- 1School of Engineering, Newcastle University, Newcastle upon Tyne, United Kingdom
- 2Departamento de Ingeniería Químíca, Universidad de Castilla-La-Mancha, Ciudad Real, Spain
- 3Department of Energy Engineering, Zhejiang University, Hangzhou, China
- 4School of Natural and Environmental Sciences, Newcastle University, Newcastle upon Tyne, United Kingdom
The development of a sustainable catalyst for the oxygen reduction reaction (ORR) is still a major bottleneck for the scale-up and commercialization of Microbial Fuel Cells (MFCs). In this work, we have studied the utilization of iron-oxidizing bacteria (IOB) enriched from natural environment and Fe2+ in MFCs equipped with gas diffusion electrodes (GDEs) as an alternative to traditional Pt-based catalysts. In half-cells systems, the oxidation of Fe2+ into Fe3+ by IOB and its regeneration at the cathode produced constant current densities close to 2 A m−2 for more than 45 days. In MFCs operated in batch mode, significant pH changes in both compartment led to the instability of the system. However, when operated in continuous mode, pH remained stable in both compartments and MFCs produced maximum power densities of 1.1 W m−2 were then reached, compared to 0.5 W m−2 for MFCs equipped with Pt catalyst. Diffusion of oxygen through the GDEs improved mass transport and the performance of the MFCs, and avoided the utilization of costly aeration system. This IOB GDE system also provides a reproducible and fast start-up for biocathode for MFCs.
Introduction
Microbial fuel cells (MFCs) are a promising technology with widespread applications such as wastewater treatment with the ability to generate power from wastes in order to decrease the energy consumption associated with these applications (Seelam et al., 2018). However, MFC technologies are still limited to laboratory scale mainly due to the low power outputs generated or the limitations associated with their scale up (Du et al., 2007; Rahimnejad et al., 2015). A conventional MFC consists of two compartments (anodic and cathodic) which can be separated by a membrane. At the anode, electrochemically-active microorganisms oxidize the organics from wastes and release electrons, carbon dioxide, and protons. At the cathode, reduction reaction occurs: oxygen is the preferred terminal electron acceptor due to its virtually inexhaustible availability and its high value of standard potential (E0 = 1.229 V vs. Standard Hydrogen Electrode). However, kinetics of the oxygen reduction reaction (ORR) are sluggish, associated with high overpotentials which is why the ORR is still a bottleneck for the development of MFCs. Therefore, the cathode compartment is one of the main factor limiting the MFC performance in power generation (Yu et al., 2007, 2012; Rabaey et al., 2008; Rismani-Yazdi et al., 2008). Different methods have been suggested to improve the reduction reaction at the cathode. Using different electron acceptors with high reduction potential such as permanganate or ferricyanide showed improvement in terms electricity generation (Oh et al., 2004; Kong et al., 2010; Oliveira et al., 2013). However, due to the irreversibility of these reactions, the catholyte should be replaced regularly. Therefore, this method is not economically favorable especially at larger scale. The utilization of chemical catalysts such as platinum (Pt), transition metal-based catalysts (Yu et al., 2007, 2009), or activated carbon has been extensively studied in the past (Burkitt et al., 2016). However, they have disadvantages such as high cost, lack of robustness, and sustainability. A promising and sustainable alternative is to replace chemical catalysts with biological catalysts. Biological catalysts offer several advantages, as they are cheaper, more stable in the long term, and environmentally sustainable (He and Angenent, 2006; Liew et al., 2014; Milner et al., 2014). In the past few years, several studies have focused on aerobic biocathodes using mixed cultures of microorganisms enriched from natural environments with various communities responsible for the ORR (Huang et al., 2011). In a previous study on aerobic biocathode using activated sludge as inoculum, it was shown that Gammaproteobacteria was mainly responsible for the ORR with direct electron uptake from the cathode, with the biofilm enriched at an applied potential of +0.2 V vs. Ag/AgCl (Milner et al., 2016). In spite of the ability of aerobic biocathodes to consume the electron directly from the cathode, the development of the biofilm associated with the ORR is a long process (usually at least 50 days). The utilization of a chemical mediator can help overcome this limitation as some bacteria can reduce oxygen by using mediators as electron donors. Previous studies have shown that the acidophilic iron oxidizing bacteria (IOB) Acidithiobacillus ferrooxidans are able to catalyze ORR at the cathode compartment at the acidic pH (Ter Heijne et al., 2006; Carbajosa et al., 2010). It was reported that IOB are able to derive energy directly from the cathode (Carbajosa et al., 2010), however the presence of ferrous ions (Fe2+) as a mediator significantly improves the kinetics at the cathode and makes the biocathode much faster to develop. Acidithiobacillus ferrooxidans are autotrophs that use CO2 as the carbon source, derive energy from the oxidation of Fe2+ and use oxygen as electron acceptor at low pH (typically around 3). Fe3+ produced from the biological oxidation is then regenerated at the cathode to Fe2+ (Lovley, 1991). Oxidizing ferrous iron and reducing oxygen by the iron oxidisers in an acidic condition yields almost 30 KJ/mole energy for their growth. Using Calvin cycle to fix CO2, it has been estimated that Acidithiobacillus ferrooxidans oxidizes 71 mole Fe2+ to fix one mole CO2 (Hedrich et al., 2011). Although at neutral pH, neutrophilic iron oxidisers are able to oxidize Fe2+, the reduction of oxygen—and thus the energy gain for the bacteria—is more favorable at pH 2 than at pH 7 (Ferguson and Ingledew, 2008). A successful scaled-up MFC using acidophilic iron oxidisers at the cathode at pH 2 and acetate oxidation at the anode compartment by a bioanode biofilm resulted in a high power density of 2 W.m−2 (Ter Heijne et al., 2011). However, in this study, expensive materials were used such as a biopolar membrane to overcome the pH shifts in both compartments as well as costly Pt- and Ir-coated electrodes.
