- 1Faculty of Engineering, Bioengineering Department, Recep Tayyip Erdogan University, Rize, Turkey
- 2Complex Carbohydrate Research Center, Athens, GA, United States
- 3Jiangsu Co-innovation Center of Efficient Processing and Utilization of Forest Resources, Nanjing Forestry University, Nanjing, China
- 4College of Chemical Engineering, Nanjing Forestry University, Nanjing, China
- 5Jiangsu Province Key Laboratory of Green Biomass-Based Fuels and Chemicals, Nanjing, China
- 6Mascoma LLC, Lebanon, PA, United States
- 7Chemical Engineering and Material Science, Great Lakes Bioenergy Center, Michigan State University, East Lansing, MI, United States
- 8Engineering Technology Department, Biotechnology Program, College of Technology, University of Houston, Houston, TX, United States
Lignocellulosic biomass is highly recalcitrant and requires a pretreatment step to improve the enzyme accessibility and fermentable sugar yields during enzymatic hydrolysis. Our previous studies demonstrated the rearrangement of the hydrogen bond network within CIII, makes it “amorphous-like” and facilitates easier glucan chain extraction by enzyme. Also, these changes increase the number of solvent-exposed glucan chain hydrogen bonds with water ~50% lowering the surface-bound cellulase by 60–70%. Also, major chemical modifications to lignin occur via ammonolysis of ester-linked ferulate and coumarate linkage. These apparent ultrastructural changes help the enhancement of cellulase activity resulting in higher production of fermentable sugars during enzyme hydrolysis of EA pretreated corn stover relative to Ammonia Fiber Expansion (AFEX) pretreatment. To understand ultra-structural modifications that occur during EA pretreatment, Scanning Electron Microscopy (SEM) and Transmission Electron Microscopy (TEM) were used to examine untreated and EA-pretreated corn stover in an effort to visualize changes in the biomass resulting from the pretreatment. In addition, Immunofluorescence Microscopy was applied to both untreated and pretreated plant cell walls using glycan-directed monoclonal antibodies to reveal possible changes in the spatial distributions of wall glycan epitopes resulting from EA pretreatment. This evaluation was complemented with glycome profiling to determine the glycan epitope compositions of EA-pretreated cell walls relative to untreated and AFEX pretreated corn stover, where lignin and carbohydrates are not extracted. Distinct differences could be observed in the case of xyloglucan, unsubstituted and substituted pectin- and pectic-arabinogalactan-epitope levels in the plant cell wall after EA pretreatment compared with untreated and AFEX-pretreated walls. Liquid ammonia induced de-lignification of cell wall has helped to clearly identify the glucans that are intact after pretreatment. These studies support some of our hypothesis that liquid ammonia cleaves lignin–carbohydrate ester linkages, partially solubilizing lignin and its associated carbohydrates. Specifically, the imaging studies clearly show that some of the cell wall components are extracted as a separate liquid stream during the EA process, thereby creating porous, interconnected tunnel-like networks in the plant cell walls thereby providing better access of carbohydrate polymers to enzymes and thereby increasing the sugar yield from the EA-pretreated biomass.
Introduction
Lignocellulosic biomass is the most abundant source of carbohydrates to produce liquid renewable fuels via fermentation. Interest in this field has been increased markedly in the past two decades due to high fluctuation of petroleum prices and climate change concerns (Sarks et al., 2017). Overcoming biomass recalcitrance is a key factor for reducing the price of lignocellulosic-based fuels and chemicals so that they become more competitive with petroleum products (Himmel et al., 2007; da Costa Sousa et al., 2009; Kumar and Sani, 2018; Kim et al., 2019). Various physical, chemical, physicochemical, and biological pretreatment methods have been tested on different biomasses in order to improve enzymatic hydrolysis yields, with varying degrees of success. However, developing an economically viable pretreatment process is one of the most important challenges to overcome for a commercial lignocellulosic biorefinery (Mosier et al., 2005).
Ammonia Fiber Expansion (AFEX), is an ammonia-based pretreatment process that has shown tremendous promise to cost-effectively reduce the recalcitrance of lignocellulosic biomass to enzymatically catalyzed deconstruction into fermentable sugars (Gao et al., 2014). Unlike other aqueous pretreatments, AFEX is a dry-to-dry process that uses gaseous ammonia and reduces plant cell-wall recalcitrance through a unique physicochemical mechanism (Mielenz, 2009; Campbell et al., 2013; Chundawat et al., 2013). The volatile nature of ammonia helps in its 97% recovery and reuse for subsequent cycles and helps downstream enzyme and microbial fermentation. In a previous study, 249 and 256 kg Mg−1 ethanol yields were obtained from AFEX-pretreated sugarcane bagasse and cane leaf matter after high solids loading (18%) enzymatic hydrolysis and fermentation (Mokomele et al., 2018a). For the same biomass samples, steam explosion-pretreatment resulted in substantially lower ethanol yields that ranged between 162 and 203 kg Mg−1 (Mokomele et al., 2018b). AFEX pretreatment drastically changes the physical properties of the pretreated material with respect to specific surface area, pore volume, cellulose crystallinity index, degree of polymerization, lignin content in biomass, reduced acetyl content in biomass, among other properties (Balan, 2014). High severity pretreatments (high temperature and higher ammonia to biomass ratio) increase the rate of enzyme hydrolysis and significantly decrease the amount of enzymes needed to convert the biomass into sugars that are subsequently utilized by microorganisms to produce fuels and chemicals (Gao et al., 2014). Previously, a comprehensive glycome profiling study was done on untreated and AFEX pretreated plant biomass from diverse phylogenetic classes monitoring the fate of most major non-cellulosic cell wall glycan epitopes as affected by AFEX pretreatment regimes (Pattathil et al., 2015). This study demonstrated that AFEX pretreatment regimes induce an overall loosening effect of non-cellulosic cell wall components (xylan, xylo-glucan, arabino-xylan, arabino-galactan, and pectin) of the monocot plant biomass, as indicated by the increased extractability of non-cellulosic glycans including pectin and hemicellulose components, without destroying most of the detectable wall glycan epitopes. Also, dicot hardwoods and softwoods biomass have different hemicellulose composition and reduced arabinoxylan ester linkages, which also reduces impacts the action of the ammonia pretreatment (Balan et al., 2009b). These non-cellulosic cell wall components get hydrolyzed by commercial hemicellulose and pectinase enzymes to produce significant amount of oligomeric (accumulate to the extent of about 18–25% of the total soluble sugars in the hydrolysate) in addition to producing monomeric sugars (Gunawan et al., 2017). Low degree of polymerization (DP) oligosaccharides were inhibitory than high DP oligosaccharides to commercial enzymes (Ctec2, Htec2, and Multifect pectinase) and the addition of monomeric sugars would further contribute to the inhibitory effect (Xue et al., 2015).
Recently, Extractive Ammonia (EA) pretreatment was developed that aids the selective extraction of lignin via ammonolysis of ester-linked ferulate and coumarate linkages leaving 95% of the carbohydrates in the ammonia-insoluble phase (da Costa Sousa et al., 2016a). Depending on the processing conditions, EA can extract up to 45% of the lignin present in corn stover, which can potentially be used for conversion to fuels and chemicals. Details characterization of various lignin-rich streams generated during EA processing of corn stover has been reported before (da Costa Sousa et al., 2016b). Lignin removal by EA changes the composition of biomass to higher cellulose and hemicellulose content and also helps improving enzymatic hydrolysis yields by 6% due to reduced enzyme inhibition due to lignin (da Costa Sousa et al., 2016a). In addition to extracting lignin, EA uses anhydrous liquid ammonia to improve the biomass conversion to fuels and chemicals by 27% (da Costa Sousa et al., 2016a; Jin et al., 2016) when compared to AFEX due to conversion of native CI allomorph to CIII. Due to re-organization of hydrogen bonding next work in CIII, the hydroxyl residues are oriented above the crystal lattice there-by make it more hydrophilic (miscible in water). This modified cellulose crystal allows doubling of enzymatic hydrolysis rates due to more efficient enzyme adsorption-desorption onto the substrate (60–70% lower maximum surface-bound cellulase capacity) reducing non-productive enzyme binding, and thereby resulting in significant enzyme cost savings during biomass conversion to fuels and chemicals (Chundawat et al., 2011a; Gao et al., 2013). These findings clearly show the change in ultrastructure of corn stover contribute to the enhancement of enzymatic hydrolysis. The ammonia-insoluble lignin that remains intertwined with the structural carbohydrates of the plant cell wall can be recovered as un-hydrolyzed solids (UHS) after enzymatic hydrolysis of the carbohydrates (da Costa Sousa et al., 2016a).
