- 1Copernicus Institute of Sustainable Development, Utrecht University, Utrecht, Netherlands
- 2Research Centre for Carbon Solutions, School of Engineering and Physical Sciences, Heriot Watt University, Edinburgh, United Kingdom
The concept of net-zero-CO2 power systems has gained increased attention by the EU goal to be a climate neutral continent by 2050. As potential pathways toward a net-zero-power system, this work analyzes future power systems based on intermittent renewable electricity with long-term storage through chemical energy carriers, so called Power-to-Fuel-to-Power systems, and a system based on the combustion of natural gas with 100% carbon capture and storage. The chemical energy carriers selected for electricity storage are hydrogen, methane and ammonia. Using life cycle assessment, we determine and compare the environmental impacts of 1 kWh of dispatchable electricity produced by the two pathways on seven impact categories. There was not one single pathway that had the most environmental benefits on all seven impact categories. Of the Power-to-Fuel-to-Power systems assessed the use of hydrogen for storage has the lowest environmental impact in all categories. Additionally, all the Power-to-Fuel-to-Power systems have a lower environmental impact on climate change, photochemical ozone formation and fossil resource depletion compared with the natural gas with carbon capture and storage system. The natural gas with carbon capture and storage system has a lower environmental impact on particulate matter formation, marine eutrophication and mineral resource scarcity. Our work is complemented by an analysis of pathways from a net-zero-direct-CO2 to a life-cycle net-zero-CO2-equivalent power system which is actually climate neutral, achieved by direct air capture of the residual CO2 from the atmosphere. However, this leads to an increase in all other impact categories of 11% for the Power-to-Fuel-to-Power systems and 21% in the natural gas combustion with carbon capture and storage system. A system sizing study also highlights the very low capacity factors of the capital employed for electricity storage, raising the point of economic feasibility.
Introduction
The European Green Deal states that the European Commission aims to be the first climate neutral continent by 2050 (European Commission, 2019). The transition to a (near) zero-CO2-emissions energy system is likely to depend on the availability of (i) vast amounts of emission-free electricity and (ii) the technologies and mechanisms to balance the large differences between intermittent Renewable Energy Supply (iRES) and end-user demand (Hertwich et al., 2014; Davis et al., 2018). Currently, gas and coal-fired power plants are used to balance the asynchronous production of the intermittent renewable electricity supply (iRES). In a future energy supply based on increased penetration of iRES, however, the long term (e.g., seasonal) storage of electricity is likely indispensable (Bussar et al., 2016).
Where there are many technologies proposed for short-term electricity storage, longer term (e.g., seasonal) storage of iRES is currently limited to storage in chemical energy carriers (e.g., through power-to-gas systems) and to a lesser extend pumped hydro. Pumped hydro is, however, only applicable when the topography is suitable and is best suited for storage in the order of days to weeks instead of weeks to months (Budt et al., 2016). The power-to-gas option usually starts with water electrolysis to produce hydrogen that can be stored in the subsurface directly or can react with CO2 to produce methane or other hydrocarbons, which has the advantage of being able to use the existing natural gas infrastructure. Alternatively, hydrogen can react with N2 to produce ammonia or ammonia derivatives (Grinberg Dana et al., 2016). Ammonia can be stored as a liquid at only 10 bars of pressure, which may be advantageous from the perspective of storage and energy required for pressurization. These chemical energy carriers can subsequently be used for the production of electricity, as described in Sutter et al. (2019), whence they are colloquially called Power-to-X-to-Power (P-X-P) systems.
Intermittent RES storage in both methane and ammonia would need large quantities of respectively CO2 and N2 as feedstock. As 80 percent of our atmosphere consists of N2, it is relatively easy to obtain by an air separation unit (ASU) to produce pure streams of N2 and O2. Research on capturing CO2 is mainly focused on the flue gasses from fossil fuel-fired plants as these contain high CO2 concentrations and because it could reduce greenhouse gas (GHG) emissions of these plants if the CO2 is subsequently stored (i.e., CO2 capture and storage, CCS). In addition, direct air capture (DAC) of CO2 is also being researched as a potential technology to reduce atmospheric CO2 concentration and supply CO2 (Wohland et al., 2018).
The interest for the combination of iRES with P-X-P systems is motivated by their potential to limit environmental impacts compared to existing, fossil-based electricity production systems. To that end, such systems need to have a very low carbon footprint and preferably be net-zero-CO2 (Davis et al., 2018; Sutter et al., 2019), as well as present limited other environmental burdens. An effective and comprehensive method to analyze the greenhouse gas and other environmental impacts of a technology or system is Life Cycle Assessment (LCA). Some LCAs of iRES storage are found in the literature: Oliveira et al. (2015) compared compressed air energy storage, pumped hydro, hydrogen and several types of batteries and showed that the type of electricity feedstock is decisive for the performance of storage systems. Reiter and Lindorfer (2015) and Zhang et al. (2017) did research on the global warming potential of Power-to-Gas, both for hydrogen and methane, but the conversion back to electricity was not included. Both studies showed that the system could only reduce environmental impacts if renewable electricity was used.
Research has also been done into the economics of power to gas technology as a technology to store intermittent renewable electricity, and found that it might be difficult to make profitable (Götz et al., 2016). Grinberg Dana et al. researched Power-Ammonia-Power (P-A-P) systems, but only on a technical level and did not include an environmental assessment (Grinberg Dana et al., 2016). Sutter et al. (2019) performed a first screening assessment, including the system design, efficiency, and exergy analysis of net-zero-CO2 systems for iRES storage. They included Power-to-Fuel-to-Power, as well as Power-to-Fuel-to-Propulsion and investigated hydrogen, methane, methanol and ammonia as energy carriers. They tentatively concluded that only hydrogen makes energetic sense as an electricity-derived propulsion fuel, as the other systems showed cyclic energy efficiencies as low as ten percent with limited upside for exergy improvements. Methane and ammonia were found to have potential use for iRES based Power-to-Fuel-to-Power in addition to hydrogen as they have infrastructure based advantages concerning the method of storage.