It was proven that the performance of aerobic biocathodes can be limited by the oxygen mass transfer to the biofilm (Milner and Yu, 2018). To provide sufficient dissolved oxygen in the catholyte, aeration by air pump was used at different flow rates, showing that increasing the flow rate led to an increase in the dissolved oxygen concentration and consequently of the cathode and cell potentials (Milner and Yu, 2018). However, using air pump is not economically viable. Passive aeration using gas diffusion electrodes (GDEs) showed the appealing alternative to aeration by pump as it was proven that using GDEs for development of aerobic biocathodes could produce cell power comparable to that using Pt as the cathode catalyst (Xia et al., 2013). Despite the ability of GDE in overcoming the limitation of oxygen mass transfer, not many studies focused on using GDE for development of aerobic biocathodes. In this study, we developed a MFC using an aerobic biocathode with Fe2+ as a mediator in a dual chamber reactor equipped with GDE. Acidithiobacillus ferrooxidans was enriched from natural environment and used as biocatalyst. Reactors were started as a batch and later changed to continuous to overcome pH shifts-related limitations. The performance of the developed MFC was compared with a similar system equipped with a GDE coated with Pt catalyst.
Materials and Methods
Enrichment of IOB
Inoculum for cathode compartment was collected from the iron-rich river sediment in Lake District, Cumbria, U.K. The sediment had an initial orange-brown color and a pH of 3.6. For the enrichment, 20 g L−1 FeSO4·7H2O was added to 100 mL of the environmental samples and kept in incubator at the temperature of 33°C and 170 rpm. One milliliter-samples were taken from the solution every 1–3 days to measure the pH and concentration of the Fe2+/Fe3+ ions using the ferrozine method (Viollier et al., 2000). The control was composed of the exact same composition as the enrichment sample but was autoclaved to ensure no living bacteria was present. When pH of the solution dropped to 2.0, the color of the solution was dark red-orange, 20% of the samples were transferred to a fresh medium composed by 20 g L−1 FeSO4·7H2O, 0.4 g L−1 (NH4)2SO4, 0.4 g L−1 KH2PO4, and 0.4 g L−1 MgSO4 (Fontmorin and Sillanpaa, 2015). After two more transfers, IOB were successfully enriched based on the color, pH, and the concentration of the Fe2+/Fe3+ ions (Ter Heijne et al., 2007; Malki et al., 2008; Hedrich et al., 2011), and 20% of the solution was transferred to the cathode compartment of the MFC.
BES Setup and Operation
Dual chamber cells with GDEs as cathodes were used in this study. Cells were made of 3 Perspex pieces; two pieces were used as the anode and cathode compartments with a working volume of 60 mL each. The two compartments were separated by an anion exchange membrane (AEM, Fumasep FFA-3-PK-130, Fumatech, Germany). Carbon paper with gas diffusion layer (GDL) was purchased from Quintech (H2315 I2 C6, Göppingen, Germany) and used as the GDE. Before the MFC operation, carbon paper was pre-treated to increase hydrophilicity by immersing the electrode in a mixture containing 20 mL of concentrated H2SO4 (98%) and 2 g of KMnO4 at room temperature for 2 min, washing properly by deionized water and finally heating at 150°C for 2 h (Qiu et al., 2015). In MFCs equipped with platinized carbon paper (Pt-C) cathode, the same carbon paper was used and coated with 0.5 mg cm−2 Pt. The surface of the cathode was 12.5 cm2 and a titanium sheet as a current collector. Carbon felt purchased from VWR (Cat. No. 43200.RR, Alfa Aesar, UK) was used as anode material. The carbon felt had dimensions of 2 × 3 cm and 0.5 cm thickness. Temperature was kept constant during the whole experiment at 30°C. MFCs were operated in duplicate for the both parts of the experiment.
Half-Cells
The first part was performed to investigate the development of acidophilic IOB biocathode and investigate its performance in terms of cathodic current consumption. During 47 days, BESs were operated in 3 electrode configuration (half-cells) with carbon paper GDEs as working electrodes at an applied potential of 200 mV vs. Ag/AgCl. Potential was controlled using a Quad Potentiostat (Whistonbrook Technologies, U.K.). Platinum mesh and Ag/AgCl (BASi, RE-5B) were used as counter and reference electrodes, respectively. Cathode medium consisted of the same chemicals used in the enrichment step (pH 2) but with lower concentration of 1 g/L FeSO4·7H2O. 20% of the cathode medium was inoculated with IOB from the enrichment step. Anode medium was the same medium used in cathode compartment without FeSO4·7H2O and inoculum. A control was operated with the similar medium without IOB inoculum. All the potentials in this study are reported against Ag/AgCl, unless stated otherwise.
MFC Set Up and Operation
The second part of the experiment was carried out in full MFCs with external resistors of 200 Ω. Figure 1 shows the MFC operated for this part of experiment. Cell voltages and anode potentials were recorded using a Pico Technology ADC-24 data logger (St Neots, U.K.). MFCs were first operated with Pt-C at the cathode in order to develop bioanodes. Graphite felts with the projected surface area of 4.9 cm2 were used as the anode, using titanium sheet as current collector. Twenty percentage of the anode compartment was inoculated with the effluent of an MFC running in the laboratory over a year using glucose and acetate as carbon sources. The bacterial community of the inoculum was dominated by Geobacter (Spurr et al., 2018). Anode medium consisted of 50 mM phosphate buffer (PBS), 1 g L−1 of sodium acetate, 25 mL L−1 of macronutrients solution, 1 mL L−1 of micronutrients solution, and 0.5 mL L−1 of vitamins solution (see composition in Table S1).
The anolyte was replaced by fresh medium every 4 days after completion of one batch cycle (after the current generated would drop). After development of bioanodes, Pt-Cs were replaced with iron oxidizing biocathode developed in the half-cells and the same medium as used in half-cells was used. In continuous mode, 30 mL h−1 flow rate was used for both anolyte and catholyte using a dosing pump. The strategy for the BES operation in this study is summarized in Table 1.
Analysis
Electrochemical Analysis
Cyclic voltammetry (CV) was performed using Metrohm Autolab potentiostat (Runcorn, U.K.). All the CVs were recorded at a scan rate of 2 mV s−1.
Polarization Curves
After reaching the stable cell voltage in the MFC, polarization, and power density curves were recorded. The values of the external resistances used were: open circuit, 100 kΩ, 52.2 kΩ, 25.6 kΩ, 10.0 kΩ, 5.1 kΩ, 2.5 kΩ, 997 Ω, 505 Ω, 301 Ω, 103 Ω, 51 Ω, 12 Ω. The cell voltages and anode potentials for each resistance were recorded by data logger. The Ohm law was used to calculate the current values for each voltage and resistance.