Currently, a powerful tool that has proven useful for that purpose is glycome profiling, which uses a large and diverse library of antibodies that recognize non-cellulosic plant cell wall glycans to monitor glycan epitope composition and extractability in plant biomass samples (Pattathil et al., 2010, 2012). These antibodies can also be used for immunohistochemistry which can localize plant glycan in plant biomass samples (Avci et al., 2012). Both techniques have been used successfully to evaluate the presence and relative abundance of non-cellulosic glycans during pretreatment, microbial fermentation, and genetic manipulations of plant biomass (DeMartini et al., 2011; Ratnaparkhe et al., 2013; Shen et al., 2013; Izquierdo et al., 2014; Li et al., 2014; Pattathil et al., 2015; Gunawan et al., 2017). The information obtained from these studies has helped significantly to better understand why certain pretreatment conditions result in better sugar conversion and thereby overcoming the recalcitrant nature of plant biomass.
In this study, we have applied glycome profiling and immunohistochemistry to better understand how EA pretreatment modifies the ultrastructure and glycan composition of corn stover cell walls, the glycan epitope composition, extractability and localization were determined for corn stover cell walls before and after AFEX and EA pretreatment. In addition, ultrastructural changes in AFEX- and EA-pretreated corn stover cell walls were analyzed using Scanning Electron Microscopy (SEM), Transmission Electron Microscopy (TEM), and powder X-ray diffraction (XRD) techniques. These studies allowed, for the first time, the identification of the glycans that are not affected by EA pretreatment and those that are cleaved and extracted during the process.
Materials and Methods
Biomass Source
Corn stover was harvested in September 2008, was obtained from the Michigan State University (MSU) research plot (East Lansing, MI, USA). The corn hybrid used in this study was NK 49-E3 (Syngenta, Basel, Switzerland), which is a typical corn stover hybrid used in the Great Lakes region. We refer to it here as MSU corn stover. The biomass was milled to a 40 mesh size, dried at 50°C to bring the moisture content down to 8%, vacuum sealed in polythene bags and refrigerated at 4°C until further use. Composition of biomass did not change even after 10 years of storage. For imaging work, fresh whole corn plants were harvested and used. Corn stems were sectioned using a razor blade into thin slices (slice thickness ranging from 100 to 1 mm) and air-dried (to the desired moisture level) in a clean hood prior to AFEX treatment. For high solids loading enzymatic hydrolysis experiments after subjecting to AFEX or EA pretreatment, we used pre-milled corn stover obtained from Great Lakes Bioenergy Center (GLBRC) that passed through a 10 mm. The corn (Pioneer 36H56) from which the stover was produced was planted in May of 2009 in field 570-N at the Arlington Agricultural Research Station in Columbia Country, WI and harvested in November of 2009 and stored in zip-lock bags with 8% moisture content at room temperature.
AFEX Pre-treatment
The corn stover was pretreated using AFEX process as previously described (Balan et al., 2009a) using a high pressure 5-gallon stainless steel PARR reactor under the following conditions: 1:1 (w/w) ammonia to biomass ratio, 60% moisture, 100°C, and 30 min reaction time in a walk-in hood. After the AFEX process was completed the ammonia was released from the reactor and pretreated samples were transferred to a plastic tray and kept in a hood overnight to remove residual ammonia from the biomass. Crystalline CIII samples were prepared using cotton linter derived cellulose (Sigma, St. Louis, MO) in the presence of liquid ammonia based on a previously published protocol using an HEL high pressure reactor system comprised of a 500 ml stainless steel reactor with a motorized auger to mix the cellulose during pretreatment. CIII was produced using the following conditions: 6:1 (w/w) ammonia to biomass ratio, 10% moisture, 100°C, 30 min reaction time (Chundawat et al., 2011b). Details about the pretreatment conditions and the compositions of different samples used in this work are listed in Tables 1, 2.
EA Pre-treatment
Corn stover was pretreated using EA using a previously published protocol (Chundawat et al., 2011b). The pretreatment conditions were 10% moisture, 6:1 (w/w) ammonia-to-biomass ratio for 30 min at 120°C in a high-pressure stainless steel reactor vessel. EA pretreatment removed 16 wt% of the biomass or about 44 wt% of the total lignin present in the biomass. The EA treated samples was transferred to a plastic tray and kept in a fume hood to remove residual ammonia from the biomass. The composition of the EA treated corn stover was found to be similar to previously published results (da Costa Sousa et al., 2017).
In one another experiment to evaluate the effect of lignin removal from corn stover on sugar conversion, we varied the pretreatment conditions (temperature, ammonia-to-biomass ratio (NH3:BM), and residence time) and evaluated glucan conversion after 24 h. We used Box-Behnken experimental design using Minitab software (Minitab Inc., State College, PA, USA). Since CIII conversion was observed only beyond 2.5:1 ammonia-to-biomass loading, we chose 3:1 The high and low values for temperature (25 and 115°C), NH3:BM ratio (3:1 and 6:1) and residence time of (5 and 30 min.) were used, respectively. We plotted the % lignin removal from different EA pretreated corn stover against glucan conversion at high solids loading (18%) using the protocol given below.
X-Ray Diffraction Method
Crystallinity index (CrI) was measured using the Rigaku Rotaflex 200B X-ray diffractometer. The sample was placed vertically and analyzed using the horizontal goniometer. The sample was scanned at 1°/min from 2θ = 10° to 26° with a step size of 0.5° (Gollapalli et al., 2002).
Transmission Electron Microscopy (TEM)
Ultrathin sections (80 nm) of corn stover stems from the blocks that were prepared for immunolabeling were taken with a Leica EM UC6 microtome (Leica Microsystems, Wetzlar, Germany), and stained with 2% (w/v) uranyl acetate for 5 min and 1% (w/v) potassium permanganate (KMnO4) for 10 min. Sections were observed under a Zeiss 902A transmission electron microscope operated at 80 kV.
Scanning Electron Microscopy (SEM)
SEM was carried out on a JEOL (Japan Electron Optics Laboratories) 6400V SEM with a LaB6 emitter. Biomass samples were gold sputter coated without critical point drying (CPD), to avoid possible extraction of AFEX surface deposits during CPD, and imaged at accelerating voltages between 10 and 20 kV to prevent electron beam induced damage to the specimens. Cell wall lumen perimeter and enclosed area were calculated using Zeiss LSM Image Browser (Version 3.0, Carl Zeiss International, Jena, Germany).
High Solid Loading Enzymatic Hydrolysis
The pretreated corn stover samples were hydrolyzed at 6% (w/w) glucan loading in a fermenter equipped with a pitched blade impeller. Hydrolysis was performed over a period of 3 days with 20 mg protein/g glucan enzyme loading at 50°C and 1,000 rpm. Enzymatic hydrolysis was performed using a commercial enzyme mixture including Cellic® Ctec2 10 mg protein/ g glucan (in pretreated biomass), Htec2 (Novozymes, Franklinton, NC), 5 mg protein/g glucan and Multifect Pectinase (Genencor Inc, USA), 5 mg protein/g glucan. Samples were taken every 24 h. The hydrolysate was harvested by centrifugation at 6,000 rpm for 30 min and then 14,000 rpm for 30 min to remove UHS. Residual sugars bound to UHS was washed with 10 volume of water, mixed using glass rod, centrifuged, and supernatant collected separately. This process was repeated three time to ensure almost all the residual sugars bound to UHS are removed. Both the hydrolysate and water washed supernatant samples were analyzed for their sugar content and combined to estimate the high solids loading hydrolysis sugar conversion. The residual sugars bound to un-hydrolyzed solids did contribute to the overall enzymatic hydrolysis yield. Hydrolysate and sugars recovered from UHS were then sterile filtered through a 0.22-um filter cup and stored at −20°C before subjecting to HPLC analysis using Bio-Rad Aminex HPX-87P column. Samples obtained from compositional analysis (Hames et al., 2008) were subjected to HPLC using Bio-Rad Aminex HPX-87H column to determine sugar concentrations against standards.