Here, we build on the work performed by Sutter et al. and other researchers, by investigating the environmental potential and trade-offs of net-zero-CO2 systems for the long term (seasonal) storage of intermittent renewable electricity with chemical energy carriers. This work limits itself to the energy carriers that were earlier found to be most interesting from an energy efficiency perspective, i.e., hydrogen, methane, and ammonia. The first objective of this paper is to design and size P-X-P systems based on hydrogen, methane and ammonia to fulfill the role of long-term iRES storage in the future European electricity market, taking into consideration (intermittent) electricity supply and demand profiles and the European Commission's 2050 goal to become a climate neutral continent. The second objective is to investigate environmental performance and trade-offs between the systems by looking at multiple environmental impacts. These trade-offs are key to understand for the selection and investment of future electricity systems in the European Union. Since the complete CO2 abatement of natural gas-fired power plants through CCS is considered another route toward zero emission electricity, this alternative is compared to the P-X-P systems to provide a point of reference.
Method
As stated above, this work aims to investigate the environmental performance of future power systems, coupling iRES with long-term electricity storage. In order to quantify this performance, we integrated two methods: simplified power systems modeling to determine P-X-P system sizes given real iRES production and projected electricity demand profiles and an attributional LCA following ISO14044 standards (ISO, 2006; Weidema, 2018). The method section follows three steps, visualized in Figure 1, in which order the research was performed. First, goal and scope are defined for the system design and the LCA including the need for a new functional unit. Second, the system design model and the Life Cycle Inventory (LCI) model are explained. Third, the approach to the dimensioning of the systems using a customized electricity supply-demand model is explained.
Goal and Scope Definition
The goal of the LCA is to investigate the environmental impacts of different coupled iRES/P-X-P systems, subject to the constraint of net-zero-CO2-emissions. This pushes the boundaries of traditional LCA, where CO2 emissions are one of the investigated stressors (outputs), rather than constraint to zero (input). The goal is furthermore to understand the environmental trade-offs between the chemical energy carriers with respect to selected environmental indicators (see section Environmental Impact Categories), and the root cause of these stressors, to allow for policy and decision making.
With respect to scope definition, the electricity market in Europe is divided in seven Wholesale Electricity Markets where electricity is traded over national borders (European Commission, 2018). In this study, Central West Europe (CWE) was chosen as the geographical focus. The CWE market constitutes Austria, Belgium, France, Germany, the Netherlands and Switzerland. The CWE was selected because it has a large interconnection capacity between the member states and it uses a more advanced method to calculate the optimal use of this capacity, called flow-based market coupling (CWE NRAs, 2015; Amprion GmbH, 2018). Besides the good interconnection, these countries are located in a geographical position where seasonal storage is expected to become of importance (see also the iRES production profiles presented in section System Dimensioning and the Storage Requirements) and therefore make a useful case study.
Function and Functional Unit
Electricity is the main output function. However, 1 kWh of electricity produced by a renewable intermittent source cannot be directly compared with 1 kWh of electricity directly delivered from a thermal power plant as is still often done. The latter can generate and dispatch electricity according to demand, by cycling the plant up and down (Heuberger et al., 2016; van Der Spek et al., 2018). Conversely, a system with intermittent renewable electricity production without any electricity storage is not demand responsive, since its production is subject to the availability of wind, sun, or other natural phenomena (wind parks are however able to reduce production, so-called curtailment). The functions under discussion are noticeably different. Thermal power plants have the function of providing electricity, but also of grid balancing and providing inertia (Brouwer et al., 2015; Mac Dowell et al., 2017). IRES only fulfills the function of electricity production. Therefore, we here propose the functional unit of 1 kWh of dispatchable electricity. We define that electricity production in a system is dispatchable if it can respond to a given demand curve. As shown in Figure 2, this implies that iRES systems need to include an electricity storage function to provide grid balancing. This storage is only used if the intermittent supply does not match the demand profile at a point in time.
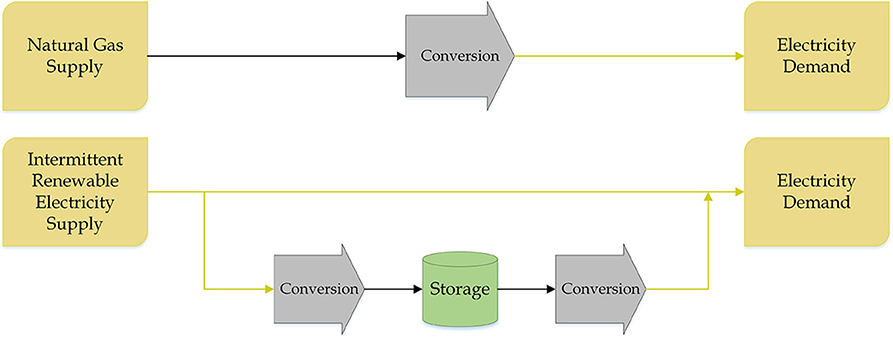
Figure 2. Simplified system designs of the natural gas-based power system (above) and the P-X-P system (below). Yellow lines indicate electricity; black lines indicate chemicals energy carriers.
Net-Zero-Direct-CO2-Emission Constraint
The systems under consideration were configured in such a way, that their direct CO2 emissions to the atmosphere were set to zero: either the system does not emit direct CO2 by nature (e.g., renewable power-to-hydrogen-to-power) or the system is expected to take up the same amount of CO2 as is emitted directly, for instance through CO2 capture from a combustion process and/or the air. The systems thus have net-zero direct CO2 emission. The net-zero-CO2 constraint was here enforced because given European and global climate ambitions (IPCC, 2014, 2018; European Commission, 2019), it is virtually certain that iRES with P-X-P is needed to achieve a net-zero-CO2 world.
