.019
Johnová, K., Thinová, L., and Giammanco, S. (2014). Revealing the hidden faults in the SE flank of Mt. Etna using radon in-soil gas measurement. Radiat. Prot. Dosim. 160, 70–73. doi:10.1093/rpd/ncu092
Josse, B., Simon, P., and Peuch, V.-H. (2004). Radon global simulations with the multiscale chemistry and transport model MOCAGE. Tellus B Chem. Phys. Meteorol. 56, 339. doi:10.3402/tellusb.v56i4.16448
Kozłowska, B., Morelli, D., Walencik, A., Dorda, J., Altamore, I., Chieffalo, V., et al. (2009). Radioactivity in waters of Mt. Etna (Italy). Radiat. Meas. 44, 384–389. doi:10.1016/j.radmeas.2009.05.002
Kozłowska, B., Walencik-łata, A., Giammanco, S., Immè, G., Catalano, R., and Mangano, G. (2019). Radioactivity content in volcanic rocks and radionuclides transfer from rocks to groundwater at Mt. Etna volcano. Ann. Geophys. 62. doi:10.4401/ag-7549
Kozłowska, B., Walencik-Łata, A., Immè, G., Catalano, R., Mangano, G., Morelli, D., et al. (2016). Natural radioactivity content in groundwater of Mt. Etna’s eastern flank and gamma background of surrounding rocks. Ann. Geophys. 59. doi:10.4401/ag-6799
La Delfa, S., Agostino, I., Morelli, D., and Patanè, G. (2008). Soil radon concentration and effective stress variation at Mt. Etna (Sicily) in the period January 2003-April 2005. Radiat. Meas. 43, 1299–1304. doi:10.1016/j.radmeas.2008.02.004
La Delfa, S., Immè, G., Lo Nigro, S., Morelli, D., Patanè, G., and Vizzini, F. (2007). Radon measurements in the SE and NE flank of Mt. Etna (Italy). Radiat. Meas. 42, 1404–1408. doi:10.1016/j.radmeas.2007.06.004
La Delfa, S., Vizzini, F., and Patanè, G. (2010). Identification of radon anomalies at Mt. Etna (sicily) by using three different methods. Environ. Semeiot. 3, 1–16. doi:10.3383/es.3.1.1
La Delfa, S., Vizzini, F., and Patanè, G. (2012). Radon migration into different building types at medium and low south-eastern flank of Mt Etna (Sicily): Connection with the volcanic activity. Environ. Earth Sci. 66, 923–931. doi:10.1007/s12665-011-1302-7
Lambert, G., Bristeau, P., and Polian, G. (1976). Emission and enrichments of radon daughters from Etna Volcano magma. Geophys. Res. Lett. 3, 724–726. doi:10.1029/GL003i012p00724
Le Cloarec, M. F., Pennisi, M., Ardouin, B., Roulley, J. C. L., and Lambert, G. (1988). Relationship between gases and volcanic activity of Mount Etna in 1986. J. Geophys. Res. 93, 4477–4484. doi:10.1029/jb093ib05p04477
Le Cloarec, M. F., and Pennisi, M. (2001). Radionuclides and sulfur content in Mount Etna plume in 1983-1995: New constraints on the magma feeding system. J. Volcanol. Geotherm. Res. 108, 141–155. doi:10.1016/S0377-0273(00)00282-1
Mancini, S., Vilnitis, M., Todorović, N., Nikolov, J., and Guida, M. (2022). Experimental studies to test a predictive indoor radon model. Int. J. Environ. Res. Public Health 19, 6056. doi:10.3390/ijerph19106056
Marty, B., and Le Cloarec, M. F. (1992). Helium-3 and CO2 fluxes from subaerial volcanoes estimated from polonium-210 emissions. J. Volcanol. Geotherm. Res. 53, 67–72. doi:10.1016/0377-0273(92)90074-N
Morelli, D., Di Martino, S., Immè, G., La Delfa, S., Lo Nigro, S., and Patanè, G. (2006). Evidence of soil radon as tracer of magma uprising in Mt. Etna. Etna. Radiat. Meas. 41, 721–725. doi:10.1016/j.radmeas.2006.04.026
Morelli, D., Immé, G., Altamore, I., Aranzulla, M., Cammisa, S., Catalano, R., et al. (2011a). “Radiation measurements as tool for environmental and geophysics studies on volcano-tectonic areas,” in Nuovo cimento della Società italiana di Fisica C (Italy: Società Italiana Di Fisica), 155–165. doi:10.1393/ncc/i2011-10823-0
Morelli, D., Immè, G., Altamore, I., Cammisa, S., Giammanco, S., La Delfa, S., et al. (2011b). Radionuclide measurements, via different methodologies, as tool for geophysical studies on Mt. Etna. Nucl. Instrum. Methods Phys. Res. Sect. A Accel. Spectrom. Detect. Assoc. Equip. 652, 911–914. doi:10.1016/j.nima.2011.01.172
Neri, M., Behncke, B., Burton, M., Galli, G., Giammanco, S., Pecora, E., et al. (2006). Continuous soil radon monitoring during the July 2006 Etna eruption. Geophys. Res. Lett. 33, L24316. doi:10.1029/2006GL028394
Neri, M., Ferrera, E., Giammanco, S., Currenti, G., Cirrincione, R., Patanè, G., et al. (2016). Soil radon measurements as a potential tracer of tectonic and volcanic activity. Sci. Rep. 6, 24581. doi:10.1038/srep24581
Neri, M., Ferrera, E., Giammanco, S., Patanè, G., and Zanon, V. (2014). Un metodo per riconoscere faglie attive sepolte mediante misure areali di radon dal suolo. Geol. dell’Ambiente ISSN 1591-5352, 1–8.