Monoclonal Antibodies (mAbs)
mAbs recognizing epitopes on diverse plant cell wall glycans were obtained as hybridoma cell culture supernatants from stocks at the Complex Carbohydrate Research Center (CCRC). Antibodies used in this study are available from CarboSource (http://www.carbosource.net). LAMP and BG-1 antibodies are available from Bio-supplies (Parkville, Victoria, Australia; http://www.biosupplies.com.au).
Glycome Profiling
Glycome profiling of untreated, AFEX, and EA pretreated corn stover samples was performed as described before (Pattathil et al., 2012). Briefly, Alcohol Insoluble Residue (AIR) cell wall materials were prepared from MSU corn stover and were subjected to sequential extractions with ammonium oxalate (50 mM), sodium carbonate (50 mM), KOH (1 and 4 M) and acidic chlorite. The extracts were then subjected to ELISAs against a suite of cell wall glycan-directed mAbs and the results reported as heat maps. The amounts of cell wall materials recovered during each extraction are depicted as bar graphs above the respective heat map panels.
Immunolabeling
Untreated and EA pretreated corn stover stems were fixed and processed for immunolabeling as described in detail previously (Avci et al., 2012). In brief, semi-thin sections (250 nm) were taken using a Leica EM UC6 microtome (Leica Microsystems, Wetzlar, Germany) and mounted on glass slides (color frost/plus, Fisher Scientific, Pittsburgh, PA, USA). Sections were blocked for 30 min with 3% (w/v) non-fat dry milk in 10 mM potassium phosphate, pH 7.1, containing 0.5 M NaCl (KPBS), and then were washed for 5 min. in KPBS. Undiluted hybridoma supernatants containing the monoclonal antibodies were applied to the sections for 60 min. Sections were then washed three times for 5 min. with KPBS. Goat anti-mouse conjugated to Alexa-fluor 488 (Invitrogen, Carlsbad, CA, USA) diluted 1:100 in KPBS was applied as a secondary antibody for 60 min. Sections were then washed for 5 min. with KPBS, and then for 5 min. with distilled water. CITIFLUOR antifadent medium AF1 (Electron Microscopy Sciences, Hatfield, PA, USA) was applied along with a cover slip. Samples were observed under an Eclipse 80i epifluorescence microscope equipped with a Nikon DS-Ri1 camera and NIS Elements Basic Research software (Nikon, Melville, NY, USA). Images were assembled using Adobe Photoshop (Adobe Systems, San Jose, CA, USA).
Results and Discussion
Understanding Cell Wall Ultrastructure Using X-Ray Diffraction, Sem, Tem
Pretreatment setup is given for AFEX pretreatment, EA pretreatment (Figures 1A,B). We found that high moisture content and/or low ammonia-to-biomass ration does not lead to CIII formation (da Costa Sousa et al., 2016a). In order to understand changes in cell wall structure resulting from EA pretreatment of corn stover, powder X-Ray Diffraction (pXRD), Scanning Electron Microscopy (SEM), and Transmission Electron Microscopy (TEM), were applied to untreated, AFEX-pretreated, and EA-pretreated corn stover biomass. Data from X-Ray diffraction studies show that the CIII allomorph is produced from the cellulose-ammonia complex during EA pretreatment (Figure 2). Our previous study demonstrated that enzymatic hydrolysis rates are improved 2- to 5-fold by restructuring the CI allomorph to CIII (Chundawat et al., 2011a; Gao et al., 2013). When EA pretreatment was applied to corn stover, 16 wt% of the biomass was extracted by ammonia (Table 2) based on the conditions showed in Table 1. The ammonia-soluble fraction contained 44 wt% of the total lignin, while almost all the carbohydrates remain available in the residual dry solids for subsequent processing. In addition, the pretreated corn stover cell walls exhibit significant morphological differences with respect to the untreated corn stover (UT-CS) (Chundawat et al., 2011b). In the SEM images (Figure 3), corn stover samples obtained from the stem region looked darker in color and distorted after the pretreatment compared to the untreated sample. Besides, high resolution TEM was also conducted to reveal the effects of the EA pretreatment (Figure 4). The images revealed delamination and kinking of primary cell walls after EA pretreatment (Figure 4). Plant cell walls became loose and more porous after the pretreatment. There were no severe structural changes in the secondary cell walls indicating these walls are more resistant to the pretreatment conditions. Generally, alkali pretreatments work better on herbaceous plants due to the presence of more susceptible arabinoxylan linkages and lesser amount of lignin present when compared to woody biomass (Wyman et al., 2005). Lignin re-localization after the AFEX pretreatment is due to solubilization of lignin during pretreatment followed by deposition on biomass surfaces when ammonia gets released from the reaction vessel (Donohoe et al., 2011). We used potassium permanganate that selectively stains lignin for clear visualization. We observed coalesced dark stained structures with irregular shapes in delamination zones (see arrows in Figure 4D). Similar lignin coalescence was also observed in maize cell walls after dilute acid pretreatment (Donohoe et al., 2008). A similar result was also observed during lignin staining between extractive ammonia treated corn stover (EA-CS) in this work. The pretreated cell walls exhibit an overall lower contrast to safranin staining due to reduced lignin content when compared to UT-CS (Figure 5I). For EA-pretreated cells walls, the staining intensity is reduced and is more uniform across the different cell types, whereas for UT-CS, the intensity of safranin staining is greater in the vascular bundles due to high lignin content. This result indicates that cellulose fibers have been exposed following EA pretreatment, likely due to cell wall delignification, delamination, and swelling. Although, both EA and AFEX pretreatments produce similar changes in overall cell wall morphology, EA also removes lignin and other decomposition products from the biomass while simultaneously producing CIII. These differences are crucial to the improved biological conversion of pretreated corn stover to fermentable sugars. According to the result showed in Figure 1C, the glucan conversion for EA-CS (88%), when compared to UT-CS (25%) and AFEX-CS (61%).
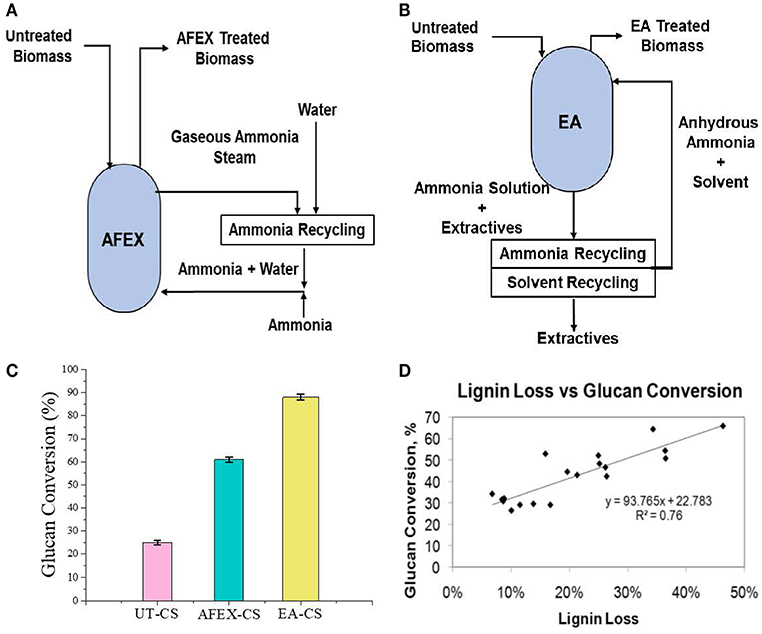
Figure 1. Process flow diagram showing Extractive Ammonia (EA) and ammonia fiber expansion (AFEX) pretreatment and sugar conversion. Here, (A) reactor diagram of AFEX pretreatment, (B) reactor diagram of EA pretreatment, (C) glucan conversion of AFEX/EA pretreated CS, and (D) effect of sugar conversion as a function of lignin removal.