Product Systems
Based on the defined function, functional unit, and the net-zero-direct-emission constraint, three P-X-P systems and two reference systems were analyzed in this study:
• Power-to-Hydrogen-to-Power (P-H-P)
• Power-to-Methane-to-Power (P-M-P)
• Power-to-Ammonia-to-Power (P-A-P)
• Future reference system: Natural gas combined cycle with carbon capture and storage complemented with direct air capture to mitigate residual direct CO2 emissions (CCS)
• Current reference system: Natural gas combined cycle (NGCC).
For the P-X-P systems, a common first step in the chemical storage of intermittent renewable electricity is the production of hydrogen through the electrolysis of water. Proton Exchange Membrane Electrolysis Cells (PEMEC) were assumed in this research as they are projected to be the dominating technology in the near-future, replacing Alkaline Electrolysis Cells (AEC) (Schmidt et al., 2017), among others due to their increased operational flexibility.
The second step is the long-term storage of hydrogen. This can be done mechanically (high pressure, cryogenic), chemically (via Methane, Methanol or Ammonia) or through physisorption (Fullerenes, Nanotubes) (Niaz et al., 2015). Here, we assumed the same chemical energy carriers for storage as earlier done in Sutter et al. (2019): compressed hydrogen (100 bar), methane and ammonia. Methanol was excluded from this research because of the lower net system efficiency compared to methane and the fact that it lacks the inherent benefit of the existing natural gas infrastructure (Sutter et al., 2019). Methanol could have the potential benefit of allowing a coupling to the chemistry sector, but sector coupling is outside the scope of this paper and was not considered here. Ammonia was here included because it is a carbon free synthetic alternative to methane and hydrogen and thereby naturally closer to the goal of a net-zero-CO2-emission system.
Two reference systems were chosen: first, a future reference system where an NGCC is fully abated by CCS, both post-combustion capture and DAC to conform to the net-zero-CO2 constraint. This reference scenario was chosen because the use of natural gas in combination with CCS is often seen as an alternative option to decarbonize electricity supply. Second, a current reference system where the dispatchable electricity was supplied by an unabated Natural Gas Combined Cycle (NGCC), to provide a status-quo benchmark.
Environmental Impact Categories
The LCA evaluated the environmental impact of energy systems in seven impact categories (Table 1) that represent relevant environmental stressors to land, air, and water, human health and resource depletion. Based on the goal of the LCA that environmental trade-offs should be understood for the net-zero technologies, the choice of impact categories should not be limited to only carbon emissions. The seven impact categories were chosen because they provide a balanced view of important resources depletion (e.g., mineral resources and fossil fuel depletion) and the environmental impact associated with the direct emissions of the fossil fuel- fired thermal power plants, such as NOx, particulate matter and SO2 (see Table 1). The life cycle impact assessment method used was the Hierarchist perspective in the ReCiPe 2016 methodology. The underlying assumptions and midpoint categorization factors can be found in Huijbregts et al. (2016).
System Design and Life Cycle Inventory Analysis
This section discusses the system designs and the key design parameters, followed by the methods applied for system dimensioning.
Future Reference System (CCS): Natural Gas Combined Cycle (NGCC) With Carbon Capture and Storage (CCS) and Direct Air Capture (DAC)
The future reference system is illustrated in Figure 3. Natural gas is supplied to an NGCC, where it is combusted to produce electricity. In Central West Europe, natural gas is supplied from different countries. The assumed NG basket is provided in the Supplementary Information.
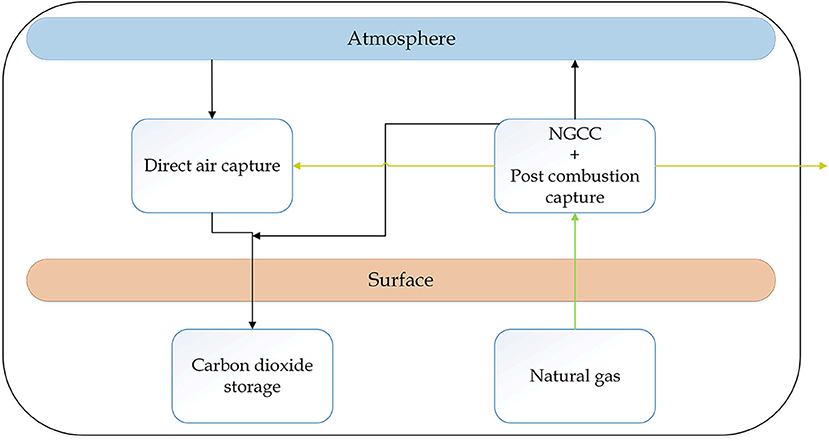
Figure 3. System diagram of the future reference system (CCS): Yellow lines represent electricity, black is CO2 and green is natural gas.
Ninety-five percent of the CO2 is captured using integrated post combustion CO2 capture, that draws off steam from the steam cycle for sorbent regeneration. The specific energy requirement for post-combustion capture shows a sharp increase if the capture rate exceeds 90% (Mletzko et al., 2016), whereas the specific energy requirements for direct air capture are constant. The ratio of post-combustion capture and DAC where total energy losses are at their minimum was found to be 95% post-combustion capture and 5% DAC respectively. The captured CO2 is compressed, transported and stored in a geological formation where it remains indefinitely if the formations are properly engineered and serviced (Bai et al., 2016). In this work, the potential leakage risk is considered to be negligible within the temporal scope of the impact assessment (100 years) and is not taken into account.
The electricity requirement for DAC, which is 0.25 kWh/kg captured CO2 (Wohland et al., 2018), can be directly obtained from the power produced within the system. The DAC heat demand, 1.75 kWh/kg CO2 captured (Wohland et al., 2018), is also obtained from the system, where we assumed that the low temperature heat (~100°C) is produced using an industrial heat pump with a Coefficient Of Performance (COP) of 2 (Arpagaus et al., 2018).
