Effects of wildfire on soils: field studies and modelling on induced underground temperature variations
- Interuniversity Department of Regional and Urban Studies and Planning (DIST), University of Torino, Turin, Italy
Wildfire statistics report an increasing number of deaths, damages, and force society to face exceptional repair costs also due to secondary effects such as landslides, soils erosion and water quality problems. Literature agrees that the extent of the thermal disturbance of the soil due to wildfire is strongly dependent on fire intensity, on its duration and recurrence, as well as on fuel load, and soil properties. The unpredictability of these phenomena is one of the main challenges for scientists trying to study their characteristics. Degradation of the biological, chemical, and physical properties of forest soils due to the wildfires can reduce their capacity to function fully, with such effects either temporary or permanent. Direct measurements of the effects of these phenomena are difficult to be collected in the field, especially when the area affected by fires is a wilderness. In this study, controlled fires reproducing real conditions of wildfire affecting the Susa Valley (NW Italy) in 2017, which induced intense erosion and debris flows, have been monitored both above and below the ground surface. Moreover, numerical simulations based on real data increased the know-how to reproduce real changes on the underground. The small-scale fire simulations have allowed to verify how the depth affected by significant increases in temperatures is truly pellicular. Field tests show that at −2 cm depth temperature never exceed 70°C, meaning that it usually does not affect soil components or properties. The results of the study suggest that temperature variations in the subsoil are very localized and limited. If confirmed by further studies, the processes inferred to produce large ground effects on slopes after wildfires should be re-considered.
1 Introduction
Fires are considered a destructive factor in most forest ecosystems of tropical, temperate, and boreal areas (García-Llamas et al., 2019) and are viewed as global phenomena affecting most land areas. The extent of soil disturbance by fire is largely dependent on fire intensity, duration and recurrence, fuel load, and soil characteristics (Agbeshie et al., 2022). Low-intensity fires with ash deposition on soil surfaces cause changes in soil chemistry, including the increase in available nutrients and pH. On the other hand, high intensity fires are noted for the complete combustion of organic matter and result in severe negative impacts on forest soils (Certini, 2005). These fires also result in nutrient volatilization, the break down in soil aggregate stability, an increase of the bulk density and of the hydrophobicity of soil particles which leads to decreased water infiltration, followed by an increased erosion and destruction of the soil biota. High soil heating (>120°C) due to high-intensity forest fires is detrimental to the soil ecosystem, especially to its physical and biological properties.
Since future scenarios linked to the effect of climate change predict that forest fire frequency and severity will likely increase, as will the extreme rainfall events, wildfires may constitute a looming issue in the Alpine region too. For example, in 2017, an unusually severe wildfires season occurred in NW Italy and especially in Piedmont (Western Alps). One of the areas most affected was the Susa Valley, where wildfires hit the south facing slope for more than 4,000 hectares: it had a considerable media coverage, as the smoke from the fires reached Turin, the largest city in the area (Vacha et al., 2021). The following spring season, a series of debris-flows took place, the largest of them hitting the town of Bussoleno (Figure 1), located in the valley floor. This came from the Comba delle Foglie secondary watershed (Figure 2) and caused considerable damage to buildings and infrastructures.
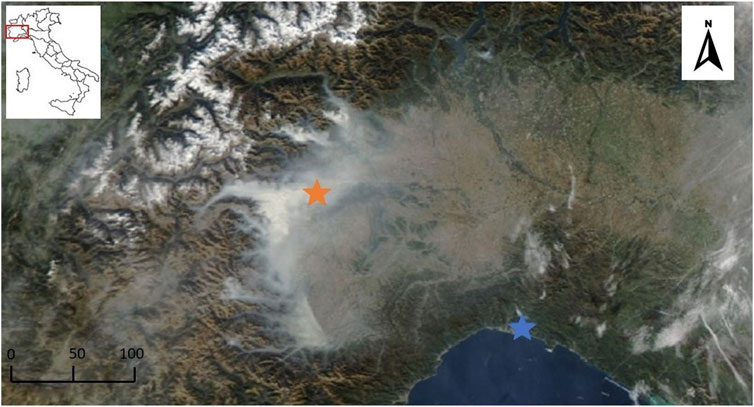
FIGURE 1. Satellite image acquired in the visible band by the MODIS sensor on NASA’s EOS Aqua polar satellite, 11:55 UTC on 25/10/2017 showing western alps and smoke from wildfires. Stars: orange = Torino and blue = Genova, about 150 km apart.
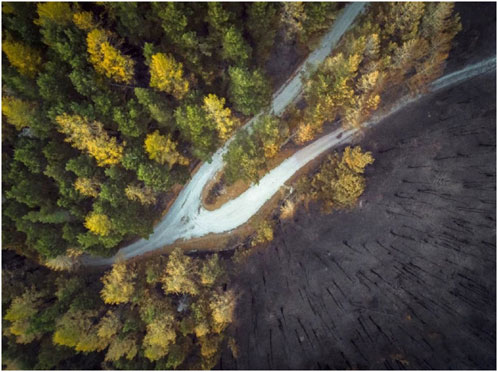
FIGURE 2. Aerial view of the juxtaposition between burnt and unaffected areas in a pine forest (2017), Susa Valley, Western Italian Alps (Courtesy of Gipix Drone).
The fire occurrence is mainly determined by low soil litter moisture, and by the presence of an abundant ignition source. In this case, fuels humidity values can depend on precipitation, temperature, wind and relative humidity. Once the fire has started, the intensity and spreading potential of a forest fire is influenced by other variables, such as vegetation type and structure, fuel amount, topography, wind, slope aspect, humidity (Valsecchi et al., 2010; Pezzatti et al., 2013; Girardin and Terrier, 2015; Fréjaville et al., 2016). Therefore, fire effects on soil constitute a very broad spectrum, also within the same burned area. They can be subdivided into physical, chemical, and biological modifications (Neary et al., 1999; Úbeda and Outeiro, 2009; Mataix-Solera et al., 2011), varying in magnitude and duration depending on several factors, among which the most important is fire severity that in turn depends on fire intensity. As a result of this great number of factors, and of their variability, the effect of a fire can spans from little or negligible damage to the most extreme scenario where all aboveground organisms are erased.
Experimental tests of controlled burning simulating small fires have been conducted in an area with similar characteristics to the real case study in Susa Valley (NW Italy), where several wildfires followed by an intense erosion and debris flows events occurred. The aim was to understand the heat transfer in the subsoil and the temperature reached by the soil during wildfires. In this study, thermocouples above and below the ground surface at different depths, have been placed to record temperatures. During controlled fires, different fuels have been reproduced in typology and quantity, as in natural pre-fire conditions; the burning phase has been followed till natural shut-down. Based on these results, numerical models about effect of wildfire on soil and subsoil temperature have been refined.
2 Fire effects on the underground
Wildfires disturb normal hydrologic and soil conditions. Burned forest soils often repel water, causing fire-induced reductions in infiltration, leading to an increased likelihood of flooding and runoff-generated debris flows. Post-fire debris flows can be lethal hazards, resulting in high numbers of fatalities (Rengers et al., 2020).