Results
Enrichment of IOB From Mixed Culture of Microorganisms
The enrichment of IOB was followed visually, with pH and iron speciation measurements. Environmental samples from Lake District (United Kingdom) were initially brown and turned to red-orange after 5 days of enrichment. The change in the color of the inoculated solutions was accompanied by a significant drop of pH (Figure 2). The pH of both inoculated and control solutions decreased from 3.5 to 2.7 on day 6, and to just under 2 on day 7, while the pH value of the control solution stayed stable circa 2.9. These observations can be associated with the faster and more advanced oxidation of Fe2+ into Fe3+ due to the presence and growth of IOB in the inoculated samples (Fontmorin and Sillanpaa, 2015).
In aerobic conditions, it is known that the oxidation of Fe2+ can occur chemically. The rate of this reaction (Equation 1) depends on the environment conditions such as concentration of protons and dissolved oxygen (Stumm and Morgan, 1996):
Where k is the rate constant of the reaction (3 × 10−12 mol L−1 min−1 at 20°C). Therefore, at pH 2, the spontaneous oxidation of ferrous ions by molecular oxygen is very limited. In addition, at such pH, the redox potential of the oxygen/water couple is about 0.3 V more positive than at pH 7, which makes the oxidation of iron and the utilization of oxygen as electron acceptor energetically favorable for IOB (Hedrich et al., 2011).
At day 11 (see Figure 2), 20% of the inoculum was transferred to the fresh medium containing 20 g L−1 FeSO4·7H2O. Higher rate of the pH drop in the solution with inoculum compared to the control solution after the transfer and change in the color of the inoculated solution confirmed the biological conversion of Fe2+ to Fe3+ (Ter Heijne et al., 2007). The color of the control solution was orange-brown during the 20 days of the enrichment step. Fe2+ concentration was also measured during the enrichment step using ferrozine method (Viollier et al., 2000), indicating the decrease in the concentration of the Fe2+ in the inoculated solution while Fe2+ concentration was stable in the control solution (data not shown). The difference in color, pH, and Fe2+ concentration between the inoculated and control solutions indicated the successful enrichment of IOB in the inoculated solution which was used as the inoculum for the half-cells and MFCs experiments.
IOB as Biocatalyst and Fe2+ as a Mediator in Half-Cell Systems
Half-cells equipped with GDEs were operated at 0.2 V and inoculated with the enriched environmental sample. Figure 3 shows the current density of one of the duplicate half-cells during the 47 days of half-cell experiment and the control without inoculum during the 17 days of the abiotic experiment. Similar current density was observed in the second half-cell (duplicate) as presented in Figure S1. Very low current density was measured over 17 days of the experiment in the control cell, therefore it was terminated after this period. Electron uptake was observed in the half-cells inoculated from the beginning of the experiment: current densities of 1 A m−2 were measured just after inoculation and about 2 A m−2 after day 5 and for more than 45 days of operation (Figure 3). The presence of Fe2+/Fe3+ as a mediator with iron-oxidizing bacteria (IOB) as biocatalyst allows to avoid the long start-up usually associated with the development of biocathodes catalyzing the ORR. It was reported in previous studies that IOB such as Acidithiobacillus ferrooxidans can accept electrons directly from a cathode without the need for Fe2+ as a mediator (Carbajosa et al., 2010). However, in this case, the current generation only appeared after 22 days which corresponded to the time necessary for a biofilm to grow directly at the surface of the electrode. In addition, maximum current densities were measured at a potential of 0.05 V, potential corresponding to the catalysis of the ORR by the biofilm (Carbajosa et al., 2010).
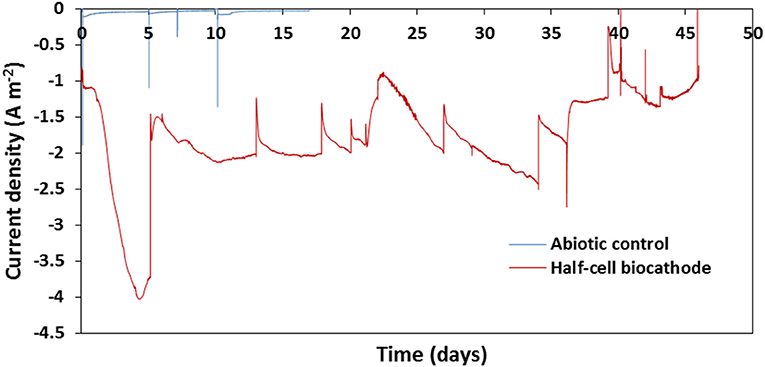
Figure 3. Chronoamperometry of half-cell poised at 0.2 V and inoculated by enriched IOB during the 47 day experiment and control half-cell during the 17 days abiotic experiment.
In order to confirm the mechanism occurring in the system used in the study, CVs were recorded after 47 days of operation, and compared with these recorded on a Pt-C GDE (Figure 4). The voltammogram recorded on the Pt-C GDE (Figure 4A) shows a clear catalysis of the ORR with an onset potential around 0.4 V. It was reported that in absence of mediator, IOBs are able to accept electron directly from a cathode to catalyze the reduction of oxygen (Carbajosa et al., 2010). In our system using IOB and iron as mediator, however, there is no obvious shift of the onset potential of the ORR but the presence of a pseudo-reversible signal of E0′ about 0.4 V which can be attributed to the Fe2+/Fe3+ redox couple. In addition, the very little difference between the CVs recorded in presence and absence of oxygen (Figure 4B) suggests the limited impact of oxygen on the reaction occurring at the electrode. These observations would confirm a mediated electron transfer mechanism between the cathode and IOB and that in presence of iron in the medium there is no direct biological catalysis of the ORR occurring at the electrode, despite oxygen being used as a terminal electron acceptor by IOB.