Power-to-Hydrogen-to-Power (P-H-P)
Figure 4 shows the P-H-P system where there are no direct CO2 emissions. The energy that is needed for the production and compression of hydrogen is assumed to be supplied by intermittent renewables and thus the P-H-P system only runs when there is surplus iRES. Hydrogen production was assumed by PEMEC at 30 bar (Carmo et al., 2013) with subsequent compression to 100 bar (HyUnder, 2014). Hydrogen storage is expected to be facilitated underground in a salt cavern as they are present in CWE, have high storage capacities and have high flexibility regarding injection and withdrawal (IEA, 2012; Michalski et al., 2017). Considering the fact that it is not always possible to build the electrolyser exactly on top of the underground storage location, a transport distance of 50 km was assumed between hydrogen production and storage as well as between the storage location and the Polymer Electrolyte Membrane Fuel Cell (PEMFC) facility. Hydrogen losses due to transport are 5 times higher compared to natural gas in steel pipes, but when polyethylene pipelines are used the losses are merely 0.0005–0.001% of total transported volume (Haeseldonckx and D'haeseleer, 2007). For this research a loss of 0.00075% of total transported volume was assumed.
Power-to-Methane-to-Power (P-M-P)
The P-M-P system comprises of the methane production and the consecutive methane incineration in an NGCC with CCS in order to meet the net-zero CO2 constraint (Figure 5). The technical specifications of the NGCC are identical to the NGCC described in the reference system, including the capture efficiencies, residual direct CO2 emissions are recaptured from the air using DAC. The short-term hydrogen and CO2 storage were added so that the methanation plant and DAC unit can run at a high capacity factor (thus also for the periods without iRES, see section System Dimensioning and the Storage Requirements), which is economically favorable. The produced methane was expected to use existing natural gas infrastructure for transport and storage, with an assumed distance from production to storage and storage to use of 50 km to account for the infrastructural needs and methane leakage.
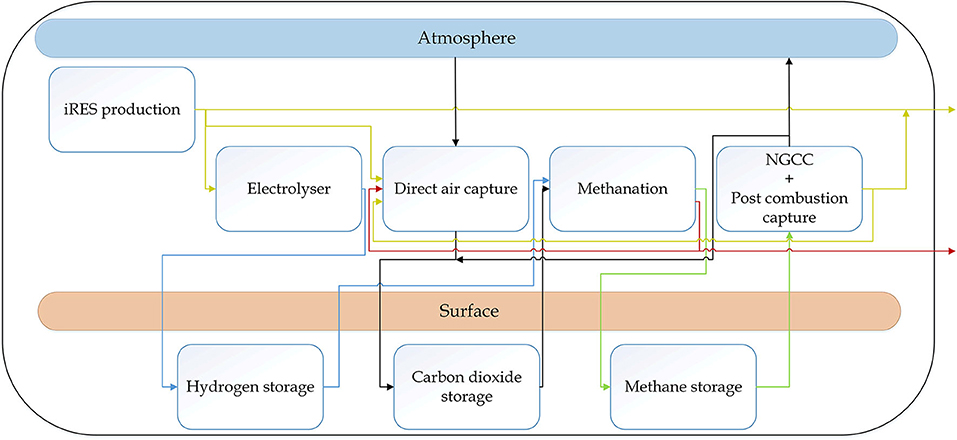
Figure 5. System diagram of the P-M-P system: Yellow lines represent electricity, blue is hydrogen, black is CO2, green is methane and red is heat.
Methane can be produced by hydrogenation of CO2 and is widely researched in literature (Hutchings, 2002; Müller et al., 2011).
Here, we assumed catalytic methanation as this is the only technology that is available on the required scale and data on this process is readily available (Götz et al., 2016). Catalytic methanation is a highly exothermic process and the excess heat, with temperatures exceeding 300°C, can be used for the heat and electricity demand of the DAC unit. If the heat exceeds the demand of the DAC unit, it can be used for other industrial purposes and was considered a useful by-product, replacing heat produced by an industrial Combined Heat and Power unit which combusts fossil fuels.
Power-to-Ammonia-to-Power (P-A-P)
Hydrogen for ammonia synthesis is nowadays produced by the reforming of natural gas. Here, however, the hydrogen is assumed to be produced by PEMEC. In contrast to the CO2 cycle that is added in the P-M-P system, the P-A-P system has a nitrogen cycle. Ammonia is produced from hydrogen and nitrogen in the reaction shown below in the Haber-Bosch process.
Ammonia synthesis needs a high temperature and pressure, although there is on-going research done on catalysts that could operate under atmospheric conditions (Vojvodic et al., 2014). A pure stream of nitrogen is required for this reaction which is produced by an ASU. In analogy to the methane system, the system in Figure 6 includes short-term hydrogen storage to increase the capacity factor and decrease the size of the ammonia production plant. Therefore, the electricity supply to the ASU and ammonia production plant have to be dispatchable and cannot fully originate from iRES. In times when iRES is limited the electricity is supplied from the ammonia combined cycle (ACC). The ammonia combined cycle is a new technology that has been researched and is expected to be technologically feasible (Institute for Sustainable Process Technology, 2017), although many uncertainties remain on its (environmental) performance (see discussion in section Combustion of Ammonia). Ammonia is stored as a liquid in tanks that are on site and under 10 bars of pressure.
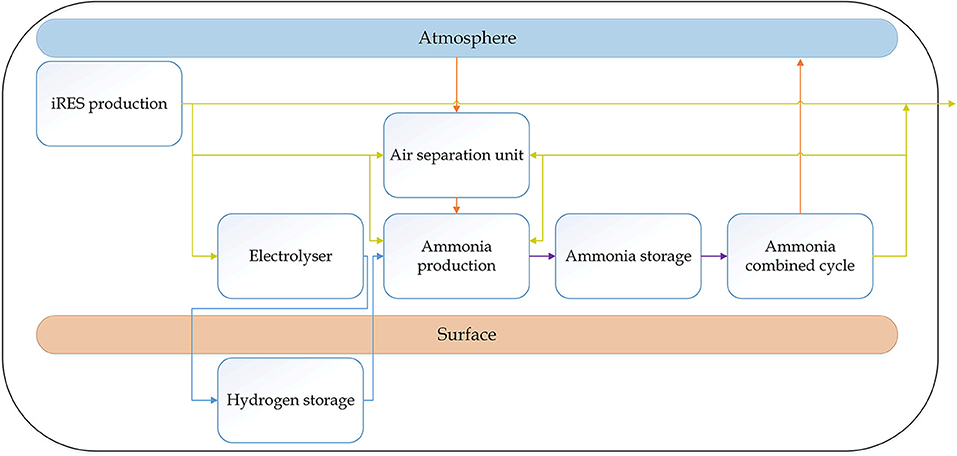
Figure 6. System diagram of the P-A-P system. Yellow lines represent electricity, blue hydrogen, orange nitrogen and purple ammonia.