Heat conduction and convection are the most relevant processes responsible for soil heating during wildfires, while radiation contribution is marginal. The way these processes affect soil depends mostly on quantity of released energy and duration of heating (i.e., fire intensity). This, in turn, is related to the amount of available fuel and type of fire (Mataix-Solera et al., 2011): crown fires are usually large scale, fastmoving, wind-driven and usually uncontrollable. They often have a deep flame front. Despite this, fire front usually passes rapidly through tree canopy causing little soil heating, unless sufficient fuel is accumulated from forest floor to crowns. Slowly spreading surface fires, on the other hand, are usually small-scale and characterized by a thick flame front. They can combust a large part of forest biomass and can substantially transfer heat to the soil. Wind-driven grass fires spread quickly and sometimes over large areas. In these types of fires the available biomass is limited, so their effect on soil is often irrelevant. Smoldering fires are flame-free, slow moving and long lasting unimpressive, but frequently have long burnout times. Many authors agree that this affects considerably both the soil and the subsoil, releasing a remarkable amount of heat; direct measures of temperature in the underground related to these thermal perturbances, are very rare in literature.
It is often very hard to assess the fire intensity in the post-fire situation, being unknown many of the variables which may have played a role, and the environmental conditions that were present prior to the fire. One of the proxies for the estimation of the amount of energy released in the soil is the depth of burn as reflected in the amount of surface litter, organic soil horizons, and woody fuel consumed (Ryan, 2002; Neary et al., 2008). For example, the depth of lethal heat penetration into the soil (approximately 60°C) can be expected to increase with increasing depth of surface organic material that is burned, and with duration of burning. Burn depth can be classified, if no other information is available, based on visual observation of degree of fuel consumption and charring on residual plant and soil surfaces (Ryan and Noste, 1985). Neary and Leonard (2020) give a summary of the relationships between depth of burn and charring of plant materials in grassland, which can be used as a guide for classification.
Soil physical and chemical alteration due to fire effect are usually related to a transition to a more friable, less cohesive, and more erodible soil. This can be related to the combustion of organic matter in soil that results in a decrease in aggregate stability. Moreover, literature reports modification of other parameters such as particle-size distribution, bulk density, plasticity and elasticity. (DeBano et al., 1998; Hubbert et al., 2012; Parise and Cannon, 2012). As an example, bulk density is reported to increase as organo-mineral aggregates collapse because of pore sealing by the finer particles (Giovannini et al., 1988). Reduction or destruction of fungal and microbial activity, which produce cohesive compound as hyphae, are reported as a destabilizing effect (Shakesby and Doerr, 2006). The amount of the modifications reported is correlated with type of soil and temperature reached within burned soil horizon (Guerrero et al., 2001).
Aggregate stability is a commonly used parameter for assessing the resistance of the soil to external factors. In literature, effect of fire on this feature is apparently contradictory: in most cases, fire leads to fragmentation of aggregates and loss of stability, while in some other studies no significant changes have been reported; in a few cases an increase in the aggregate stability has been observed (Mataix-Solera et al., 2011 and references therein, Figure 3). High temperature can fuse soil particle, generating a coarser texture with less cohesive aggregates; in addition, if temperature reaches a value above 460°C clays lose the hydroxyl group which promotes a soil structure weakening (De Bano et al., 1998; Neary et al., 1999; Parise and Cannon, 2012). In some soils, heat exchanged lead to a new aggregation of particles by recrystallization of Fe and Al oxides and thus the wettability of aggregate surfaces may be reduced, causing an increase in aggregate stability (Giovannini and Lucchesi, 1983; Giovannini, 1994; Mataix-Solera and Doerr, 2004). Another direct effect of the fire is the change in soil wettability: fire, depending on the amount/type of fuels and the temperature reached, can produce water repellent layers in non-repellent soil, or modify either positively and negatively pre-existing water repellent attitude (Certini, 2005; Shakesby and Doerr, 2006). Water repellency has been recorded in many unburned soils, such as eucalypt and coniferous forest (Doerr et al., 2000; Shakesby et al., 2007) and in chaparral environment of southern California (Cawson et al., 2016). One of the physico-chemical processes responsible for creation of new repellent layers is the volatilization, followed by the condensation, of hydrophobic organic substances available in litter and topsoil. The process is dependent from temperature, oxygen availability and duration of heating. Laboratory tests shows little effects for temperatures lower than 175°C, maximum effect for temperature from 175°C to 200°C and destruction of water repellency from 280°C to 400°C (DeBano, 2000). As reported in Doerr et al. (2004), in eucalypt forest the maximum water repellency has been found after heating soil at temperature from 250°C to 280°C, while an additional five-minute heating from 310°C to 340°C has been able to destroy hydrophobic effect. Doubling heating time, a range of temperature from 290°C to 330°C has been enough for erase water repellency. Results from laboratory tests described in literature, have proven that is very difficult to replicate real field conditions: some authors reported an increase in water repellency for temperature above 400°C, others a decrease of the same parameter for temperature below 200°C. Factors such as soil moisture, oxygen availability, pre-fire conditions and fuel characteristics may represent uncontrolled variables (Vadilonga et al., 2008; Stoof et al., 2011; Cawson et al., 2016).
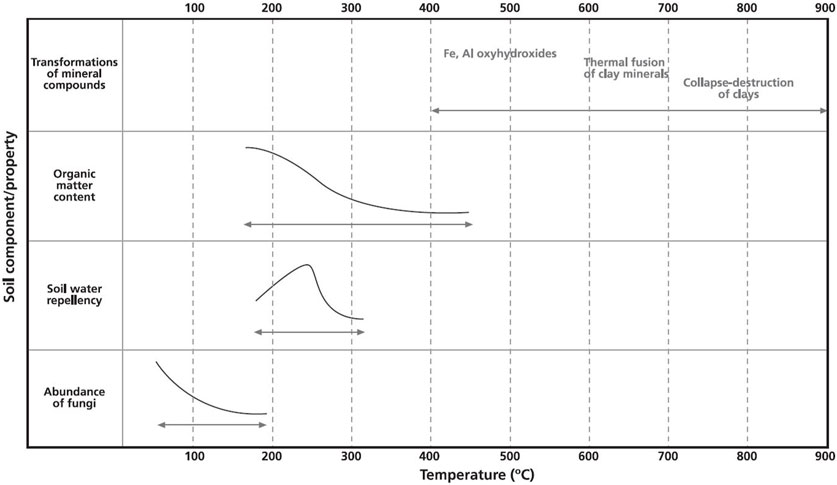
FIGURE 3. Main soil properties changes at different temperature (Mataix-Solera et al., 2011).
Other parameters are affected by fire occurrence: soil pH shows a tendential increase due to organic acid’s denaturation. Significant changes are reported at high temperatures (>450°C). In contrast, fire induced increase in pH is negligible in carbonated-buffered soils (Certini, 2005). Exchangeable capacity can generally decrease, while electrical conductivity can slightly increase (Badia and Marti, 2003; Certini, 2005). In conclusion, the greater modifications are likely to occur at temperature higher than 150°C–200°C.