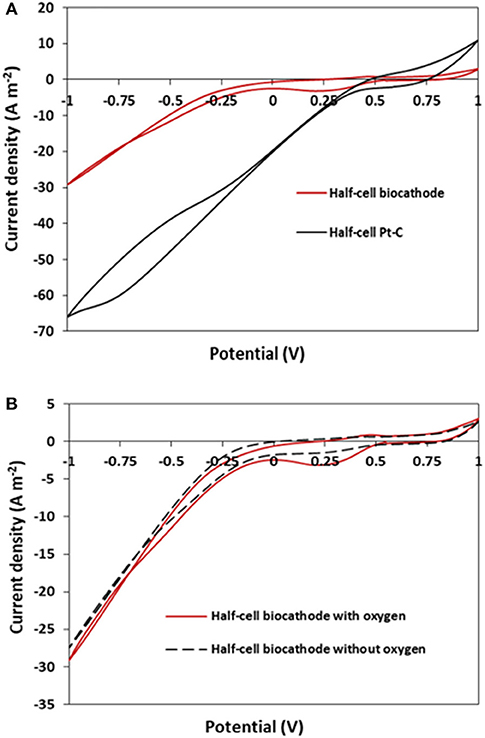
Figure 4. (A) Voltammograms recorded in half-cell reactors equipped with GDEs with Pt-C (black) and IOB biocathode as catalysts (red); (B) voltammograms recorded in half-cells reactors equipped with GDEs and IOB biocathode as catalyst after 47 days of operation, in presence (red) and absence (dashed black) of oxygen. CVs recorded at a scan rate of 2 mV s−1.
The proposed mechanism occurring at the electrode is presented in Figure 5. After the cells were opened, a red-orange layer could be observed at the surface of the GDEs. This was most likely due to the deposition of layer of iron oxides/hydroxides. It can thus be expected that the cathode potential of the MFCs equipped with IOB as biocatalyst and Fe2+ as mediator will be close to the reduction potential of Fe3+ into Fe2+ observed in Figure 4, i.e., about 0.3 V.
IOB as Biocatalyst and Fe2+ as Mediator in MFCs Equipped With GDEs
Development of Bioanodes
Following the half-cells experiments, MFCs were set up with GDE cathodes equipped with either Pt-C or IOB. Bioanodes were first developed using Pt-C as cathodes. The effluent from a glucose/glutamic acid-fed parent MFC was used as inoculum. Figure S2 shows the anode and MFC potentials during the bioanode development stage. As can be seen in Figure S2A, a quick start-up of the electricity generation after 1 day, reaching a cell potential of 0.3 V after 2 days. The other duplicate showed a very similar trend. To confirm the bioanode development, CVs were recorded on day 15 of MFC operation, after the 7th batch cycle, after acetate was depleted (Figure S3). Redox features appeared at potentials of −0.1, −0.30, −0.37 and −0.47 V which can be associated with the electron transfer between the biofilm and the electrode, as it was reported in previous studies involving bioanode formed by G. sulfurreducens (Fricke et al., 2008; Richter et al., 2009). In addition, polarization and power density curves of the cell showed maximum power production of 0.6 W m−2 and open circuit potential (OCP) of 0.74 V (Figure S2B). Similarly, MFC 2 reached power densities of 0.52 W m−2 and OCP of 0.73 V.
Performance of MFCs Equipped With GDE and IOB Using Fe2+ as Mediator
After the development of bioanodes, the platinized GDEs were replaced by the GDEs used in the half-cell experiment discussed in section IOB as Biocatalyst and Fe2+ as a Mediator in Half-Cell Systems. The objective was then to compare the performance of MFCs equipped with IOBs and iron at the cathode with the MFCs equipped with Pt-C catalyst. As demonstrated in Figure 4, the CVs recorded on the GDE with IOBs present a pseudo-reversible signal of E0′ about 0.4 V. There is no apparent catalysis of the ORR and the expected potential of the cathode in the MFC is therefore the potential of reduction of Fe2+/Fe3+ mediator, i.e., about 0.3 V.
Cell, anode, and cathode potentials after replacing the cathodes are reported in Figure 6. After switching cathodes, the cell potential decreased to 0.25 V after 1 h and to 0.04 V after 1 day, before reaching almost zero at the end of day 2. The drop of potential was associated with the significant drop in the anode potential, as depicted in Figure 6. After day 2, media of both compartments were replaced with fresh media, the cell potential reached 0.13 V and remained stable for almost 3 days before dropping back to zero on day 5. This drop was accompanied by significant changes in the pH of the anolyte and catholyte.
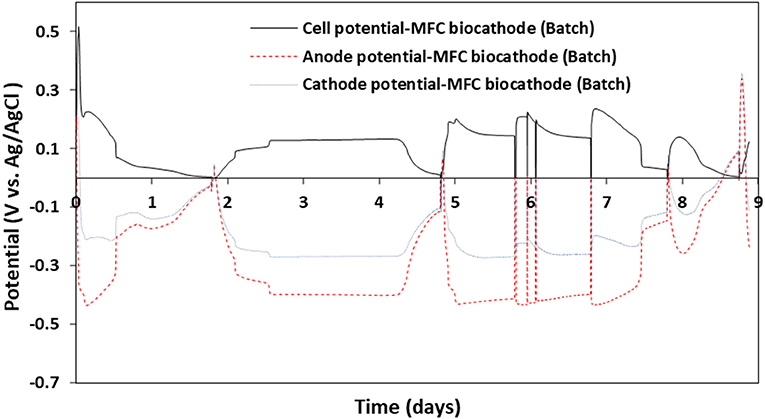
Figure 6. Cell, anode, and cathode potentials of MFC equipped with GDE and IOB biocathode and operated in fed-batch mode.