Technology Performance Assumptions
The key technology performance assumptions are summarized in Table 2. The calculated mass and energy flows, the outputs of system designs step (sections System Dimensioning and System Dimensioning and the Storage Requirements), were used as the input for the life cycle inventory modeling. The detailed foreground data that was used as LCI data is presented in the Supplementary Information, the background data was obtained from the EcoInvent database v3.5.
System Dimensioning
Storage and Component Sizing Model
One of the critical parameters in our system analysis (see also section Hourly vs. daily vs. weekly data points) is the percentage of iRES that is to be stored to achieve the desired function of dispatchable electricity. Here, an optimization model was applied, using electricity supply and demand data to determine the amount of electricity storage needed and therefore the size of the different systems' components. We acknowledge this is a simplification because our systems would in reality not be stand-alone, but be part of a complex power system including many different types of electricity generators, storage options, and country interconnections (e.g., Zappa et al., 2019). This simplification, however, allows the comparative LCAs of the systems studied that we seek to undertake here.
To determine the dimensions of the different components and flows of the systems, an electricity supply-demand model was specified in Python. This model calculated, based on estimated future demand and real iRES production curves using Equation (3) (see also section Electricity Supply and Demand Data), the necessary storage capacity of the system using Equation (4) (here presented for hydrogen):
Where, Dt and St are the demand and supply of electricity at time t, Pt, Ht, and Ut are the production, storage, and use of hydrogen at time t, ηel and ηfc are the electrolyser and fuel cell efficiencies, and SF is the storage systems scaling factor.
The model minimizes the total iRES production needed to fulfill a given demand profile as a function of the storage system scaling factor. This method of minimization prevents the model from producing and using the chemical energy carrier at the same time, which in real life would be unlikely [see Equation (5)]:
Equations 6 and 7 are constraints stating that the chemical energy stored cannot become negative and that the energy stored at the end of the year equals the energy stored at the beginning. This is added so the system does not get any “free” energy, as the system starts with a certain amount of stored energy.
Electricity Supply and Demand Data
The future demand curve for the supply-demand model was taken from Zappa et al. (2019). It is based on assumptions in the EU Energy Roadmap 2050 on increased electricity demand for heating (500 TWh y−1) and transport (800 TWh y−1) in the EU (European Commission, 2012). Zappa et al. used the 2015 demand profiles for EU countries from the ENTSO-E database and added the increased demand for heating and transport (Zappa et al., 2019). Here, the demand profiles of the countries constituting Central West Europe (CWE) where added up to obtain a demand profile for the total CWE region. This means we made the simplifying assumption that there will be unlimited transmission capacity between these countries. Our systems were not expected to fulfill the demand for the complete CWE region, but were designed to have a maximum capacity of 1 GW (the size of a typical large-scale thermal power plant). Therefore, the CWE demand curve was scaled such that the maximum total supply it reached was 1 GW.
The iRES production profiles were constructed using the “Renewables.ninjas” database (Pfenninger and Staffell, 2016; Staffel and Pfenninger, 2016). For wind production, the “long-term future fleet” datasets were used; for the solar energy production, the MERRA-2 dataset was used. These datasets contain 30 years (1985–2015) of hourly values for the capacity factor of wind and solar production and are available per country. To predict the total production of wind and solar energy in CWE in 2050 these capacity factors were multiplied by the planned installed capacity in 2050 in these countries, based on the “Energy, Transport and GHG emissions Trends 2050” from the EU (European Commission, 2016). The wind and solar profiles of the CWE countries were combined to obtain the iRES supply profiles of the whole CWE. Because the used iRES supply dataset includes 30 years of weather profiles we obtained 30 different potential CWE iRES supply profiles. The system dimensions were determined such that the systems can operate in all 30 years. Both the demand and supply profiles are can be found in detail in the Supplementary Information.
Results
System Dimensioning and the Storage Requirements
Figure 7 shows the storage requirements resulting from the projected supply-demand optimization for one exemplary year. The left Figure 7A shows the modeled daily supply and demand of the P-M-P system using the 2015 meteorological data and the required dispatch (in GW) of the electrolyser and the NGCC. The system's maximum hourly consumer demand was set to 1 GW (see section Electricity Supply and Demand Data). Because the hourly data points were aggregated into daily points, the maximum of the daily averaged data is lower than 1 GW (763 MW). Figure 7A also shows that for the P-M-P system, as expected, the electrolyser is operated more in the summer months, whereas the NGCC delivers more electricity in the winter months. This is reflected in the fluctuations of the total amount of energy storage required. Figure 7B shows that the systems indeed provide seasonal electricity storage, as the stored amounts of energy carriers dip before, and peak after the summer. Exemplary profiles for the P-H-P and P-A-P systems can be found in the Supplementary Information.