2.1 Field observations
On-site surveys in the burned areas have been performed, together with a task force consisting of technicians of the Piedmont Region and some other local authorities, involved for preparing an Emergency Plan for recovery intervention aimed at individuating areas with a higher priority, because with a higher hydrogeological risk due to the fire. Surveys allowed evaluating the main effects of fire such as macroscopic and visual effects on the landscape, focused on the vegetations and ground.
In the Comba delle Foglie watershed, fire affected an area already severely burned in the past, which led to a modification on the ability to withstand weather forcing and cope with enhanced erosional and depositional dynamics. In particular, this area showed the most evident signs of post-fire geomorphic dynamics such as an extensive areal and channeled erosion, especially in the upper part of the catchment where traces of flows transit have been found down to the valley outlet. The arboreal cover also had the greatest damages: large area of coniferous forest showed an almost total plant mortality and a complete biomass consumption from the ground to the crown of the trees (Figure 4).
Field surveys allowed also to define the erosivity dynamics (Mandrone et al., 2023; Vacha et al., 2023). Soil modification observed in the field are generally shallow (less than 10 cm) and all of them correlated with the overland flow dynamics. Rills, gullies and zero order streams incision are found to be more evident in high fires severity sectors, especially where the tree canopy is severely reduced. Surveys conducted in the uppermost part of watershed, showed evident traces of overland flow coalescing into rills, and then into the main drainage line at the watershed bottom. In the lower part of the basin, characterized by a generally higher percentage of residual vegetation cover, rills and little gullies were detected. In the main channel the erosion, increasing downstream, was marked by channel incision with steep banks and frequently exposed bedrock; down the channel, sorting of the deposit decreased while the amount of matrix increased.
The erosive processes following fires, favored by an increase in surface runoff, contribute substantially to the availability of mobilizable material that can be conveyed to the drainage network and give rise to mass transport phenomena.
Among post fire effects, hydrophobic spots (not generalized), a general abundance of coarse-grained materials typical of undeveloped soils as well as the complete obliteration of shrubs density and of the grass coverage, have also been observed. Concerning the large ash production, as one of the most obvious effects of the studied post-fire event, the great amount of ash means a large fuel load and it is believed to being capable to affect infiltration and runoff generation.
3 Experimental equipment
Experimental tests of controlled burning, simulating a small-scale fire, has been conducted by igniting a known amount of fuel on top of a selected soil. The purpose is to evaluate relationships between combustion of biomass on ground and transfer of heat in subsoil. In fact, most of physic-chemical modifications occurring in soil depend on temperature gradient and on its temporal duration.
The amount of energy released by combustion depends on the type of fire, the type and the amount of fuel, and the amount of the organic matter in the soil. The heat transfer in the subsoil depends on the maximum combustion temperature, its duration and the thermophysical characteristics of the underground soils within which the heat pulse propagates (thermal conductivity, volumetric heat capacity, temperature, moisture, etc.). Among these, the most important physical quantity is the thermal conductivity: it defines the ability of a material to transfer heat, corresponding to the amount of heat transferred from a body per unit area. Mathematically, it is expressed by Fourier’s Law of thermal conduction (Eq. 1):
where q is the heat flow in the direction of X; and T is the temperature. The coefficient λ, is the thermal conductivity (Sobota, 2014).
The effective thermal conductivity of soil depends on the degree of saturation, grain size, porosity, mineral composition, and organic content. In fact, a heterogeneous and multiphase granular medium it is linked to thermal conductivity of single phases that make up the material, that is conductivity of grains and intergranular fluids (water and air). As an example, typical ranges for quartz mineral which is usually effective in increasing thermal conductivity (Chicco et al., 2019), are between 6.15 and 11.3 W m−1 K−1, while for water and dry air (at 20°C) are respectively of 0.58 and 0.024 W m−1 K−1 (Clauser and Huenges, 1995; Bristow, 1998). Effective thermal conductivity decreases with decreasing grain size and this reduction is more significant for fine grain materials (Midttømme and Roaldset, 1998; Huetter et al., 2008). On the contrary, it increases with a decrease in porosity due to a greater fraction of soil material, while decreasing with an increase in organic matter content. In addition, effective thermal conductivity shows a nonlinear dependence with temperature: according to Campbell et al. (1994) thermal conductivity of “moist soil” can be 3 to 5 times greater at 90°C than ambient value, while temperature has a more limited effect on “dry soils”.
Commonly—although heat in moist soil is transported faster and penetrates deeper than in dry soils—latent heat of vaporization prevents soil temperature from exceeding 95°C until water completely vaporizes; once this threshold is passed, the temperature then typically rises to 200°C–300°C or exceeds these values, if heavy fuels are present (Tecle and Neary, 2015).
In this work, field tests were conducted in the Alps, on steep slopes consisting of little evolved soils, poor in organic matter which favor erosive dynamics and scarce accumulation of nutrients. Average grain-size of these soils is generally coarse, and porosity is high; moreover, fires generally started after prolonged dry and windy periods, so it can be assumed with reasonable confidence that humidity level of the soils in first horizons was also very low. In fact, the thermal conductivity measures in the summer season show extremely low values, with an average of 0.488 W/mK and 0.734 W/mK for the Bussoleno and Mompantero areas, respectively.
The fires have been conducted in the field on a 0.4 × 0.4 m natural soil test area (Figure 5) varying the amount of fuel disposed on top of it; the soil is constituted by a sand with gravel and silt (Gravel = 18.28%; Sand = 63.22%; Silt = 17.88%; Clay = 0.62%), with a porosity of 0.58, average saturation of 0.61 and an average apparent density of 1.46 kg/m3.
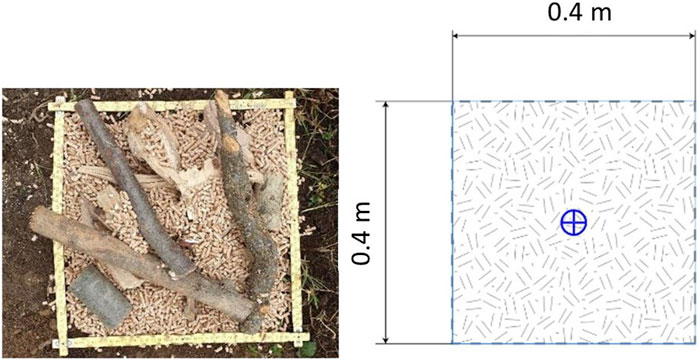
FIGURE 5. Picture (left), and planimetric view of the simulated fire setup (right). The blue cross (right) indicates the vertical projection of the thermocouples position.