Despite using 50 mM phosphate buffer at pH 7 in the anode compartment, the pH dropped to 4 after 2 days due to the high difference between the pH of the anolyte (pH 7) and catholyte (pH 2) separated by AEM. Although daily media refreshment helped reaching cell potential of 0.2 V, such a potential could only be sustained for a few hours as the pH of the anolyte would rapidly decrease to 5. Similarly, a significant change was observed in the pH of the cathodic solution. Over the first 5 days after switching the cathodes, the pH of catholyte raised to about 5. The pH could be decreased to around 3 with regular medium replacement; however, it couldn't be stabilized at the initial and optimal pH of 2. The performance and pH of the duplicate MFC followed the similar trend, as presented in Figure S4. Polarization and power density curves of the MFCs equipped with IOBs as biocatalyst and iron as mediator were recorded and compared with the MFC equipped with Pt catalyst (Figure S5 and also Figure 8A). As can be seen, the inability to control the pH had a big impact on the performance of the cells as the maximum power density achieved was 0.59 W m−2 for MFCs equipped with Pt compared to 0.12 W m−2 in the MFCs by IOB in batch mode, which was similar in duplicates. This change is also associated with higher internal resistance in the MFC, as suggested by the increase of the slope of the polarization curve. It is understood that pH of both anolyte and catholyte have a great impact on MFCs performance. It was shown that the optimal value of anolyte pH is between 7 and 8 and that a pH outside this range decreases the MFC performance (He et al., 2008). Previous studies reported that acidic anolyte (pH 5) affects the microbial activity of the biofilm which then led to lower current generation (Gil et al., 2003; Ren et al., 2007). Similarly, in the presence of IOBs and iron as catalyst and mediator, pH higher than 2 in the cathodic compartment results in iron precipitation which affects the performance of the biocathode (Ter Heijne et al., 2006). In addition, and as described in section Enrichment of IOB, pH of 2 provides optimal conditions for acidophilic IOB to produce ATP from the oxidation of Fe2+ and using molecular oxygen as terminal electron acceptor (Hedrich et al., 2011). Therefore, the pH of both the catholyte and anolyte needs be controlled to maintain the MFCs performance.
MFC Performance With IOB Biocathode Operated in Continuous Mode
In order to control the pH in both compartments and avoid using expensive bipolar membranes, the cell operation was converted from fed-batch to continuous mode using a dosing pump for both anolyte and catholyte with similar hydraulic retention times (HRTs) of 2.0 h. Such a low HRT helped stabilize pH in both compartments and significantly improved MFCs performance compared to fed-batch operation. Over the whole experiment in continuous mode, pH of the anode and cathode media remained stable around 6.8 and 2.0, respectively. Five hours after switching from fed-batch to continuous mode, the anode potential dropped to −0.4 V whereas the cathode potential increased to −0.1 V, resulting in a cell voltage of 0.3 V (Figure 7). The anode potential stabilized at −0.45 V and the cathode potential kept increasing slowly to reach −0.05 V after 65 h, for a corresponding cell potential of ~0.4 V. In order to confirm that the oxygen mass transfer was not limiting the bio-electrochemical process, the catholyte reservoir tank was purged with air using an aquarium air pump for 8 h (between hours 40 and 48). As can be seen in Figure 7, no significant change in cathode potential nor in cell voltage was observed, showing that the passive diffusion of oxygen through the GDE is not a limitation to the performance of the MFCs. Therefore, such a design allows to avoid expensive aeration system which is not viable especially at larger scale.
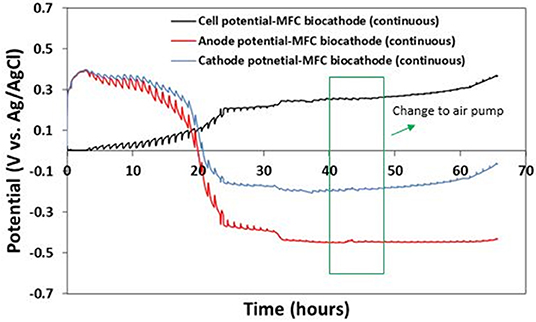
Figure 7. Cell, anode, and cathode potentials of MFC with IOB biocathode operated in continuous mode. Green box shows the time between hours 40 and 48 that catholyte reservoir tank was aerated using an aquarium air pump.
Previously, GDEs were mainly used for abiotic cathodic reactions such as catalysis of the ORR. It was for example reported that air-cathode MFC with GDE modified with activated carbon produced a comparable power to the MFC operated with platinum as the cathode after 1 month of operation (Zhang et al., 2014). To date, only few studies focused on the utilization of GDEs for aerobic or anaerobic biocathodes formation. In previous research, GDE design was used for the diffusion of CO2 through the GDL to provide sufficient CO2 for the development of anaerobic biocathode (Bajracharya et al., 2016; Srikanth et al., 2018). It was reported in this study that mass transfer of carbon dioxide was higher than when purging directly in the catholyte, leading to the improvement of the BES performance in terms of CO2 reduction and bio-production. Studies focusing on MFCs equipped with GDE for aerobic biocathodes are still scarce compared to systems with aeration, but the possibility to grow aerobic biofilms on such electrodes was already shown in the past (Wang et al., 2013; Xia et al., 2013; Montpart et al., 2018) However, the growth of such biofilms is time-consuming (usually at least 50 days), takes much longer time than the growth of bioanodes and is very challenging on non-porous materials such as carbon paper (Rimboud et al., 2015). Therefore, the combination of GDE with IOBs as biocatalyst and Fe2+ as mediator stands as a good alternative to classical Pt-based catalysts in aerated system.
Figure 8A shows the polarization and power density curves of the MFC after 65 h of continuous operation in comparison with the polarization and power density figures of the MFC in fed-batch mode using platinized carbon paper and biocathode. A maximum power density of 1.02 W m−2 was produced in continuous mode compared to 0.12 W m−2 in fed-batch mode using IOBs and 0.59 W m−2 using platinized carbon paper as cathode (see in Table 2). By stabilizing pH of both the anolyte and catholyte, continuous mode operation led to significant improvement of the MFC outputs, then outperforming the MFC equipped with Pt catalyst. It can however be observed that there is a “doubling back” of the power curve for current densities exceeding 1.7 A m−2 which is also known as “power overshoot” (Kim et al., 2017). Similar phenomenon was observed in MFCs equipped with aerobic biocathodes (Milner and Yu, 2018), which was further attributed to the impact of aeration and DO concentration in solution on the cathode and cell potentials as there was a sharp decrease on cathode potential. However, in the present study, the utilization of an air pump did not have any significant impact on the cell performance, showing that the GDE configuration provided sufficient oxygen to the system. Therefore, the cause of sudden drop in performance in this study was due to different reason.