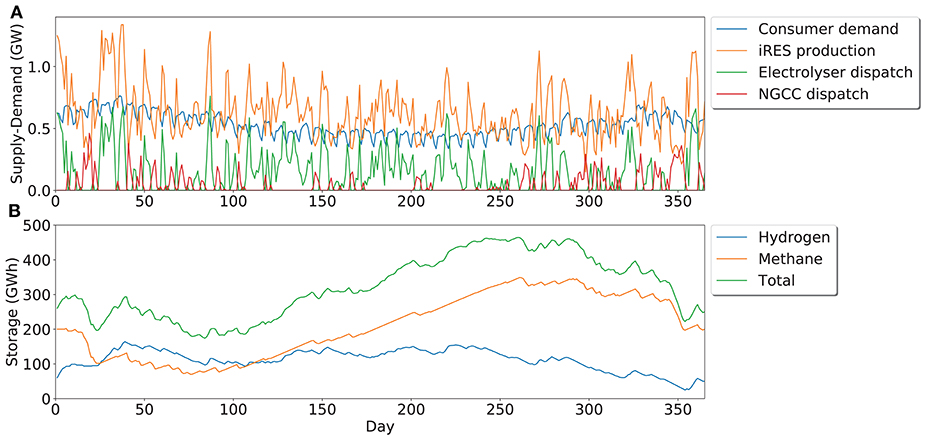
Figure 7. (A) Daily fluctuations in the projected demand and supply for the P-M-P system (shown as lines “Consumer demand” and “iRES production”) using 2015 meteorological data, and the resulting dispatch capacity required for the electrolyser and NGCC (GW). (B) Hydrogen and methane storage requirements (GWh) and the two curves combined. Day 1 as January first. The small peak in hydrogen production around day 45 is represents a peak in wind power production in February 2015.
Table 3 shows the results of the key design parameters based on the required electricity storage capacity for all three P-X-P systems. The capacity factors of the electrolyser are around 17% for all three systems, where the capacity factors of the power plants and fuel cell are around 8%. These low capacity factors show that a large part of the electrolyser capacity remains unused during the year, which may have implications for economic feasibility. Although economics are outside the scope of this paper, it is worth noting that PEMECs are expected to have a capital cost between 250 and 1270 €/kW in 2030 (FCH JU, 2015) and that such low capacity factors would imply inefficient and therefore expensive use of installed capital. Similarly, construction of a power plant that operates <10% of the time may be expected to be unprofitable (unless the power plant has surpassed its economic lifetime, or if it would be possible to use the remaining capacity of the NGCC for the combustion of natural gas with CCS, net-zero hydrogen or ammonia).
In both the P-M-P and P-A-P systems there is still the need for a relatively sizeable hydrogen storage of 330–350 GWh. This results from the assumption of at least 90% capacity factors of the methane and ammonia production plants (which was made taking into account the economic feasibility of methanation and ammonia production). If this constraint is deleted, the hydrogen storage size would greatly reduce. However, this would, in turn, lead to an increase in the size and decrease in the capacity factor of the methane and ammonia production, indicating a trade-off in system design where a full economic analysis would be required to determine a potential optimum.
For the P-M-P system, if the 2015 weather data is used, the methane storage fluctuates between 50 GWh and 350 GWh (see Figure 7). However, the methane storage needed for the P-M-P system to function over the complete 30 years of weather data in Table 3 is 859 GWh. This shows that there is a large difference in storage capacity requirement over the years and that this strongly depends on the production profile of the renewable electricity.
For the P-X-P systems, the total required storage is ~1.1 TWh for the dispatchable capacity of 1 GW. With the maximum electricity demand in the CWE region in 2050 predicted to be 385 GW (Zappa et al., 2019), 424 TWh of electricity storage is needed to deliver all electricity in the CWE region in 2050. To put this into perspective, there is currently around 640 TWh of natural gas storage in the CWE region (Gas Infrastructure Europe, 2018). If natural gas usage declines in the coming decades and if the geology allows it, this excess storage capacity could be used for hydrogen or synthetic methane instead (DBI-GUT, 2017).
Finally, the amount of electricity that goes through storage will influence the technical and environmental performance of the systems. If a higher percentage of renewable electricity needs to be stored, it will decrease the overall system efficiency due to the energy losses of storage. Consequently, the required sizes of the different components will also be affected. This point is further discussed in section Data Uncertainty.
Results of Environmental Life Cycle Assessment
The system sizing is fed into the LCA, here presented in the following three subsections. First, the overall comparison of the environmental impacts is addressed to allow discussing the environmental trade-offs between the investigated P-X-P systems (with net zero direct emissions) and the two reference systems (i.e., NGCC and fully abated NGCC). Second, each impact category will be interpreted in detail. Last, the environmental impacts are interpreted based on the geographical scopes of the value chain.
Overview Comparison of Environmental Impacts
Figure 8 shows the life cycle environmental performance of the five electricity supply systems studied. Comparing the two NGCC reference systems, the figure shows that fully abating the direct CO2 emissions of the NGCC system (“CCS” in Figure 8) reduces its life cycle climate change (CC) impact by around 70% compared to the unabated NGCC system, but it leads to an increased impact on all other impact categories. The 70% of climate change reduction is in the range which was found in earlier studies by Corsten et al. (2013) and Singh et al. (2011), where CCS implementation to an NGCC decreased CO2-equivalent emissions by 60–80% while increasing all other impacts. Note that the CCS system in this study assumed a higher CO2 capture rate (95%) at the NGCC compared to the systems analyzed by Corsten and Singh (90% capture rate); also, in our study DAC was assumed to reduce the remaining 5% of emissions in the foreground. The noticeable increase (~25%) in fossil resource scarcity is largely due to the reduced efficiency of the NGCC and thus the increased use of natural gas, which was also observed by the aforementioned authors.
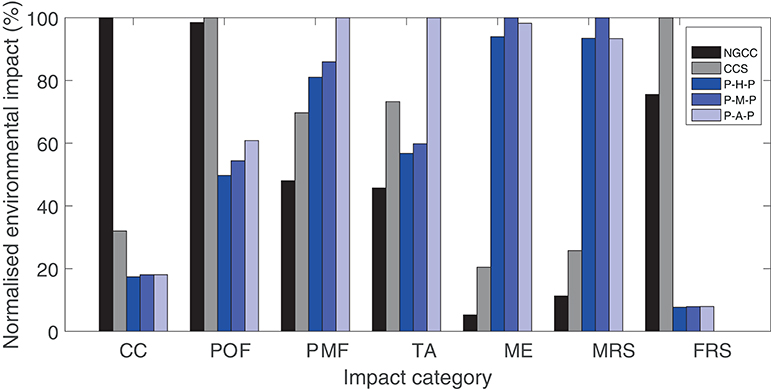
Figure 8. Comparison of cradle-to-factory gate environmental impact of 1 kWh dispatchable electricity for the studied cases on seven selected impact categories. Results normalized to the system with the highest impact (set to be 100%). CC, Climate Change; POF, Photochemical Ozone Formation; PMF, Particulate Matter Formation; TA, Terrestrial Acidification; ME, Marine Eutrophication; MRS, Mineral Resource Scarcity; FRS, Fossil Resource Scarcity.