Thermal conductivity of the soil before each experiment has been measured by using the commercial KD2Pro apparatus, a thermal property analyzer developed by Decagon Devices that fully complies with the ASTM D5334 and IEEE 442 standards (ASTM 2014; IEE-442 2003). It relies on the transient line heat source (TLS) methods (Kluitenberg et al., 1993) based on the generation of heat at a constant rate by heated wire, and assuming an infinitely long and thin line source inside an infinite and homogeneous medium (Giordano et al., 2019). Among the available sensors, the dual needle SH-1 sensor (1.3 mm diameter × 3 cm long, 6 mm spacing) has been used as it is the more suitable for solid and granular soils; it allows measuring temperature, volumetric specific heat, thermal diffusivity, and thermal conductivity (accuracy ± 10%). During measurements, a specific measuring time of 600 s has been adopted which allows recording 300 s of heating and 300 s of cooling with a 10 s sampling interval. Temperature readings have been corrected based on the ambient temperature and on the sensor geometrical setup through the Eq. 2:
where:
T*, is the temperature recorded by the needle probe, obtained by subtracting the ambient temperature at time 0, multiplying by 4π and dividing by the heat per unit length q.
Results have been fitted to the Eqs 3, 4, using a non-linear least square procedure (Marquardt, 1963):
where:
Ei is the exponential integral; b0, b1 and b2 are the constants to be fit; T0 is the temperature at the start of the measurement and q is the heat input.
Thermal conductivity (λ) and diffusivity (D) are then computed by fitting Eq. 3 to the transformed data, as follow (Eqs. 5, 6):
The correct values of b0, b1 and b2 minimize the sum of squares of error between the equations and the measurements.
The fuel used in the experiment is composed by dry beech wood pellets with a calorific power of 4.5 KWh/kg and dry beech wooden sticks with a calorific power of 4.0 KWh/kg. Before each experiment, a precise amount of fuel (either pellets or a pellets-wood mixture) has been weighted, then distributed to the soil surface after grass removal. The fires have been ignited in different position of the fuel layer. The tests have been conducted with a fuel load of 9.37 kg/m2, 21.87 kg/m2 and 28.12 kg/m2 respectively, approximately equivalent to the dry mass of litter sampled in Mompantero area of about 18 kg/m2.
Six type of K thermocouples (flexible type, 2 mm diameter, Special class, MgO coating, range: 0°C–1100°C; Resolution = 0.1°C) have been placed to record the temperature variations (Figure 6). The first thermocouple - which records air temperature - was put just above the fuel layer. The second one has been placed on the ground-fuel interface. The other four thermocouples have been inserted horizontally into the soil aimed at providing measurements of temperature changes over time, along a vertical axis at different depths (−2, −4, −6 and −8 cm). The thermocouples have been carefully inserted into the soil after digging a 10 cm vertical cut in the soil, at about 15 cm from the boundary of the 0.4 m × 0.4 m experimental boundary. All the thermocouples have been connected to the data logger (Omega OM-HL-EH-TC) and measurement has been made with a 5 s time step starting from the ignition time and lasting for about 5 h. Experiments have been conducted with an air temperature varying between 10°C and 25°C. Initial temperatures of the fuel and soil before the fires therefore differed a little bit from one experiment to another. The complete combustion of the fuel has been reached after each experiment.
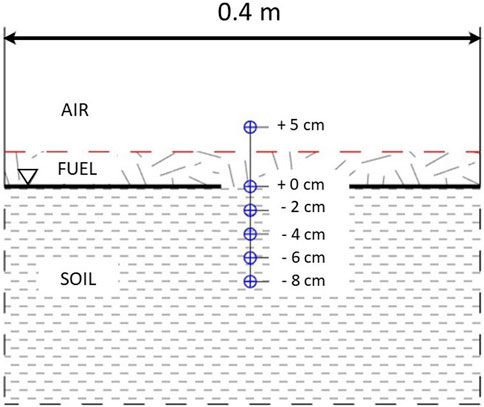
FIGURE 6. Vertical cross section of the simulated fire setup. The blue crosses represent the position of the six thermocouples.
4 Results
4.1 Experimental tests
Results from the three experimental tests as described in Section 3, are reported in Table 1 where the mean thermal conductivity values and the relative temperature for each experiment are shown. Before starting the burning phase, a series of four thermo-physical measurements has been conducted along each side of the test site aimed at validating the obtained data. Therefore, through the simple insertion of the needle probe into the undisturbed ground, has been possible to obtain these data and to take a final average value. Furthermore, main results obtained from the three tests are summarized in Table 2, while Figure 7 represents temperature profiles over the time for each thermocouple at different depth in the subsoil, based on the three different amounts of fuel used during the burning phase.
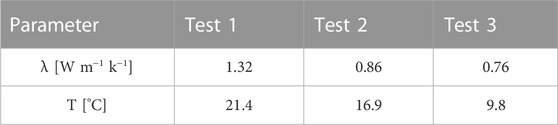
TABLE 1. Mean Thermal Conductivity (W m−1 k−1) and Temperature (°C) values, for the three conducted tests.
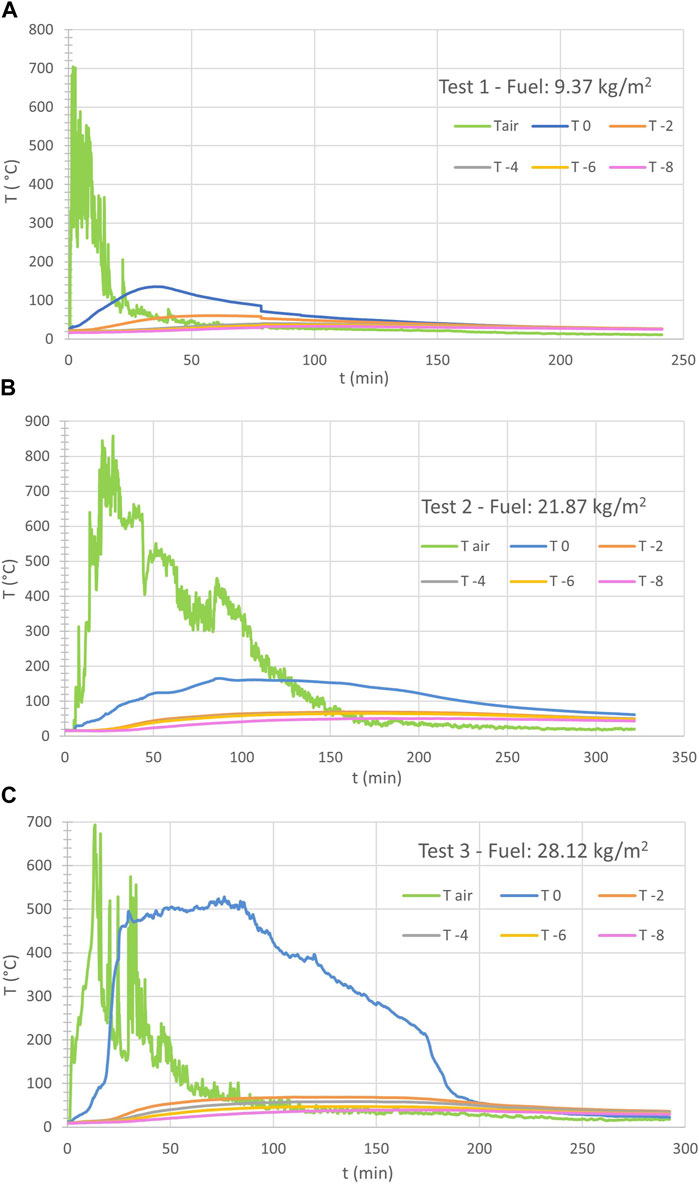
FIGURE 7. Temperature (°C) over time (min) recorded by six thermocouples placed above the ground surface (Tair), at surface (T0), and at different depths below the surface (−2 cm, −4 cm, −6 cm, −8 cm), based on the three different amounts of fuel used during the burning phase: 9.37 kg/m2 (A), 21.87 kg/m2 (B), 28.12 kg/m2 (C).