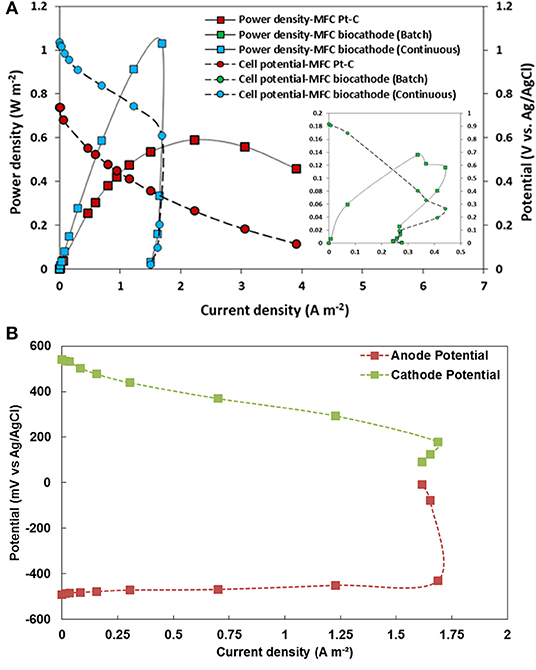
Figure 8. (A) Polarization curve and power density of MFC equipped with different cathodes and operated in batch or continuous mode; (B) Anode and cathode potentials during the polarization of the MFC equipped with IOB biocathode and operated in continuous mode.
The doubling back observed in Figure 8A can be explained with the potential profiles of the anode and cathode recorded during the polarization and presented in Figure 8B. As can be seen, the anode potential is relatively stable between −0.45 and −0.4 V until the current density reached 1.68 A m−2. At this current density, the cathode potential reaches ~0.25 V which is the potential at which Fe3+ starts to be electrochemically reduced into Fe2+ (as shown in CVs presented in Figures 5, 7). The current required by the cathode thus increases and exceeds what the bioanode can supply. At this stage, it can be assumed that the bioanode becomes limiting, thus explaining the drop of both the anode potential and the cell voltage. Although the cathode potential also decreased, it did not seem as significant as the drop of the anode potential. In MFCs using oxygen as terminal electron acceptor, the power output is usually limited by the performance of the cathode, whether the ORR is catalyzed by Pt or biocatalysts (Milner et al., 2016). Nevertheless, in the presence of IOBs as Fe2+ as mediator, and according to the potential profiles presented in Figure 8B, the bioanode appears to be limiting. This phenomenon was explained in a previous study showing that the power overshoot can occur when anodic kinetics are slower than cathodic kinetics, thus causing electrons depletion (Kim et al., 2017). In our specific case, this could be explained by the development of a non-mature biofilm due to a too short inoculation process at lower external resistances (Hong et al., 2011), or in other words by a biocathode outperforming the bioanode.
Conclusions
In this study, we demonstrated the feasibility of using IOB as biocatalyst and Fe2+ as a mediator as an alternative to Pt catalyst in MFCs equipped with GDEs. IOB were enriched from a natural environment sample and first used as an inoculum in half-cell reactors. Stable current densities of 2 A m−2 were produced during 45 days. Due to the presence of Fe2+ as mediator, no lag time was observed before such current densities were reached, thus avoiding the long start-up phase usually associated with aerobic biocathodes. Although the contribution of a direct electron transfer between the cathode and the bacteria cannot be totally ruled out, cyclic voltammogramms strongly suggest mediated electron transfer (by Fe2+) as the main mechanism. In the second part of the study, when MFCs were operated in batch mode, the performances of the cells containing IOB as biocatalyst were comparable to these equipped with Pt. However, these performances could not be sustained due to the drop of pH in the anodic compartment and the increase of pH in the cathodic compartment. Operating MFCs in continuous mode helped stabilize pH in both compartments. Maximum power densities of 1.02 W m−2 were then reached, compared to 0.59 W m−2 for MFCs equipped with Pt catalyst. Finally, the passive diffusion of oxygen through the GDEs did not appear as a limiting factor to the performance of the MFCs, showing that the system studied represent or good alternative to both chemical catalysts and non-mediated aerobic biocathodes without the need for costly aeration systems.
Data Availability
The datasets generated for this study are available on request to the corresponding author.
Author Contributions
All authors listed have made a substantial, direct and intellectual contribution to the work, and approved it for publication.
Conflict of Interest Statement
The authors declare that the research was conducted in the absence of any commercial or financial relationships that could be construed as a potential conflict of interest.
Acknowledgments
The authors thank EPSRC LifesCO2R project (EP/N009746/1), EPSRC NECEM (EP EP/R021503/1) and NERC MeteoRR (NE/ NE/L014246/1). PI thanks the Doctoral Training Awards (SAgE DTA, 2015 cohort) from Faculty of Science, Agriculture and Engineering, Newcastle University for supporting her PhD study. The authors thank William Foxall for his contribution in preliminary experiments.