Compared to the two NGCC systems, the P-X-P systems offer substantial environmental impact reductions in three categories out of seven, namely, 80% reduction in climate change (CC), 40–50% reduction in photochemical ozone formation (POF) and 90% reduction in fossil resources scarcity (FRS). However, the P-X-P systems have higher impacts on PM formation (PMF), marine eutrophication (ME) and mineral resource scarcity (MRS), than the NGCC systems. For terrestrial acidification (TA), the NGCC case has the lowest impact whereas the P-A-P system has the highest impact. The detailed interpretation of each impact category will be provided in the following.
Breakdown by Key Processes
Figure 9 shows the breakdown of the LCA results by key processes for each environmental impact category. The natural gas input includes the extraction and transport of natural gas. The renewable electricity process includes the production and installation of PV and wind capacity. Infrastructure therefore does not include PV and wind capacity but only the NGCC, ACC, fuel cell, electrolyser, and storage assets.
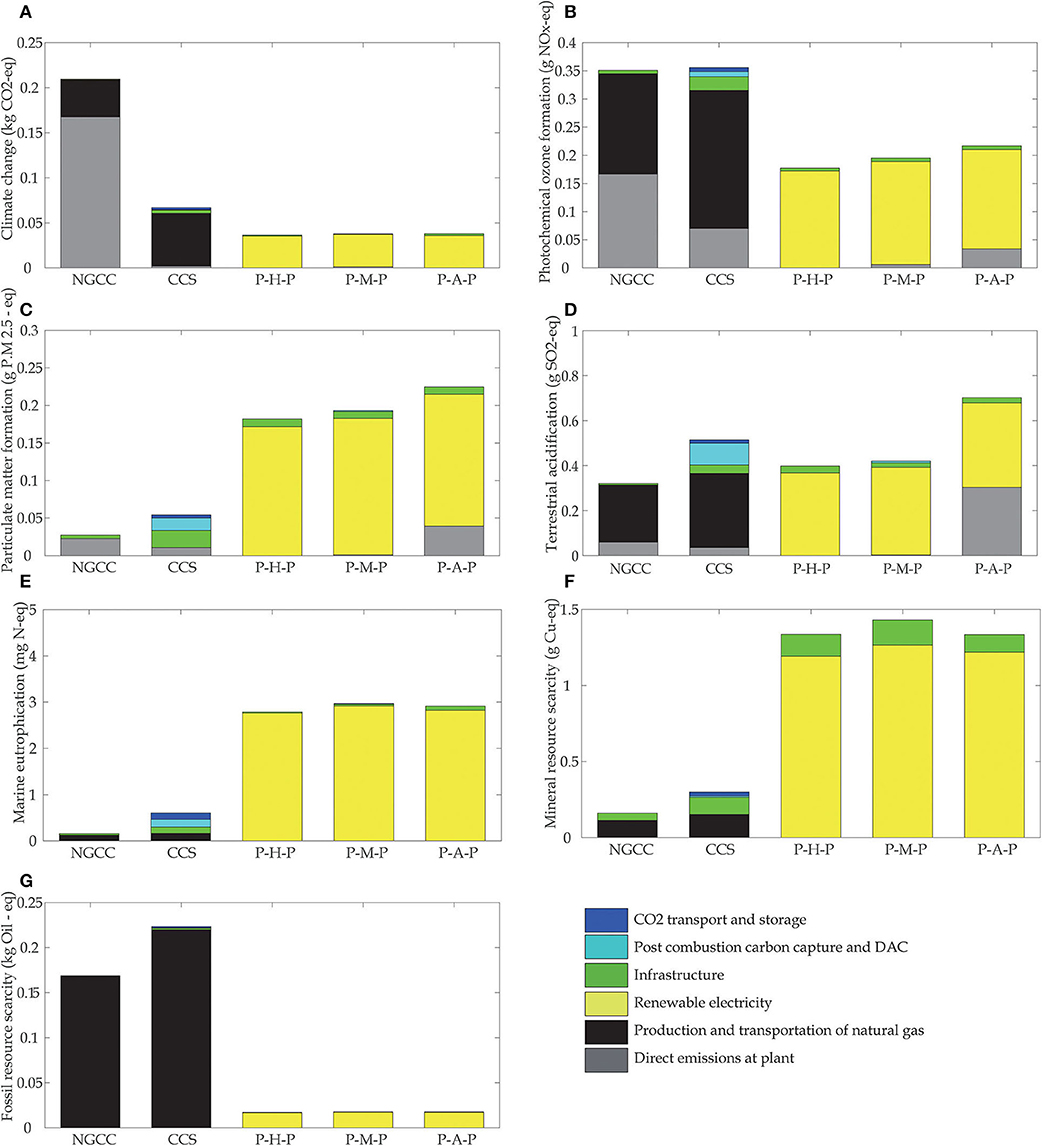
Figure 9. Cradle-to-factory gate impacts of 1 kWh dispatchable electricity of the five studied product systems, breakdown by key processes. (A) Climate change, (B) Photochemical ozone formation, (C) Particulate matter formation, (D) Terrestrial acidification, (E) Marine eutrophication, (F) Mineral resource scarcity, and (G) Fossil resource scarcity.
Impacts resulted from the emissions from the fuel input, being either natural gas or renewable electricity, dominate the environmental impact in the systems. The current reference NGCC system is the exception where the direct emissions are responsible for the largest share (80%) of the CC (see Figure 9A), 91% of PMF (Figure 9C) and 47% of the POF (Figure 9B).