Test 1, conducted with a fuel load of 9.37 kg/m2, results in a maximum temperature registered at the soil surface of 135.9°C, corresponding to a maximum value registered at −2 cm depth of 61°C; maximum temperatures registered at −4, −6 and −8 cm from the surface are of 40.4°C, 37.0°C and 33.5°C respectively. Test 2, conducted with a fuel load of 21.87 kg/m2, results in a maximum temperature registered at the soil surface of 165.5°C, corresponding to a maximum value registered at −2 cm depth of 69.7°C; maximum temperatures registered at −4, −6 and −8 cm from the surface are 66.3°C, 65.4°C and 51.0°C respectively. Test 3, conducted with a fuel load of 28.12 kg/m2, results in a maximum temperature registered at the soil surface of 528.5°C, corresponding to a maximum value registered at −2 cm depth of 68.8°C; maximum of temperatures registered at −4, −6 and −8 cm from the surface are 58.6°C, 47.3°C and 39.4°C respectively. In this last case, the behavior of the interface between air and soil is quite different, reaching a much higher temperature: probably a piece of burning embers directly touched the thermocouple.
4.2 Numerical modelling
Numerical modelling has been characterized by a triangular super mesh able to reproduce complex shapes as well as to easily change the level of refinement. A global refinement of the entire mesh has been set up with a 0.2 m for polygons and point target size, as well as a point gradation of 2. As specified in previous works (Chicco and Mandrone, 2022a; Chicco and Mandrone, 2022b) a further mesh smoothing has been done, to avoid irregular shaped elements and obtuse-angled triangles. A further check for obtuse angles and triangles violating the Delaunay criterion, has also been conducted. The equation system solver used for this modelling is the “Standard iterative” method, which provides a robust and flexible setting; in this case, the matrix system is solved for the given discretization grid as it is. Iterative solvers can be selected for the symmetric (flow) and unsymmetric (transport) equations systems. The system uses preconditioned conjugate -gradient (PCG) solver for flow, and a BICGSTABP-type solver for transport. Calculations have been computed on each active node of the finite element mesh and interpolated within them. Once the model has been discretized in 2D, a 3D model with prismatic elements was obtained using the “3D layer configuration” tool. The modelled area has been built as a rectangular shape consisting of 13 layers on a 1.2 m × 1.2 m × 0.22 m mesh, divided by a total of 14 slices at different depths, from + 0.003 m above the ground level to −0.22 m below the ground level (Figure 8).
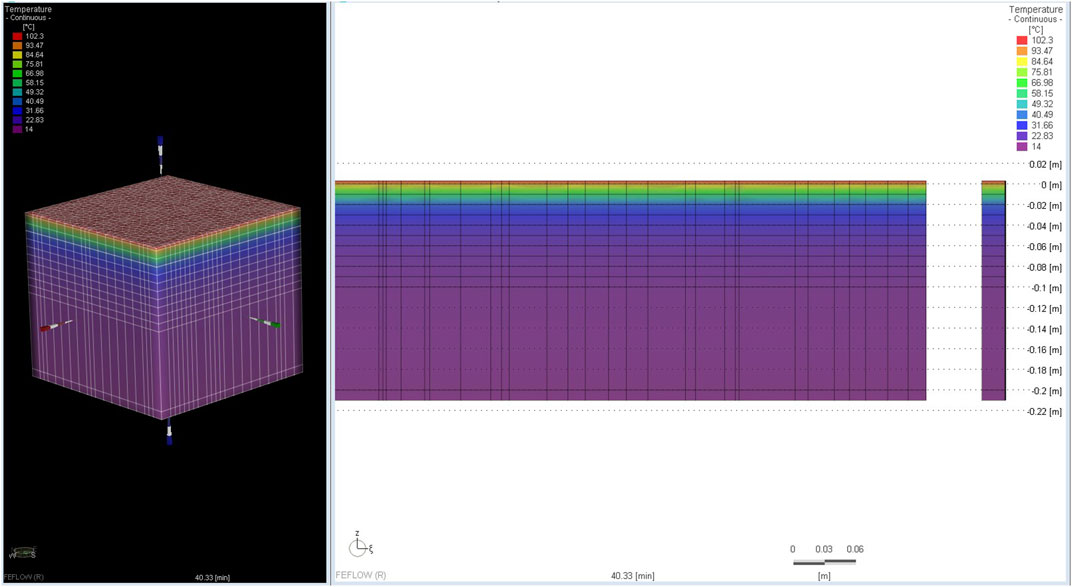
FIGURE 8. 3D domain (left) and 2D section view (right): the two figures are out of scale, for a vertical scale change. On the right, the 3D domain is divided by 14 slices as well represented in the 2D section on the left.
Experiment n. 2 (fuel load = 21.87 kg/m2) has been modelled in back analysis for deepening the comprehension of the heat propagation in the subsoil. A full 3D meshed model has been implemented in the software FeFlow (DHI), consisting in a finite simulation domain (0.5 m × 0.5 m × 0.213 m) subdivided in slices at progressive depths (Figure 9). The simulating domain is considered homogeneous (monophase, porosity = 0) and characterized by a volumetric heat capacity equal to 2.5 MJ m−3 k−1. The uppermost layer simulates the heat source, and its temperature follows the heating curve of the uppermost thermocouple (in the air). The layer 2 and 3 represent the ground level and the level at -2 cm below the ground; they are characterized by a time dependent thermal conductivity value, which has been retrieved through a trial-and-error procedure to fit the experimental data. Based on the general theory for which the thermal conductivity increases with the temperature, different curves with thermal conductivity values have been used, aimed at finding the value as close as possible to the real one. A constant thermal conductivity value equal to 1.6 W m−1 k−1, has been set for all the other layers. The bottom boundary conditions represent a constant temperature value equal to 14°C. The temperature profile at progressive time steps is evaluated by logging data in correspondence of six control points positioned at the same experimental thermocouples’ depth (0, −2, −4, −6, −8, −10 cm respectively). Figure 10 shows a frame of the simulation after 150 min from the initiation (approximately at the end of the maximum of the fire).