Neri, M., Giammanco, S., Ferrera, E., Patanè, G., and Zanon, V. (2011). Spatial distribution of soil radon as a tool to recognize active faulting on an active volcano: The example of Mt. Etna (Italy). J. Environ. Radioact. 102, 863–870. doi:10.1016/j.jenvrad.2011.05.002
Neri, M., Giammanco, S., and Leonardi, A. (2019). Preliminary indoor radon measurements near faults crossing urban areas of Mt. Etna volcano (Italy). Front. Public Heal. 7, 105. doi:10.3389/fpubh.2019.00105
Neri, M., Guglielmino, F., and Rust, D. (2007). Flank instability on Mount Etna: Radon, radar interferometry, and geodetic data from the southwestern boundary of the unstable sector. J. Geophys. Res. Solid Earth 112. doi:10.1029/2006JB004756
Pellegriti, G., De Vathaire, F., Scollo, C., Attard, M., Giordano, C., Arena, S., et al. (2009). Papillary thyroid cancer incidence in the volcanic area of Sicily. J. Natl. Cancer Inst. 101, 1575–1583. doi:10.1093/jnci/djp354
Pinault, J. L., and Baubron, J. C. (1996). Signal processing of soil gas radon, atmospheric pressure, moisture, and soil temperature data: A new approach for radon concentration modeling. J. Geophys. Res. Solid Earth 101, 3157–3171. doi:10.1029/95jb03121
Quattrocchi, F., Di Stefano, G., Pizzino, L., Pongetti, F., Romeo, G., Scarlato, P., et al. (2000). Geochemical Monitoring System II prototype (GMS II) installation at the “Acqua Difesa” well, within the Etna region: First data during the 1999 volcanic crisis. J. Volcanol. Geotherm. Res. 101, 273–306. doi:10.1016/S0377-0273(00)00177-3
Rodríguez-Martínez, A., Torres-Durán, M., Barros-Dios, J. M., and Ruano-Ravina, A. (2018). Residential radon and small cell lung cancer. A systematic review. Cancer Lett. 426, 57–62. doi:10.1016/j.canlet.2018.04.003
Seidel, J. L., and Monnin, M. (1984). Mesures de Radon-222 dans le sol de l’Etna (sicile): 1980-1983. Bull. Volcanol. 47, 1071–1077. doi:10.1007/BF01952363
Siniscalchi, A., Tripaldi, S., Neri, M., Giammanco, S., Piscitelli, S., Balasco, M., et al. (2010). Insights into fluid circulation across the Pernicana Fault (Mt. Etna, Italy) and implications for flank instability. J. Volcanol. Geotherm. Res. 193, 137–142. doi:10.1016/j.jvolgeores.2010.03.013
Terray, L., Gauthier, P. J., Breton, V., Giammanco, S., Sigmarsson, O., Salerno, G., et al. (2020a). Radon activity in volcanic gases of Mt. Etna by passive dosimetry. J. Geophys. Res. Solid Earth 125. doi:10.1029/2019JB019149
Terray, L., Gauthier, P. J., Salerno, G., Caltabiano, T., La Spina, A., Sellitto, P., et al. (2018). A new degassing model to infer magma dynamics from radioactive disequilibria in volcanic plumes. Geosci 8, 27. doi:10.3390/geosciences8010027
Terray, L., Royer, L., Sarramia, D., Achard, C., Bourdeau, E., Chardon, P., et al. (2020b). From sensor to cloud: An IoT network of radon outdoor probes to monitor active volcanoes. Sensors Switz. 20, 2755. doi:10.3390/s20102755
Vaupotič, J., Žvab, P., and Giammanco, S. (2010). Radon in outdoor air in the Mt. Etna area. Italy. Nukl. 55, 573–577.
Vizzini, F., and Brai, M. (2012). In-soil radon anomalies as precursors of earthquakes: A case study in the SE slope of Mt. Etna in a period of quite stable weather conditions. J. Environ. Radioact. 113, 131–141. doi:10.1016/j.jenvrad.2012.05.027
Wilhelm, E., Battino, R., and Wilcox, R. J. (1977). Low-pressure solubility of gases in liquid water. Chem. Revs. 77, 219–262. doi:10.1021/cr60306a003
Woith, H. (2015). Radon earthquake precursor: A short review. Eur. Phys. J. Spec. Top. 224, 611–627. doi:10.1140/epjst/e2015-02395-9
Keywords: radon, Mt. Etna, volcano monitoring, human health, soil gas, indoor pollution, magmatic degassing, faults
Citation: Giammanco S, Bonfanti P and Neri M (2023) Radon on Mt. Etna (Italy): a useful tracer of geodynamic processes and a potential health hazard to populations. Front. Earth Sci. 11:1176051. doi: 10.3389/feart.2023.1176051
Received: 28 February 2023; Accepted: 09 May 2023;
Published: 17 May 2023.
Edited by:
Yi-Xiang Chen, University of Science and Technology of China, ChinaReviewed by:
Silvia Massaro, Università degli Studi Aldo Moro, ItalyFabio Dioguardi, University of Bari Aldo Moro, Italy
Copyright © 2023 Giammanco, Bonfanti and Neri. This is an open-access article distributed under the terms of the Creative Commons Attribution License (CC BY). The use, distribution or reproduction in other forums is permitted, provided the original author(s) and the copyright owner(s) are credited and that the original publication in this journal is cited, in accordance with accepted academic practice. No use, distribution or reproduction is permitted which does not comply with these terms.
*Correspondence: Marco Neri, marco.neri@ingv.it