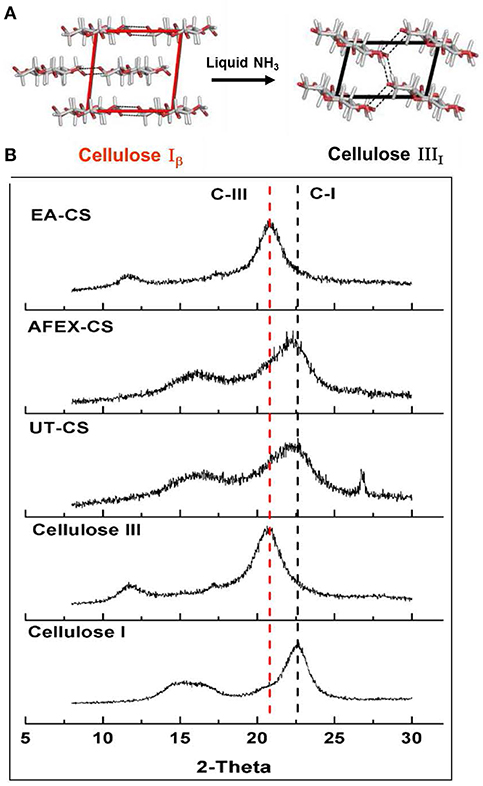
Figure 2. Structure change of cellulose after ammonia treatment. Here, (A) represent glucose gain packing in two different allomorphs of cellulose I (CI) and cellulose III (CIII) and (B) powder X-ray diffraction of CI, CIII, untreated corn stover (UT-CS), AFEX treated corn stover (AFEX-CS) and Extractive ammonia treated corn stover (EA-CS). Crystallinity of cellulose changes from CI to CIII in the case of EA-CS, while, AFEX-CS still have CI similar to UT-CS.
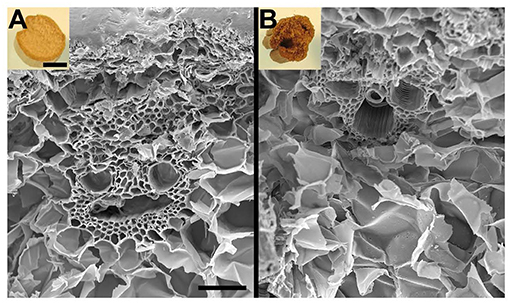
Figure 3. Pictures of untreated (A) and EA pretreated (B) of corn stover stems along with their scanning electron microscopy (SEM) images. SEM revealed no perceptible modification of cell walls, except distorted cell structures were observed after pretreatment. Bar = 1 cm inlets and 100 μm for SEM images.
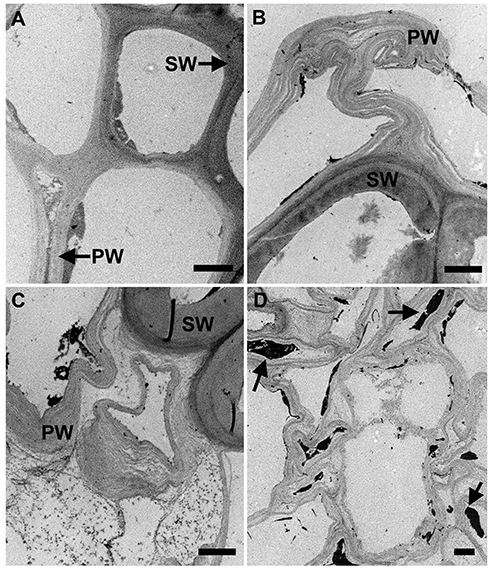
Figure 4. Transmission electron microscopy images of untreated (A) and EA pretreated (B–D) of corn stover stems images. TEM images showed that primary walls (PW) were severely affected after pretreatment. Secondary walls (SW) remained more resistant to EA pretreatment. Delamination and kinking in the primary walls were observed. Cell walls lost their integrity and empty spaces can be seen in the wall. Bar = 2 μm in all images. Sections were post-stained with uranyl acetate, and potassium permanganate (selectively stains lignin).
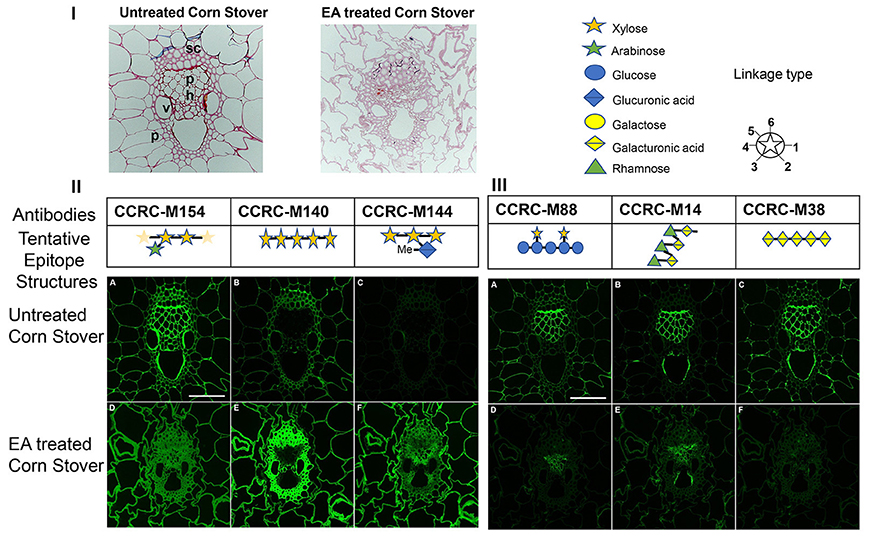
Figure 5. Imaging studies on untreated and ammonia treated corn stover cell walls. Here, (I) lignin staining indicates changes in the wall. Safranin stains lignin that is more concentrated in the vascular bundle in the untreated corn stover samples compared to more uniform distribution and less staining after EA pretreated samples. Parenchyma (p), phloem (ph), schlerenchyma (sc), vessel (v); (II) immunofluorescent labeling of untreated (A, B, C) and EA pretreated (D, E, F) of corn stover stems images by using xylan-directed antibodies. CCRC-M154 (in A and D) recognizes arobinoxylans. CCRC-M140 (in B and E) recognizes long stretches (DP >4) of xylan backbone. CCRC-M144 (in C and F) recognizes 4-O-methyl glucoronoxylans. Bar in A equals to 100 micron and applies to all images; and (III) immunofluorescent labeling of untreated (A, B, C) and EA pretreated (D, E, F) of corn stover stems images by using xyloglucan and pectin-directed antibodies. CCRC-M88 (in A and D) recognizes non-fucosylated xyloglucans. CCRC-M14 (in B and E) recognizes RG-I backbone. CCRC-M38 (in C and F) recognizes HG backbone. Bar in A equals to 100 micron and applies to all images.
Varying EA pretreatment conditions (temperature, NH3:BM, and residence time) resulted in different amount of lignin removal from corn stover and different amount of CIII formation [Unreported results]. In general we found that higher the pretreatment conditions higher the lignin solubilization and removal after EA pretreatment. A full quadratic response surface analysis using regression equations were used to predict the responses of the various effects as a function of the pretreatment conditions within the boundaries set by the experimental design and reported in our previous manuscript (da Costa Sousa et al., 2016a). For this work, we plotted the effect of varying lignin removal on the EA pretreatment and its effect on glucan conversion to demonstrate the recalcitrant nature of lignin and its role in enzyme inhibition (Figure 1D). We found a reasonable correlation (R2 = 0.76) between lignin removal and sugar conversion. In other words, higher the lignin removal higher the glucan conversion was observed.