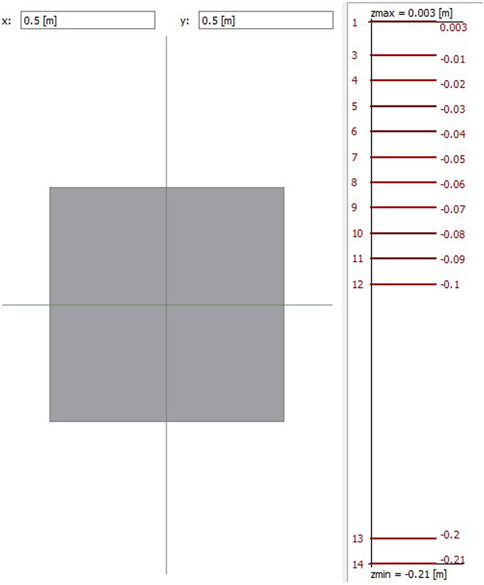
FIGURE 9. Geometrical parameters used to realize the FeFlow 3D model: along X and Y-axis (left), and along Z-axis (right).
5 Discussion
The comparison of the field observations of the post-fire conditions in Susa Valley (Comba delle Foglie watershed, NW Italy) with tests conducted through simulation of small-scale fires made it possible to confirm, albeit empirically, the accuracy of the experimental data as well as to reconstruct the heat transfer in the first centimeters of the subsoil, as occurs after a fire.
Temperatures of the flame at the ground surface and at −2, −4, −6, and −8 cm depth, were recorded both during the controlled fires and their cooling down phases. Results show a temperature increase at −2 cm depth of only 53°C. Even a fuel load greater than 30% gives a temperature rise of a few more degrees. At the maximum depth investigated during the field simulations, given the experimental conditions, the maximum temperature increase amounted at around 35°C. These temperatures are in the same order of magnitude of that recorded during a typical sunny summer day. These results further confirm how the low thermal conductivity of the soil limits the propagation of the heat wave in depth, with thermal increases not so significant as to distort the geotechnical characteristics of the soil (except for a very pellicular portion close to the soil-air interface). Clearly, these results derive from the simulation performed on a soil different from that of the burned areas, in which the component of organic litter and duff can be present in a much greater amount in the first horizons. In this case, the temperatures recorded in the subsoil following the combustion of these substances could be much higher. The literature data (DeBano, 2000; Neary and DeBano, 2008; Neary and Leonard, 2020), however, agree that it is unlikely that below the first 10 cm of depth the effects of the temperature rise can have significant effects on the physical-mechanical characteristics of the soil.
Furthermore, results from numerical simulations show a satisfactory agreement with the experimental data, correctly reproducing the shape of the measured curves, especially for the first 200 min of simulation (Figure 11). Despite this, experimental temperatures registered at −2, −4 and −6 cm appears closer one to each other with respect to the simulated temperatures; a possible explanation could lie in the not precise positioning of the thermocouples at the various depths, and in particular for the second and third sensors which would seem to be placed a little more superficially than expected. Despite these problems and the lack of refinement in the treatment of data and simulation results, it can be said that it is possible to model the analyzed phenomena with sufficient accuracy, adapting a software that uses algorithms dedicated to the modeling of heat flows in conditions of significantly lower gradients.
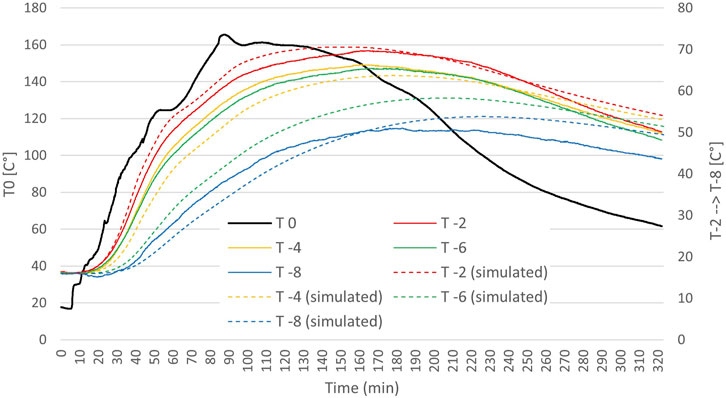
FIGURE 11. Measured vs simulated temperature values over time, for the six thermocouples placed at different depths.
6 Conclusion
According to literature, the thermal soil disturbance following the occurrence of a fire is largely dependent on its intensity, duration, and recurrence, but also on the amount of fuel, and on the hydro-geological and thermo-physical characteristics of the soils. However, the direct measures of temperature which can be reached at surface and in the underground soils during wildfire, are relatively scarce in literature; therefore, most of the statements on this topic are based on a-priori assumption that high temperatures, at least > 100°C, can develop in the ground.
In winter 2017, in NW Italy many wildfires affected a wide area in the Alps. Detailed studied investigated those occurred in the Susa Valley, dealing with characterization of soils, phenomenology of the fire, erosion, and debris flow (the most relevant affected the town of Bussoleno with about 200 people evacuated). In this framework, authors reproduced some controlled fires aimed at reproducing what happens in the underground and at simulating fuel loads like the one observed in the test sites.
The small-scale fire simulations have allowed to verify how the depth affected by significant increases in temperatures, during combustion, is truly pellicular. This is certainly true under experimental conditions; however, it is possible that in situations where the quantity of organic matter present within the first soil horizons is greater, the temperatures developed are considerably higher. Regarding this, future investigations need to be conducted. These results also highlight how the low thermal conductivity of the soil, for those amounts of fuel and duration of fire, limits the propagation of the heat wave in depth, with thermal increases never exceeding 100°C (except for a very pellicular portion close to the soil-air interface). These data, although localized and limited in number, seem to indicate that all those reactions/products expected by many authors in the literature for temperatures above 100°C are not so easy to occur. If confirmed in other situations and locations, we will probably need to re-think the processes that develop due to high temperatures in the subsurface because of fires, and to identify new causes that can account for such large ground effects on slopes after wildfires.
Data availability statement
All relevant data is contained within the article: The original contributions presented in the study are included in the article/Supplementary material, further inquiries can be directed to the corresponding author.
Author contributions
JC: Conceptualization, Data curation, Formal Analysis, Investigation, Methodology, Software, Supervision, Validation, Writing–original draft, Writing–review and editing. GM: Conceptualization, Data curation, Formal Analysis, Funding acquisition, Investigation, Methodology, Supervision, Validation, Writing–original draft, Writing–review and editing. DV: Conceptualization, Data curation, Formal Analysis, Funding acquisition, Investigation, Methodology, Software, Validation, Writing–original draft.
Funding
The author(s) declare financial support was received for the research, authorship, and/or publication of this article. The research was funded by own research funds assigned by the University to researchers and professors.
Acknowledgments
We would like to acknowledge Prof. Matteo Garbarino, Prof. Raffaella Marzano and Dr. Donato Morresi for their support and insight in the field of forestry and fire dynamics. We thank you very much also Dr. Daniele Drago for the involvement in the technical tables and for the precious suggestions.