Supplementary Material
The Supplementary Material for this article can be found online at: https://www.frontiersin.org/articles/10.3389/fenrg.2019.00093/full#supplementary-material
References
Bajracharya, S., Vanbroekhoven, K., Buisman, C. J. N., Pant, D., and Strik, D. P. (2016). Application of gas diffusion biocathode in microbial electrosynthesis from carbon dioxide. Environ. Sci. Pollut. Res. 23, 22292–22308. doi: 10.1007/s11356-016-7196-x
Burkitt, R., Whiffen, T. R., and Yu, E. H. (2016). Iron phthalocyanine and MnOx composite catalysts for microbial fuel cell applications. Appl. Catal. B Environ. 181, 279–288. doi: 10.1016/j.apcatb.2015.07.010
Carbajosa, S., Malki, M., Caillard, R., Lopez, M. F., Palomares, F. J., Martin-Gago, J. A., et al. (2010). Electrochemical growth of Acidithiobacillus ferrooxidans on a graphite electrode for obtaining a biocathode for direct electrocatalytic reduction of oxygen. Biosens. Bioelectron. 26, 877–880. doi: 10.1016/j.bios.2010.07.037
Du, Z., Li, H., and Gu, T. (2007). A state of the art review on microbial fuel cells: a promising technology for wastewater treatment and bioenergy. Biotechnol. Adv. 25, 464–482. doi: 10.1016/j.biotechadv.2007.05.004
Ferguson, S. J., and Ingledew, W. J. (2008). Energetic problems faced by micro-organisms growing or surviving on parsimonious energy sources and at acidic pH: I. Acidithiobacillus ferrooxidans as a paradigm. Biochim. Biophys. Acta. 1777, 1471–1479. doi: 10.1016/j.bbabio.2008.08.012
Fontmorin, J. M., and Sillanpaa, M. (2015). Bioleaching and combined bioleaching/Fenton-like processes for the treatment of urban anaerobically digested sludge: removal of heavy metals and improvement of the sludge dewaterability. Sep. Purif. Technol. 156, 655–664. doi: 10.1016/j.seppur.2015.10.061
Fricke, K., Harnisch, F., and Schröder, U. (2008). On the use of cyclic voltammetry for the study of anodic electron transfer in microbial fuel cells. Energy Environ. Sci. 1, 144–147. doi: 10.1039/b802363h
Gil, G.-C., Chang, I.-S., Kim, B. H., Kim, M., Jang, J.-K., Park, H. S., et al. (2003). Operational parameters affecting the performannce of a mediator-less microbial fuel cell. Biosens. Bioelectron. 18, 327–334. doi: 10.1016/S0956-5663(02)00110-0
He, Z., and Angenent, L. T. (2006). Application of bacterial biocathodes in microbial fuel cells. Electroanalysis 18, 2009–2015. doi: 10.1002/elan.200603628
He, Z., Huang, Y., Manohar, A. K., and Mansfeld, F. (2008). Effect of electrolyte pH on the rate of the anodic and cathodic reactions in an air-cathode microbial fuel cell. Bioelectrochemistry 74, 78–82. doi: 10.1016/j.bioelechem.2008.07.007
Hedrich, S., Schlömann, M., and Barrie Johnson, D. (2011). The iron-oxidizing proteobacteria. Microbiology 157, 1551–1564. doi: 10.1099/mic.0.045344-0
Hong, Y. Y., Call, D. F., Werner, C. M., and Logan, B. E. (2011). Adaptation to high current using low external resistances eliminates power overshoot in microbial fuel cells. Biosens. Bioelectron. 28, 71–76. doi: 10.1016/j.bios.2011.06.045
Huang, L., Regan, J. M., and Quan, X. (2011). Electron transfer mechanisms, new applications, and performance of biocathode microbial fuel cells. Biores. Technol. 102, 316–323. doi: 10.1016/j.biortech.2010.06.096
Kim, B., An, J., and Chang, I. S. (2017). Elimination of power overshoot at bioanode through assistance current in microbial fuel cells. Chemsuschem 10, 612–617. doi: 10.1002/cssc.201601412
Kong, X., Sun, Y., Yuan, Z., Li, D., Li, L., and Li, Y. (2010). Effect of cathode electron-receiver on the performance of microbial fuel cells. Int. J. Hydrog. Energy 35, 7224–7227. doi: 10.1016/j.ijhydene.2010.03.106
Liew, K. B., Daud, W. R. W., Ghasemi, M., Leong, J. X., Lim, S. S., and Ismail, M. (2014). Non-Pt catalyst as oxygen reduction reaction in microbial fuel cells: a review. Int. J. Hydrog. Energy 39, 4870–4883. doi: 10.1016/j.ijhydene.2014.01.062
Lovley, D. R. (1991). Dissimilatory Fe (III) and Mn (IV) reduction. Microbiol. Rev. 55, 259–287. doi: 10.1007/978-3-642-30141-4_69
Malki, M., De Lacey, A. L., Rodríguez, N., Amils, R., and Fernandez, V. M. (2008). Preferential use of an anode as an electron acceptor by an acidophilic bacterium in the presence of oxygen. Appl. Environ. Microbiol. 74, 4472–4476. doi: 10.1128/AEM.00209-08
Milner, E., Scott, K., Head, I., Curtis, T., and Yu, E. (2014). “Electrochemical investigation of aerobic biocathodes at different poised potentials: evidence for mediated extracellular electron transfer. Chem. Eng. Trans. 41, 355–360. doi: 10.3303/CET1441060
Milner, E. M., Popescu, D., Curtis, T., Head, I. M., Scott, K., and Yu, E. H. (2016). Microbial fuel cells with highly active aerobic biocathodes. J. Power Sources 324, 8–16. doi: 10.1016/j.jpowsour.2016.05.055
Milner, E. M., and Yu, E. H. (2018). The effect of oxygen mass transfer on aerobic biocathode performance, biofilm growth and distribution in microbial fuel cells. Fuel Cells 18, 4–12. doi: 10.1002/fuce.201700172
Montpart, N., Rago, L., Baeza, J. A., and Guisasola, A. (2018). Oxygen barrier and catalytic effect of the cathodic biofilm in single chamber microbial fuel cells. J. Chem. Technol. Biotechnol. 93, 2199–2207. doi: 10.1002/jctb.5561
Oh, S., Min, B., and Logan, B. E. (2004). Cathode performance as a factor in electricity generation in microbial fuel cells. Environ. Sci. Technol. 38, 4900–4904. doi: 10.1021/es049422p
Oliveira, V. B., Simões, M., Melo, L. F., and Pinto, A. (2013). Overview on the developments of microbial fuel cells. Biochem. Eng. J. 73, 53–64. doi: 10.1016/j.bej.2013.01.012
Qiu, Y., Cheng, Z., Guo, B., Fan, H., Sun, S., Wu, T., et al. (2015). Preparation of activated carbon paper through a simple method and application as a supercapacitor. J. Mater. Sci. 50, 1586–1593. doi: 10.1007/s10853-014-8719-9