Understanding Cell Wall Ultrastructure Using Glycome Profiling
Glycome Profiling is a moderate to high-throughput method for obtaining a comprehensive picture of the glycan epitope composition of a cell wall/biomass sample and some information about how tightly those epitopes are bound into the wall (Pattathil et al., 2012). In this study, glycome profiling on untreated and pretreated corn stover samples were conducted in order to understand the fate of most major non-cellulosic cell wall glycans when subjected to pretreatment conditions. Also, through these analyses, we wanted to compare and correlate the changes in composition and extractabilities of non-cellulosic glycan epitopes among AFEX and EA pretreated samples. Supplemental Figure S1 shows the data of the overall glycome profiles on untreated, AFEX and EA pretreated samples. In order to systematically understand those results, we split the entire glycome profiling data into four parts pertaining to, (i) xyloglucans, (ii) unsubstituted/substituted xylans, (iii) pectic-backbone, and (iv) pectic-arabinogalactan components. The following sections will discuss those four parts individually.
Xyloglucans profiles are depicted in Figure 6A for untreated, AFEX and EA pretreated samples. As expected, the least strong extracting reagents (oxalate and carbonate) shown in Panels 1–2 of Figure 6A did not remove xyloglucan epitopes in any of the samples. However, all the strong alkaline extracts (1M KOH, 4M KOH, and 4M KOH post chlorite) shown in Panels 3–6 (Figure 6A) contained abundant fucosylated and non-fucosylated xyloglucan epitopes. Most interestingly, the 1M KOH extract from the untreated sample did not contain any xyloglucan epitopes, while for both AFEX and EA pretreated samples, the non-fucosylated and fucosylated xyloglucan epitopes were extracted. It is to be noted that overall amounts of carbohydrate materials recovered were significantly lower on AFEX and EA pretreated samples. This may be due to the removal of some carbohydrate components as a result of pretreatment and deposited on the surface in the case of AFEX and removed as extractive in the case of EA. However, it is interesting to understand that both AFEX and EA pretreatment regimes induce essentially similar effects on the biomass by loosening xyloglucan components from the cell walls which are otherwise tightly integrated onto the walls as observed for UT-CS samples. Chlorite extracts (Panel 5) did not exhibit any abundance of xyloglucan epitopes hinting the minimal interaction between xyloglucans and lignin components. Overall, there were no detectable variations in the post chlorite alkali extracted xyloglucan epitopes abundancies suggesting that most tightly integrated xyloglucan components are not affected by any of the pretreatment conditions.
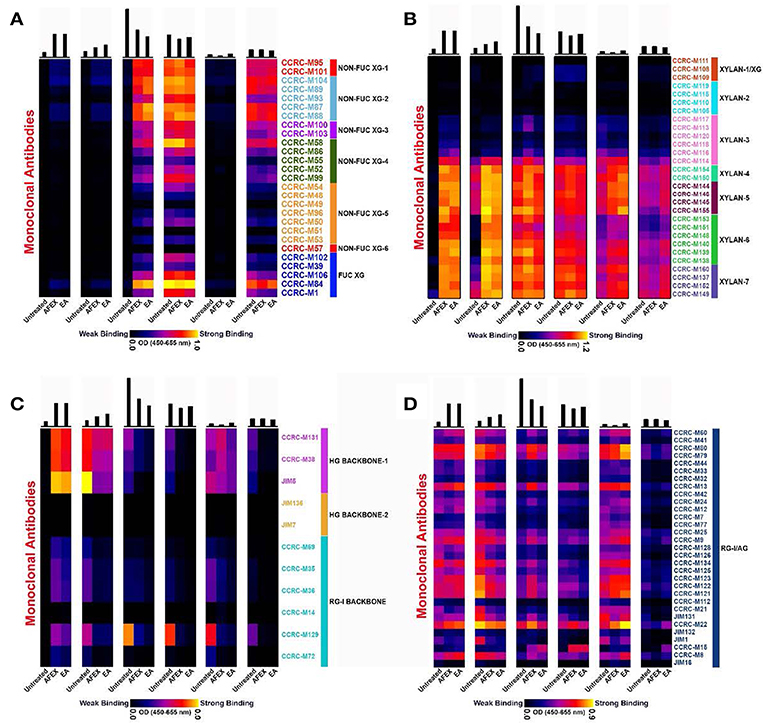
Figure 6. Glycome profiling of untreated and EA/AFEX pretreated of corn stover stems. Here, (A) xylanglucans epitopes of glycome profiling heatmap; (B) unsubstituted and substituted xylan epitopes of glycome profiling heatmap; (C) pectin backbone epitopes of glycome profiling heatmap; (D) pectic-arabinogalactan epitopes of glycome profiling heatmap. The six panels from left to right are cell wall extracts namely, P1 (50 mM Oxalate), P2 (50 mM carbonate), P3 (1M KOH), P4 (4M KOH), P5 (100 mM Chlorite), and P6 (4M KOH post Chlorite).
Figure 6B shows the profiles of unsubstituted and substituted xylan epitopes for untreated, AFEX and EA pretreated samples. Recent studies by Ruprecht et al. (2017) have characterized epitopes recognized by many of the xylan directed antibodies we employed here in detail. This study has shown that there are xylan antibody groups binding to longer stretches of β-1,4 xylan backbone epitopes such as most of the xylan 6 and 7 group of antibodies used in this study. This study also showed that CCRC M154 selectively binds to arabinose substitution at three position. Antibodies CCRC-M108, CCRC-M109, and CCRC-M110 bind specifically to xylan oligosaccharide epitope attached to arabinose substitution at two-position. These studies also showed that there are antibodies specific to un-methylated glucuronic acid side chain on xylan (CCRC-M150) and methylated glucuronic acid epitopes on xylan (e.g., CCRC-M144, CCRC-M145, CCRC- M146, and CCRC-M155). In summary, in our studies herein we have used the whole range of antibodies that could monitor substituted and unsubstituted xylan structures.
In contrast to what was observed for xyloglucan epitopes, xylan epitopes were extracted out by the least strong extracting reagents (oxalate and carbonate) shown in Panels 1 and 2 (Figure 6B). In the case of untreated biomass, while oxalate extract showed no xylan epitopes abundance, the carbonate extract contained some abundance of xylan epitopes detected by xylan-4 through 5 and xylan-7 groups of mAbs. But, interestingly, both pretreated biomass samples released significantly higher abundance of both unsubstituted and substituted xylan epitopes in both oxalate (Panel 1) and carbonate (Panel 2) extracts. This trend was similar in the case of actual carbohydrate materials recovered wherein both pretreated biomass samples exhibited higher levels of recovered carbohydrates (see top bar graphs) and EA conditions had more amounts released than AFEX conditions. Panel 3 (1M KOH) exhibited higher amounts of carbohydrates recovered as explained in the previous section. Chlorite extracts (Panel 5) from all samples exhibited significant abundance of unsubstituted and substituted xylan epitopes hinting much prevalent interaction between xylan and lignin components. A marginally higher abundance of xylan epitopes were noted in AFEX and EA samples probably due to pretreatment induced delignification in these samples. Overall, there were no detectable variations in the post chlorite alkali extracted xylan epitopes (Panel 6) abundancies suggesting that the most tightly integrated xyloglucan components are not affected by any of the pretreatment conditions tested herein.
Abundance and extractabilities of pectin backbone epitopes were monitored for untreated, AFEX and EA pretreated samples and the data are depicted in Figure 6C. All oxalate extractable pectic backbone epitopes (including HG backbone-1 and RG-I backbone epitopes) were significantly higher in the case of both pretreated samples. But, in all remaining cell wall extracts a reverse trend was noted with reduced extractability of pectic backbone epitopes (including HG backbone-1 and RG-I backbone epitopes) noted in both pretreated biomass samples. This particular trend is most evident with respect to HG backbone-1 mAbs and CCRC-M129 belonging to the RG-I backbone group of mAbs. None of the samples analyzed exhibited epitopes detected by HG backbone-2 group of mAbs.