Conflict of interest
The authors declare that the research was conducted in the absence of any commercial or financial relationships that could be construed as a potential conflict of interest.
Publisher’s note
All claims expressed in this article are solely those of the authors and do not necessarily represent those of their affiliated organizations, or those of the publisher, the editors and the reviewers. Any product that may be evaluated in this article, or claim that may be made by its manufacturer, is not guaranteed or endorsed by the publisher.
References
Agbeshie, A. A., Abugre, S., Atta-Darkwa, T., and Awuah, R. (2022). A review of the effects of forest fire on soil properties. J. For. Res. 33, 1419–1441. doi:10.1007/s11676-022-01475-4
Badia, D., and Marti, C. (2003). Plant ash and heat intensity effects on chemical and physical properties of two contrasting soils. Arid. Land Res. Manag. 17, 23–41. doi:10.1080/15324980301595
Bristow, K. L. (1998). Measurement of thermal properties and water content of unsaturated sandy soil using dual-probe heat pulse probes. Agric. For. Meteorol. 89, 75–84. doi:10.1016/S0168-1923(97)00065-8
Campbell, G. S., Jungbauer, J. D., Bidlake, W. R., and Hungerford, R. D. (1994). Predicting the effect of temperature on soil thermal conductivity. Soil Sci. 158, 307–313. doi:10.1097/00010694-199411000-00001
Cawson, J. C., Nymanb, P., Smith, H. G., Lane, N. J. P., and Sheridan, G. J. (2016). How soil temperatures during prescribed burning affect soil water repellency, infiltration and erosion. Geoderma 278, 12–22. doi:10.1016/j.geoderma.2016.05.002
Certini, G. (2005). Effects of fire on properties of forest soils: a review. Oecologia 143, 1–10. doi:10.1007/s00442-004-1788-8
Chicco, J. M., and Mandrone, G. (2022a). How a sensitive analysis on the coupling geology and borehole heat exchanger characteristics can improve the efficiency and production of shallow geothermal plants. Heliyon 8 (6), e09545. doi:10.1016/j.heliyon.2022.e09545
Chicco, J. M., and Mandrone, G. (2022b). Modelling the energy production of a borehole thermal energy storage (BTES) system. Energies 15 (24), 9587. doi:10.3390/en15249587
Chicco, J. M., Verdoya, M., Giuli, G., and Invernizzi, C. (2019). “Thermophysical properties and mineralogical composition of the Umbria-Marche carbonate Succession (Central Italy),” in The geological society of America. Special paper. 250 million years of Earth history in Central Italy: celebrating 25 Years of the geological observatory of coldigioco 542. Editors C. Koeberl, and D. M. Bice (Boulder, CO, USA: The Geological Society of America). doi:10.1130/2019.2542(02)
Clauser, C., and Huenges, E. (1995). “Thermal conductivity of rocks and minerals,” in Rock physics and phase relations: a handbook of physical constants (Washington, DC, USA: American Geophysical Union), 105–126. doi:10.1029/RF003p0105
DeBano, L. F. (2000). Water repellency in soils: a historical overview. J. Hydrol. 231-232, 4–32. doi:10.1016/S0022-1694(00)00180-3
DeBano, L. F., Neary, D. G., and Ffolliott, P. F. (1998). Fire’s effects on ecosystems. New York, NY, USA: John Wiley & Sons.
Doerr, S. H., Blake, W. H., Shakesby, R. A., Stagnitti, F., Vuurens, S. H., Humphreys, G. S., et al. (2004). Heating effects on water repellency in Australian eucalypt forest soils and their value in estimating wildfire soil temperatures. Int. J. Wildland Fire 13, 157–163. doi:10.1071/WF03051
Doerr, S. H., Shakesby, R. A., and Walsh, R. P. D. (2000). Soil water repellency: its causes, characteristics, and hydro-geomorphological significance. Earth-Sci. Rev. 51, 33–65. doi:10.1016/S0012-8252(00)00011-8
Fréjaville, T., Curt, T., and Carcaillet, C. (2016). Tree cover and seasonal precipitation drive understorey flammability in alpine mountain forests. J. Biogeogr. 43, 1869–1880. doi:10.1111/jbi.12745
García-Llamas, P., Suárez-Seoane, S., Fernández-Guisuraga, J. M., Fernández-García, V., Fernández-Manso, A., Quintano, C., et al. (2019). Evaluation and comparison of Landsat 8, Sentinel-2 and Deimos-1 remote sensing indices for assessing burn severity in Mediterranean fire-prone ecosystems. Int. J. Appl. Earth Observ. Geoinf. 80, 137–144. doi:10.1016/j.jag.2019.04.006
Giordano, N., Chicco, J., Mandrone, G., Verdoya, M., and Wheeler, W. H. (2019). Comparing transient and steady-state methods for the thermal conductivity characterization of a borehole heat exchanger field in Bergen, Norway. Environ. Earth Sci. 78, 460. doi:10.1007/s12665-019-8397-7
Giovannini, G. (1994). “The effect of fire on soil quality,” in Soil erosion as a consequence of forest fires. Editors M. Sala, and J. L. Rubio (Logrono, Spain: Geoforma Ediciones), 5–27.