Rabaey, K., Read, S. T., Clauwaert, P., Freguia, S., Bond, P. L., Blackall, L. L., et al. (2008). Cathodic oxygen reduction catalyzed by bacteria in microbial fuel cells. ISME J. 2, 519–527. doi: 10.1038/ismej.2008.1
Rahimnejad, M., Adhami, A., Darvari, S., Zirepour, A., and Oh, S.-E. (2015). Microbial fuel cell as new technology for bioelectricity generation: a review. Alex. Eng. J. 54, 745–756. doi: 10.1016/j.aej.2015.03.031
Ren, Z., Ward, T. E., and Regan, J. M. (2007). Electricity production from cellulose in a microbial fuel cell using a defined binary culture. Environ. Sci. Technol. 41, 4781–4786. doi: 10.1021/es070577h
Richter, H., Nevin, K. P., Jia, H., Lowy, D. A., Lovley, D. R., and Tender, L. M. (2009). Cyclic voltammetry of biofilms of wild type and mutant Geobacter sulfurreducens on fuel cell anodes indicates possible roles of OmcB, OmcZ, type IV pili, and protons in extracellular electron transfer. Energy Environ. Sci. 2, 506–516. doi: 10.1039/b816647a
Rimboud, M., Desmond-Le Quemener, E., Erable, B., Bouchez, T., and Bergel, A. (2015). The current provided by oxygen-reducing microbial cathodes is related to the composition of their bacterial community. Bioelectrochemistry 102, 42–49. doi: 10.1016/j.bioelechem.2014.11.006
Rismani-Yazdi, H., Carver, S. M., Christy, A. D., and Tuovinen, O. H. (2008). Cathodic limitations in microbial fuel cells: an overview. J. Power Sources 180, 683–694. doi: 10.1016/j.jpowsour.2008.02.074
Seelam, J. S., Maesara, S. A., Mohanakrishna, G., Patil, S. A., Ter Heijne, A., and Pant, D. (2018). “Resource recovery from wastes and wastewaters using bioelectrochemical systems,” in Waste Biorefinery, eds T. Bhaskar, A. Pandey, S. V. Mohan, D. J. Lee, and S. K. Khanal (Amsterdam: Elsevier), 535–570. doi: 10.1016/B978-0-444-63992-9.00018-5
Spurr, M. A., Yu, E. H., Scott, K., and Head, I. M. (2018). Extending the dynamic range of biochemical oxygen demand sensing with multi-stage microbial fuel cells. Environ. Sci. Water Res. Technol. 4, 2029–2014. doi: 10.1039/C8EW00497H
Srikanth, S., Singh, D., Vanbroekhoven, K., Pant, D., Kumar, M., Puri, S. K., et al. (2018). Electro-biocatalytic conversion of carbon dioxide to alcohols using gas diffusion electrode. Biores. Technol. 265, 45–51. doi: 10.1016/j.biortech.2018.02.058
Stumm, W., and Morgan, J. J. (1996). Aquatic Chemistry: Chemical Equilibria and Rates in Natural Waters {Environmental Science and Technology}. Hoboken, NJ: Wiley.
Ter Heijne, A., Hamelers, H. V. M., and Buisman, C. J. N. (2007). Microbial fuel cell operation with continuous biological ferrous iron oxidation of the catholyte. Environ. Sci. Technol. 41, 4130–4134. doi: 10.1021/es0702824
Ter Heijne, A., Hamelers, H. V. M., De Wilde, V., Rozendal, R. A., and Buisman, C. J. N. (2006). A bipolar membrane combined with ferric iron reduction as an efficient cathode system in microbial fuel cells. Environ. Sci. Technol. 40, 5200–5205. doi: 10.1021/es0608545
Ter Heijne, A., Liu, F., Van Rijnsoever, L. S., Saakes, M., Hamelers, H. V. M., and Buisman, C. J. N. (2011). Performance of a scaled-up microbial fuel cell with iron reduction as the cathode reaction. J. Power Sources 196, 7572–7577. doi: 10.1016/j.jpowsour.2011.04.034
Viollier, E., Inglett, P. W., Hunter, K., Roychoudhury, A. N., and Van Cappellen, P. (2000). The ferrozine method revisited: Fe (II)/Fe (III) determination in natural waters. Appl. Geochem. 15, 785–790. doi: 10.1016/S0883-2927(99)00097-9
Wang, Z. J., Zheng, Y., Xiao, Y., Wu, S., Wu, Y. C., Yang, Z. H., et al. (2013). Analysis of oxygen reduction and microbial community of air-diffusion biocathode in microbial fuel cells. Biores. Technol. 144, 74–79. doi: 10.1016/j.biortech.2013.06.093
Xia, X., Tokash, J. C., Zhang, F., Liang, P., Huang, X., and Logan, B. E. (2013). Oxygen-reducing biocathodes operating with passive oxygen transfer in microbial fuel cells. Environ. Sci. Technol. 47, 2085–2091. doi: 10.1021/es3027659
Yu, E. H., Burkitt, R., Wang, X., and Scott, K. (2012). Application of anion exchange ionomer for oxygen reduction catalysts in microbial fuel cells. Electrochem. Commun. 21, 30–35. doi: 10.1016/j.elecom.2012.05.011
Yu, E. H., Cheng, S., Logan, B. E., and Scott, K. (2009). Electrochemical reduction of oxygen with iron phthalocyanine in neutral media. J. Appl. Electrochem. 39, 705–711. doi: 10.1007/s10800-008-9712-2
Yu, E. H., Cheng, S., Scott, K., and Logan, B. (2007). Microbial fuel cell performance with non-Pt cathode catalysts. J. Power Sources 171, 275–281. doi: 10.1016/j.jpowsour.2007.07.010
Keywords: microbial fuel cell, biocathode, acidophile, gas diffusion electrode, oxygen reduction
Citation: Izadi P, Fontmorin J-M, Fernández LFL, Cheng S, Head I and Yu EH (2019) High Performing Gas Diffusion Biocathode for Microbial Fuel Cells Using Acidophilic Iron Oxidizing Bacteria. Front. Energy Res. 7:93. doi: 10.3389/fenrg.2019.00093
Received: 18 June 2019; Accepted: 21 August 2019;
Published: 10 September 2019.
Edited by:
Subba Rao Chaganti, University of Windsor, CanadaReviewed by:
Deepak Pant, Flemish Institute for Technological Research, BelgiumFeng Zhao, Institute of Urban Environment (CAS), China
Copyright © 2019 Izadi, Fontmorin, Fernández, Cheng, Head and Yu. This is an open-access article distributed under the terms of the Creative Commons Attribution License (CC BY). The use, distribution or reproduction in other forums is permitted, provided the original author(s) and the copyright owner(s) are credited and that the original publication in this journal is cited, in accordance with accepted academic practice. No use, distribution or reproduction is permitted which does not comply with these terms.
*Correspondence: Eileen H. Yu, ZWlsZWVuLnl1QG5jbC5hYy51aw==
†These authors have contributed equally to this work