The extractability and abundance of pectic-arabinogalactan epitopes were overall similar among most extracts from untreated, AFEX and EA pretreated samples and the data are depicted in Figure 6D. However, subtle variations in the abundance of these epitopes were noted. For instance, untreated samples exhibited higher abundance of pectic-arabinogalactan epitopes when compared with both pretreated samples. Again, AFEX pretreated samples exhibited higher abundance of pectic-arabinogalactan epitopes in comparison with other two sample types. Chlorite extracts (Panel 5) from all samples exhibited significant abundance of pectic-arabinogalactan epitopes hinting again much predominant interaction/s between pectic-arabinogalactan epitopes and lignin components. In the post chlorite extracts (Panel 6), in any of the sample types, we did not detect significant abundance of pectic-arabinogalactan epitopes potentially indicating overall absence of very tightly integrated pectic-arabinogalactan epitopes in the corn stover cell walls among these biomass samples. Glycome Profiling showed that the overall extractability of diverse glycan epitopes increased after EA pretreatment.
Understanding Cell Wall Ultrastructure Using Immunofluorescence Microscopy
Immunolabeling of cross sections from corn stover stem by using various antibodies was carried out in order to visualize the effect of EA pretreatment on the distribution of cell wall glycan epitopes in pretreated vs. untreated corn stover biomass. These studies were performed using monoclonal antibodies against three different classes of wall polymers: xylans (CCRC-M154, CCRC-M140 and CCRC-M144) antibodies, xyloglucans (CCRC-M88) and pectins (CCRC-M14 and CCRC-M38)-directed antibodies which successfully recognized the different composition changes between EA-CS and UT-CS. These antibodies epitope structures were showed in Figures 5II,III.
The antibody CCRC-M154 (Figure 5II, A and D) recognizes arabinoxylans, and selectively binds to the arabinose substitution in the three-position on the xylan backbone (Schmidt et al., 2015; Ruprecht et al., 2017). Arabinoxylan epitopes in the non-pretreated control (Figure 5II, A) showed more intense labeling in the vascular bundle. However, after the pretreatment (Figure 5II, D), the immunolabeling was more uniform in all cell walls. This indicates that arabinoxylan epitope structure is preserved, but, some of them might be removed or masked in the vascular bundle cell walls after the pretreatment. This is supported by the CCRC-M140 localization where there were more long stretches of xylan appeared in the vascular bundle after the pretreatment. CCRC-M140 (Figure 5II, B and E) recognizes long stretches (DP >4) of unsubstituted xylan backbone (Schmidt et al., 2015; Ruprecht et al., 2017). In control samples (Figure 5II, B), all the cell walls were labeled, but, reduced intensity of the labeling indicating the reduced accessibility of the mAb's to specific epitope they detect. In the pretreated sample (Figure 5II, E), labeling was stronger, especially in the vascular bundle cell walls presumable due to pretreatment induced de-lignification and subsequent increased accessibility of glycan epitopes to mAbs compared to the untreated samples (controls). The antibody CCRC-M144 (Figure 5II, C and F) recognizes 4-O-methyl glucoronoxylans (Schmidt et al., 2015; Ruprecht et al., 2017). Similarly, this epitope is not recognized in the control samples (Figure 5II, C), while, it is intensely recognized after the pretreatment (Figure 5II, F). Ammonia deacetylates acetyl linkages (Mitchell et al., 1990). De-acetylation of glucorono-xylan by the pretreatment allowed CCRC-M144 to recognize its epitope that is methyl glucorono-xylan. It is also known that the presence of O-acetyl groups hamper polysaccharide hydrolysis to constituent monosaccharides (Pawar et al., 2013). This clearly indicates that the EA pretreatment can benefit biofuel production by removing acetyl groups.
These results supported the conclusions drawn from glycome profiling that a marginally higher abundance of xylan epitopes were noted in pretreated samples probably due to pretreatment-induced delignification of these samples that further exposed the xylan epitopes. Similar to previous observations of AFEX pretreated biomass, EA pretreatment also causes a loosening of non-cellulosic glycan epitopes increasing their extractability or increasing their accessibility in-vitro to mAbs during labeling studies. This phenomenon is especially true to hemicellulose structures such as xylan as explained previously (Pattathil et al., 2015).
As for xyloglucan and pectin epitopes, three antibodies successfully recognized the modifications between EA-CS and UT-CS including the antibodies CCRC-M88, CCRC-M14 and CCRC-M38. The antibody CCRC-M88 (Figure 5III, A and D) recognizes galactosylated xyloglucan epitopes (Dallabernardina et al., 2017). The labeling with this antibody was stronger in the phloem and parenchyma cell walls in the control (Figure 5III, A), while, the labeling was left in the phloem area after the pretreatment (Figure 5III, D). The antibody CCRC-M14 (Figure 5III, B and E) recognizes RG-I backbone (Ruprecht et al., 2017). On the other hand, the antibody CCRC-M14 labeling was mostly localized to phloem cell walls (Figure 5III, B) and somewhat reduced after the pretreatment (Figure 5III, E). Interestingly, antibody CCRC-M38 (Figure 5III, C and F) recognizes HG backbone. The labeling with this antibody was strong in the phloem cell walls and cell corners in the control (Figure 5III, C), while, it disappeared after the pretreatment (Figure 5III, F). Similar conclusion with glycome profiling, during EA pretreatment, xyloglucan that are loosened from the cell walls by the effect of ammonia which are otherwise tightly integrated onto the plant cell walls as in the case of UT-CS samples. But, in contrast to xylan antibodies, xyloglucan and pectin-directed antibodies showed reduced labeling after the pretreatment. Particularly, cell loosening, increased glycan extractability, increased delignification, and increased accessibility of glycan epitopes to mAbs during labeling studies. The observed changes in glycan extractability and immunolabeling patterns of cell wall glycan epitopes provide us with a better understanding of the modifications that occur after different ammonia pretreatment.
Conclusions and Future Aspects
In this study, multiple techniques including, Scanning Electron Microscopy (SEM), Transmission Electron Microscopy (TEM), powder X-ray diffraction (pXRD), Immunofluorescence Microscopy and glycome profiling have been applied to explore the structural changes among UT-CS, AFEX-CS, and EA-CS. The soluble lignin components extracted during EA process created porous, interconnected tunnel-like networks that are much more accessible to enzymes. Results using these techniques demonstrated that ester bond cleavage in addition to previous NMR studies (da Costa Sousa et al., 2016a), lignin solubilization and cellulose allomorph changes has helped to get high yield of enzymatic hydrolysis compared to AFEX pretreatment. The conclusion described in this work has helped us to understand the ultrastructure, glycan composition, and extractability changes during EA pretreatment using cell wall glycan directed mAbs. Specifically, the EA pretreatment process causes a de-lignification effect on biomass. This is because the plant cell wall glycans that are otherwise integrated to cell wall through lignin get loosened up during EA pretreatment. Hence, hemicellulose integration is severally distorted by EA pretreatment process which give more accessibility to cellulolytic enzymes during hydrolysis. More research is under progress to understand the extracted cell wall components during EA pretreatment process.
Data Availability
All data generated or analyzed during this study are included in this published article with Figure S1.
Author Contributions
VB, SP, and LC collectively came up with the idea, contributed to the experimental design, helped in interpreting the results, and edited the manuscript. UA did most of the imaging working and SP did glycome profiling experiments. XZ formatted the manuscript and did some experiments. BD and MH initiated the collaboration, participated in the coordination of the study, were responsible for getting the funds for this project, and edited the manuscript. VB and YX led and coordinated the overall project and helped draft the manuscript. All authors read and approved the final manuscript.
Funding
The authors gratefully acknowledge financial support from the Priority Academic Program Development of Jiangsu Higher Education Institutions (PAPD), and acknowledge the Doctorate Fellowship Foundation of Nanjing Forestry University for supporting the work presented in this paper. The research was also supported by the Office of Biological and Environmental Research, Office of Science, United States, Department of Energy through DOE Great Lakes Bioenergy Research Center (GLBRC) Grant DE-FC02-07ER64494 and through BioEnergy Science Center administered by Oak Ridge National Laboratory and funded by a grant (DE-AC05-00OR22725). Generation of the CCRC series of monoclonal antibodies used in this work was supported by the NSF Plant Genome Program (DBI-0421683 and IOS-0923992). The authors also acknowledge University of Houston High Priority Area Research Seed Grants and the State of Texas for startup funds to VB.