Giovannini, G., and Lucchesi, S. (1983). Effect of fire on hydrophobic and cementing substances of soil aggregates. Soil Sci. 136, 231–236. doi:10.1097/00010694-198310000-00006
Giovannini, G., Lucchesi, S., and Giachetti, M. (1988). Effects of heating on some physical and chemical parameters related to soil aggregation and erodibility. Soil Sci. 146, 255–261. doi:10.1097/00010694-198810000-00006
Girardin, M. P., and Terrier, A. (2015). Mitigating risks of future wildfires by management of the forest composition: an analysis of the offsetting potential through boreal Canada. Clim. Change 130, 587–601. doi:10.1007/s10584-015-1373-7
Guerrero, C., Mataix-Solera, J., Garcia-Orenes, F., Gomez, I., and Navarro-Pedreno, J. (2001). Different patterns of aggregate stability in burned and restored soils. Arid. Land Resour. Manag. 15, 163–171. doi:10.1080/15324980151062823
Hubbert, K. R., Wohlgemuth, P. M., Beyers, J. L., and Gerrard, R. (2012). Post-fire soil water repellency, hydrologic response, and sediment yield compared between grass-converted and chaparral watersheds. Fire Ecol. 8, 143–162. doi:10.4996/fireecology.0802143
Huetter, E. S., Koemle, N. I., Kargl, G., and Kaufmann, E. (2008). Determination of the effective thermal conductivity of granular materials under varying pressure conditions. J. Geophys. Res. 113, E12004. doi:10.1029/2008JE003085
Kluitenberg, G. J., Ham, J. M., and Bristow, K. L. (1993). Error analysis of the heat pulse method for measuring soil volumetric heat capacity. Soil Sci. Soc. Am. J. 57 (6), 1444–1451. doi:10.2136/sssaj1993.03615995005700060008x
Mandrone, G., Vacha, D., and Chicco, J. (2023). Post-wildfire erosion rates and triggering of debris flows: a case study in Susa Valley (Bussoleno). E3S Web Conf. 415, 04009. doi:10.1051/e3sconf/202341504009
Marquardt, D. W. (1963). An algorithm for least-squares estimation of nonlinear parameters. J. Soc. Indust. Appl. Math. 11, 431–441. doi:10.1137/0111030
Mataix-Solera, J., Cerdà, A., Arcenegui, V., Jordán, A., and Zavala, L. M. (2011). Fire effects on soil aggregation: a review. Earth-Sci. Rev. 109, 44–60. doi:10.1016/j.earscirev.2011.08.002
Mataix-Solera, J., and Doerr, S. H. (2004). Hydrophobicity and aggregate stability in calcareous topsoils from fire-affected pine forests in south-eastern Spain. Geoderma 118, 77–88. doi:10.1016/S0016-7061(03)00185-X
Midttømme, K., and Roaldset, E. (1998). The effect of grain size on thermal conductivity of quartz sands and silts. Petrol. Geosci. 4, 165–172. doi:10.1144/petgeo.4.2.165
Neary, D. G., Klopatek, C. C., DeBano, L. F., and Ffolliott, P. F. (1999). Fire effects on belowground sustainability: a review and synthesis. For. Ecol. Manag. 22, 51–71. doi:10.1016/S0378-1127(99)00032-8
Neary, D. G., and Leonard, J. M. M. (2020). “Effects of fire on grassland soils and water: a review,” in Grasses and grassland aspects (London, UK: IntechOpen). doi:10.5772/intechopen.90747
Neary, D. G., Ryan, K. C., and DeBano, L. F. (2008). “Wildland fire in ecosystems: effects of fire on soil and water,”. U.S. General Technical Report RMRS-GTR-42 4 (Ogden, UT, USA: U. S. Department of Agriculture, Forest Service, Rocky Mountain Research Station). doi:10.2737/RMRS-GTR-42-V4
Parise, M., and Cannon, S. H. (2012). Wildfire impacts on the processes that generate debris flows in burned watersheds. Nat. Hazards 61, 217–227. doi:10.1007/s11069-011-9769-9
Pezzatti, G. B., Zumbrunnen, T., Bürgi, M., Ambrosetti, P., and Conedera, M. (2013). Fire regime shifts as a consequence of fire policy and socio-economic development: an analysis based on the change point approach. For. Policy Econ. 29, 7–18. doi:10.1016/j.forpol.2011.07.002
Rengers, F. K., McGuire, L. A., Oakley, N. S., Kean, J. W., Staley, D. M., and Tang, H. (2020). Landslides after wildfire: initiation, magnitude, and mobility. Landslides 17, 2631–2641. doi:10.1007/s10346-020-01506-3
Ryan, K., and Noste, N. (1985). “Evaluating prescribed fires,”. General Technical Report INT (Fort Collins, CO, USA: USDA Forest Service Intermountain Forest and Range Experiement Station), 230–238.182,
Ryan, K. C. (2002). Dynamic interactions between forest structure and fire behavior in boreal ecosystems. Silva Fenn. 36, 13–39. doi:10.14214/sf.548
Shakesby, R. A., and Doerr, S. H. (2006). Wildfire as a hydrological and geomorphological agent. Earth Sci. Rev. 74, 269–307. doi:10.1016/j.earscirev.2005.10.006
Shakesby, R. A., Wallbrink, P. J., Doerr, S. H., English, P. M., Chafer, C., Humphreys, G. S., et al. (2007). Distinctiveness of wildfire effects on soil erosion in south-east Australian eucalypt forests assessed in a global context. For. Ecol. Manag. 238 (1-3), 347–364. doi:10.1016/j.foreco.2006.10.029
Sobota, T. (2014). “Fourier’s Law of heat conduction,” in Encyclopedia of thermal stresses. Editor R. B. Hetnarski (Cham, Switzerland: Springer), 1769–1778. doi:10.1007/978-94-007-2739-7_384
Stoof, C. R., Moore, D., Ritsema, C. J., and Dekker, L. W. (2011). Natural and fire-induced soil water repellency in a Portuguese shrubland. Soil Sci. Soc. Am. J. 75 (6), 2283–2295. doi:10.2136/sssaj2011.0046
Tecle, A., and Neary, D. (2015). Water quality impacts of forest fires. J. Pollut. Eff. Cont. 3 (2), 1–7. doi:10.4172/2375-4397.1000140
Úbeda, X., and Outeiro, L. (2009). “Physical and chemical effects of fire on soil,” in Fire effects on soils and restoration strategies. Editors A. Cerdà, and P. R. Robichaud (Enfield, NH, USA: Science Publishers), 105–133. doi:10.1201/9781439843338-c4
Vacha, D., Mandrone, G., Garbarino, M., and Morresi, D. (2021). “First consideration about post 2017 wildfire erosion response and debris flow in Susa Valley (NW Italy),” in Understanding and reducing landslide disaster risk. WLF 2020. ICL contribution to landslide disaster risk reduction. Editors B. Tiwari, K. Sassa, P. T. Bobrowsky, and K. Takara (Cham, Switzerland: Springer), 443–450. doi:10.1007/978-3-030-60706-7_47
Vacha, D., Mandrone, G., Morresi, D., and Garbarino, M. (2023). “Mapping post-fire monthly erosion rates at the catchment scale using empirical models implemented in gis. A case study in northern Italy,” in Progress in landslide research and technology. K. Sassa, K. Konagai, B. Tiwari, Ž. Arbanas, and S. Sassa (Cham, Switzerland: Springer), 1. doi:10.1007/978-3-031-16898-7_6
Vadilonga, T., Úbeda, X., Germann, P. F., and Lorca, M. (2008). Effects of prescribed burnings on soil hydrological parameters. Hydrol. Process. 22, 4249–4256. doi:10.1002/hyp.7032
Keywords: wildfire, soil, temperature, thermal conductivity, field studies, numerical modelling, debris flow
Citation: Chicco JM, Mandrone G and Vacha D (2023) Effects of wildfire on soils: field studies and modelling on induced underground temperature variations. Front. Earth Sci. 11:1307569. doi: 10.3389/feart.2023.1307569
Received: 04 October 2023; Accepted: 04 December 2023;
Published: 15 December 2023.
Edited by:
Gian Marco Marmoni, Sapienza University of Rome, ItalyReviewed by:
António Vieira, University of Minho, PortugalLorenzo Borselli, Instituto de geologia—Universidad Autonoma de San Luis Potosi, Mexico
Copyright © 2023 Chicco, Mandrone and Vacha. This is an open-access article distributed under the terms of the Creative Commons Attribution License (CC BY). The use, distribution or reproduction in other forums is permitted, provided the original author(s) and the copyright owner(s) are credited and that the original publication in this journal is cited, in accordance with accepted academic practice. No use, distribution or reproduction is permitted which does not comply with these terms.
*Correspondence: Jessica Maria Chicco, jessica.chicco@unito.it