Conflict of Interest Statement
SP is currently employed by company Mascoma LLC (Lallemand Inc.), New Hampshire, USA.
The remaining authors declare that the research was conducted in the absence of any commercial or financial relationships that could be construed as a potential conflict of interest.
Acknowledgments
We would like to express our sincere appreciation to Ms. Christa Gunawan who helped us to perform high solid loading enzyme hydrolysis and Mr. Lee Alexander for carrying out HPLC analysis for this work.
Supplementary Material
The Supplementary Material for this article can be found online at: https://www.frontiersin.org/articles/10.3389/fenrg.2019.00085/full#supplementary-material
Figure S1. Glycome profiling of untreated corn stover, AFEX pretreatment, EA pretreatment under different pretreatment conditions.
Abbreviations
AFEX, Ammonia Fiber Expansion; EA, Extractive Ammonia; UT, Untreated; CS, corn stover; UHS, unhydrolyzed solids; mAbs, monoclonal antibodies.
References
Avci, U., Pattathil, S., and Hahn, M. G. (2012). “Immunological approaches to plant cell wall and biomass characterization: immunolocalization of glycan epitopes,” in Biomass Conversion: Methods and Protocols, ed M. E. Himmel (Totowa, NJ: Humana Press), 73–82. doi: 10.1007/978-1-61779-956-3_7
Balan, V. (2014). Current challenges in commercially producing biofuels from lignocellulosic biomass. ISRN Biotechnol. 2014:463074. doi: 10.1155/2014/463074
Balan, V., Bals, B., Chndawat, S. P. S., Marshall, D., and Dale, B. E. (2009a). “Lignocellulosic biomass pretreatment using AFEX,” in Biofuels: Methods and Protocols, Methods in Molecular Biology, Vol. 581, ed J. R. Mielenz (Totowa, NJ: Humana Press, 61–77.
Balan, V., Sharma, L. N., Chambliss, C. K., da Costa Sousa, L., Chundawat, S. P., Marshall, D., et al. (2009b). Enzymatic digestibility and pretreatment degradation products of AFEX-treated hardwoods (Populus nigra). Biotechnol. Prog. 25, 365–375. doi: 10.1021/bp.160
Campbell, T. J., Teymouri, F., Bals, B., Glassbrook, J., Nielson, C. D., and Videto, J. (2013). A packed bed Ammonia Fiber Expansion reactor system for pretreatment of agricultural residues at regional depots. Biofuels 4, 23–24. doi: 10.4155/bfs.12.71
Chundawat, S. P. S., Bals, B., Campbell, T., Sousa, L., Gao, D., Jin, M., et al. (2013). “Primer on ammonia fiber expansion pretreatment,” in Aqueous Pretreatment of Plant Biomass for Biological and Chemical Conversion to Fuels and Chemicals, ed C. E. Wyman (Chichester: John Wiley & Sons), 169–200. doi: 10.1002/9780470975831.ch9
Chundawat, S. P. S., Bellesia, G., Uppugundla, N., Da Costa Sousa, L., Gao, D., Cheh, A. M., et al. (2011a). Restructuring the crystalline cellulose hydrogen bond network enhances its depolymerization rate. J. Am. Chem. Soc. 133, 11163–11174. doi: 10.1021/ja2011115
Chundawat, S. P. S., Donohoe, B. S., Da Costa Sousa, L., Elder, T., Agarwal, U. P., Lu, F., et al. (2011b). Multi-scale visualization and characterization of lignocellulosic plant cell wall deconstruction during thermochemical pretreatment. Energy Environ. Sci. 4, 973–984. doi: 10.1039/c0ee00574f
da Costa Sousa, L., Chundawat, S. P., Balan, V., and Dale, B. E. (2009). “Cradle-to-grave” assessment of existing lignocellulose pretreatment technologies. Curr. Opin. Biotechnol. 20, 339–347. doi: 10.1016/j.copbio.2009.05.003
da Costa Sousa, L., Foston, M., Bokade, V., Azarpira, A., Lu, F., Ragauskas, A. J., et al. (2016b). Isolation and characterization of new lignin streams derived from extractive-ammonia (EA) pretreatment. Green Chem. 18:4205–4215. doi: 10.1039/c6gc00298f
da Costa Sousa, L., Foston, M., Bokade, V., Azarpira, A., Lu, F., Ragauskas, A. J., et al. (2017). Whole cell biocatalysts: essential workers from nature to the industry. Microb. Biotechnol. 10, 4205–4215.
da Costa Sousa, L., Jin, M., Chundawat, S. P. S., Bokade, V., Tang, X., Azarpira, A., et al. (2016a). Next-generation ammonia pretreatment enhances cellulosic biofuel production. Energy Environ. Sci. 9, 1215–1223. doi: 10.1039/c5ee03051j
Dallabernardina, P., Ruprecht, C., Smith, P. J., Hahn, M. G., Urbanowiczc, B. R., and Pfrengle, F. (2017). Automated glycan assembly of galactosylated xyloglucan oligosaccharides and their recognition by plant cell wall glycan-directed antibodies. Org. Biomol. Chem. 15, 9996-10000. doi: 10.1039/c7ob02605f
DeMartini, J. D., Pattathil, S., Avci, U., Szekalski, K., Mazumder, K., Hahn, H. G., et al. (2011). Application of monoclonal antibodies to investigate plant cell wall deconstruction for biofuels production. Energy Environ. Sci. Dyn. 4, 4332–4339. doi: 10.1039/c1ee02112e
Donohoe, B. S., Decker, S. R., Tucker, M. P., Himmel, M. E., and Vinzant, T. B. (2008). Visualizing lignin coalescence and migration through maize cell walls following thermochemical pretreatment. Biotechnol. Bioeng. 101, 913–925. doi: 10.1002/bit.21959
Donohoe, B. S., Vinzant, T. B., Elander, R. T., Pallapolu, V. R., Lee, Y. Y., Garlock, R. J., et al. (2011). Surface and ultrastructural characterization of raw and pretreated switchgrass. Bioresour. Technol. 102, 11097–11104. doi: 10.1016/j.biortech.2011.03.092
Gao, D., Chundawat, S. P. S., Sethi, A., Balan, V., Gnanakaran, S., and Dale, B. E. (2013). Increased enzyme binding to substrate is not necessary for more efficient cellulose hydrolysis. Proc. Natl. Acad. Sci. U.S.A. 110, 10922–10927. doi: 10.1073/pnas.1213426110
Gao, X., Kumar, R., Singh, S., Simmons, B. A., Balan, V., Dale, B. E., et al. (2014). Comparison of enzymatic reactivity of corn stover solids prepared by dilute acid, AFEXTM, and ionic liquid pretreatments. Biotechnol. Biofuels 7, 1–13. doi: 10.1186/1754-6834-7-71
Gollapalli, L. E., Dale, B. E., and Rivers, D. M. (2002). Predicting digestibility of ammonia fiber explosion (AFEX)-treated rice straw. Appl. Biochem. Biotechnol. 98–100, 23–35. doi: 10.1385/ABAB:98-100:1-9:23
Gunawan, C., Xue, S., Pattathil, S., Da Costa Sousa, L., Dale, B. E., and Balan, V. (2017). Comprehensive characterization of non-cellulosic recalcitrant cell wall carbohydrates in unhydrolyzed solids from AFEX-pretreated corn stover. Biotechnol. Biofuels 10, 1–14. doi: 10.1186/s13068-017-0757-5
Hames, B., Ruiz, R., Scarlata, C., Sluiter, A., Sluiter, J., and Templeton, D. (2008). Preparation of Samples for Compositional Analysis Laboratory Analytical Procedure (LAP) Issue Date : 8/06/2008. National Renewable Energy Laboratory, Golden, CO, 1–9.
Himmel, M. E., Ding, S.-Y., K, D. J., Adney, W. S., Nimlos, M. R., Brady, J. W., et al. (2007). Biomass recalcitrance: engineering plants and enzymes for biofuels production. Science 315, 804–808. doi: 10.1126/science.1137016
Izquierdo, J. A., Pattathil, S., Guseva, A., Hahn, M. G., and Lynd, L. R. (2014). Comparative analysis of the ability of Clostridium clariflavum strains and Clostridium thermocellum to utilize hemicellulose and unpretreated plant material. Biotechnol. Biofuels 7, 1–8. doi: 10.1186/s13068-014-0136-4
Jin, M., Sousa, L. D. C., Schwartz, C., He, Y., Sarks, C., Gunawan, C., et al. (2016). Toward lower cost cellulosic biofuel production, using ammonia based pretreatment technologies. Green Chem.18, 957–966. doi: 10.1039/c5gc02433a
Kim, S., Dale, B. E., Jin, M., Thelen, K. D., Zhang, X., Meier, P., Sharara, M., et al. (2019). Integration in depot-based decentralized biorefinery system: i. corn stover cellulosic biofuel. GCB Bioenergy 2019, 871–882. doi: 10.1111/gcbb.12613
Kumar, S., and Sani, R. K. (2018). “Biorefining of biomass to biofuels - opportunities and perception. Part of the Biofuel and Biorefinery Technologies book series (BBT, volume 4). PSpringer Cham (Pubs). doi: 10.1007/978-3-319-67678-4
Li, M., Pattathil, S., Hahn, M. G., and Hodge, D. B. (2014). Identification of features associated with plant cell wall recalcitrance to pretreatment by alkaline hydrogen peroxide in diverse bioenergy feedstocks using glycome profiling. RSC Adv. 4, 17282–17292. doi: 10.1039/c4ra00824c
Mielenz, J. R. (2009). Biofuels: Method and Protocols, ed J. R. Mielenz (Totowa, NJ: Humana Press). doi: 10.1007/978-1-4939-6412-3
Mitchell, D., Grohmann, K., Himmel, M., Dale, B., and Schroeder, H. (1990). Effect of the degree of acetylation on the enzymatic digestion of acetylated xylans. J. Wood Chem. Technol. 10, 111–121. doi: 10.1080/02773819008050230
Mokomele, T., Da Costa Sousa, L., Balan, V., Van Rensburg, E., Dale, B. E., and Görgens, J. F. (2018a). Ethanol production potential from AFEX and steam-exploded sugarcane residues for sugarcane biorefineries. Biotechnol. Biofuels 11, 1–21. doi: 10.1186/s13068-018-1130-z
Mokomele, T., da Costa Sousa, L., Bals, B., Balan, V., Goosen, N., Dale, B. E., et al. (2018b). Using steam explosion or AFEX to produce animal feeds and biofuel feedstocks in a biorefinery based on sugarcane residues. Biofuels Bioprod. Biorefin. 12, 978–996. doi: 10.1002/bbb
Mosier, N., Wyman, C., Dale, B., Elander, R., Lee, Y. Y., Holtzapple, M., et al. (2005). Features of promising technologies for pretreatment of lignocellulosic biomass. Bioresour. Technol. 96, 673–686. doi: 10.1016/j.biortech.2004.06.025
Pattathil, S., Avci, U., Baldwin, D., Swennes, A. G., McGill, J. A., Popper, Z., et al. (2010). A comprehensive toolkit of plant cell wall glycan-directed monoclonal antibodies. Plant Physiol. 153, 514–525. doi: 10.1104/pp.109.151985
Pattathil, S., Avci, U., Miller, J. S., and Hahn, M. G. (2012). “Immunological approaches to plant cell wall and biomass characterization: glycome profiling,” in Biomass Conversion: Methods and Protocols, ed M. E. Himmel (Totowa, NJ: Humana Press), 61–72.
Pattathil, S., Hahn, M. G., Dale, B. E., and Chundawat, S. P. S. (2015). Insights into plant cell wall structure, architecture, and integrity using glycome profiling of native and AFEXTM-pre- treated biomass. J. Exp. Bot. 66, 4279–4294. doi: 10.1093/jxb/erv107
Pawar, P. M.-A., Koutaniemi, S., Tenkanen, M., and Mellerowicz, E. J. (2013). Acetylation of woody lignocellulose: significance and regulation. Front. Plant Sci. 4:118. doi: 10.3389/fpls.2013.00118
Ratnaparkhe, S., Venkatachalam, S., Hahn, M. G., and Pattathil, S. (2013). Analyses using cell wall glycan-directed monoclonal antibodies reveal xylan-degradation by two microbial glycosyl hydrolases in cell walls from poplar and switchgrass biomass. J. Bioremediation Biodegrad. S4:004. doi: 10.4172/2155-6199.S4-004
Ruprecht, C., Bartetzko, M. P., Senf, D., Dallabernardina, P., Boos, I., Andersen, M. C. F., et al. (2017). A synthetic glycan microarray enables epitope mapping of plant cell wall glycan-directed antibodies. Plant Physiol. 175, 1094–1104. doi: 10.1104/pp.17.00737
Sarks, C., Jin, M., Balan, V., and Dale, B. E. (2017). Fed - batch hydrolysate addition and cell separation by settling in high cell density lignocellulosic ethanol fermentations on AFEX TM corn stover in the Rapid Bioconversion with Integrated recycling Technology process. J. Ind. Microbiol. Biotechnol. 44, 1–12. doi: 10.1007/s10295-017-1949-5
Schmidt, D., Schuhmacher, F., Geissner, A., Seeberger, P. H., and Pfrengle, F. (2015). Automated synthesis of arabinoxylan-oligosaccharides enables characterization of antibodies that recognize plant cell wall glycans. Chem. A Eur. J. 21, 5709–5713. doi: 10.1002/chem.201500065
Shen, H., Poovaiah, C. R., Ziebell, A., Tschaplinski, T. J., Pattathil, S., Gjersing, E., et al. (2013). Enhanced characteristics of genetically modified switchgrass (Panicum virgatum L.) for high biofuel production. Biotechnol. Biofuels 6, 1–15. doi: 10.1186/1754-6834-6-71
Wyman, C. E., Dale, B. E., Elander, R. T., Holtzapple, M., Ladisch, M. R., and Lee, Y. Y. (2005). Coordinated development of leading biomass pretreatment technologies. Bioresour. Technol. 96, 1959–1966. doi: 10.1016/j.biortech.2005.01.010
Keywords: extractive ammonia, pretreatment, AFEX, biomass conversion, antibody, glycome profiling, biofuels, lignocellulosic biomass
Citation: Avci U, Zhou X, Pattathil S, da Costa Sousa L, Hahn MG, Dale B, Xu Y and Balan V (2019) Effects of Extractive Ammonia Pretreatment on the Ultrastructure and Glycan Composition of Corn Stover. Front. Energy Res. 7:85. doi: 10.3389/fenrg.2019.00085
Received: 10 June 2019; Accepted: 07 August 2019;
Published: 16 September 2019.
Edited by:
Seonghun Kim, Korea Research Institute of Bioscience and Biotechnology (KRIBB), South KoreaReviewed by:
Zhi-Hua Liu, Texas A&M University, United StatesRenliang Huang, Tianjin University, China
Copyright © 2019 Avci, Zhou, Pattathil, da Costa Sousa, Hahn, Dale, Xu and Balan. This is an open-access article distributed under the terms of the Creative Commons Attribution License (CC BY). The use, distribution or reproduction in other forums is permitted, provided the original author(s) and the copyright owner(s) are credited and that the original publication in this journal is cited, in accordance with accepted academic practice. No use, distribution or reproduction is permitted which does not comply with these terms.
*Correspondence: Yong Xu, eHV5b25nQG5pZnUuZWR1LmNu; Venkatesh Balan, dmJhbGFuQHVoLmVkdQ==
†These authors have contributed equally to this work