The direct emissions at the plant also contribute a significant fraction to the impacts of POF (16%) and PMF (18%) for the P-A-P system due to unburned ammonia and large quantities of NOx in the flue gas (See Figures SI4, SI5). Improving the combustion rate of ammonia could lower this high impact, but the full combustion of ammonia is known to be challenging due to its low flame speed (Institute for Sustainable Process Technology, 2017).
The direct emission of ammonia at plant in the P-A-P system is also responsible for 43% of the terrestrial acidification impact (see Figure S16), because ammonia is a strong acidification gas with a characterization factor of 1.96 kg SO2 eq./kg ammonia. The unburned ammonia and the rate of NOx emissions at ammonia combustion are however a source of large uncertainty, and they are therefore further addressed in section Combustion of Ammonia.
By adding CCS and DAC to the NGCC the climate change (Figure 9A) impact decreases by 70%, The CC impact of CCS system is dominated by the background processes, mainly originated from the production of natural gas. Due to the decreased efficiency of the NGCC with CCS, the natural gas input per kWh produced, and with it the methane emissions from natural gas production and transport losses (fugitive emissions), increases. This increase in methane emissions therefore slightly negates the positive effects of the CO2 capture and storage.
For the three P-X-P systems, the impacts from the production of renewable electricity dominate (89–98%) in all seven categories. The impacts are associated with the manufacturing of PV panels and wind turbines for which aluminum and copper are mined and the manufacturing processes use fossil-fuel based energy. For instance, for climate change, 47% of the impact is caused by coal-based electricity; for fossil resources scarcity, 40–45% of the impact is caused by hard coal, 25–30% is caused by natural gas and ~20% is caused by crude oil.
For the two reference systems, the impacts from natural gas production are mainly contributed by the fugitive methane emissions during long-distant pipe transportation, electricity needed for compression and emissions during natural gas extraction.
The impact of other infrastructure (electrolyser, storage of fuel, fuel cell and natural gas/ammonia combustion facility) of the P-X-P systems is relatively low. It only contributes 1–5% of the life cycle impact, with the exception of the mineral resource scarcity impact (9–12%). Infrastructure impact is highest in the CCS system, mainly due to the CO2 pipeline and storage. A breakdown by substance for all impact categories can be found in the Supplementary Information.
Geographical Origin of the Environmental Impacts
One of the key findings based on the breakdown results is that the environmental impacts of the net-zero electricity systems are strongly associated with mining of aluminum and copper, the manufacturing of PV panels and wind turbines, and the extraction and transport of natural gas for the NGCC-based systems. If the EU wants to minimize the impacts of these net-zero electricity systems it is important to pay attention to these environmental hotspots occurring outside of the EU.
Figure 10 shows the geographical origin of the environmental impacts of the two net-zero electricity systems: CCS and P-M-P. The other two P-X-P systems resemble much to P-M-P systems in terms the geographical distribution of the impacts.
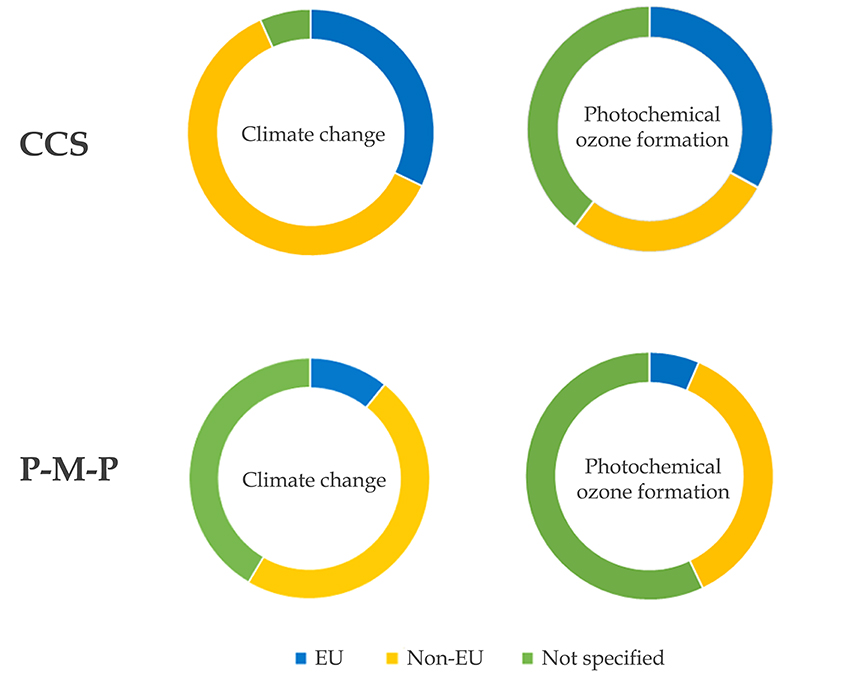
Figure 10. Geographical origin of climate change impact and photochemical ozone formation impact for selected systems.
In the CCS system, the climate change impact resides for more than 60% outside of the EU borders, mainly due to natural gas imported from Russia (45% of total). The P-M-P system has a larger uncertainty in the geography than the CCS system as more of the impact has an unknown location, but still more than 48% of the climate change impact resides outside of the EU borders, as most PV panels today are manufactured in China. For photochemical ozone formation we see a similar trend, where the P-M-P system has at least 36% of the impact originated outside of EU borders.
Discussion
Hourly vs. Daily vs. Weekly Data Points
In the modeling approach it was chosen to aggregate the hourly supply and demand data into daily data points. Thereby, the P-X-P systems do not provide to balance intraday fluctuations but rather fulfill their long-term storage role. The share of the electricity demand that is provided by the storage was 8–9% in contrast to 12–13% if the hourly data points would have been used. Rodriquez et al. found that if 100% renewable production in Europe, assuming unlimited interconnection and using hourly data, almost 15% of demand needs balancing (Rodríguez et al., 2014).
To focus even more on seasonal energy storage, weekly aggregated data points could have been modeled as well, which would have resulted in only 4.5–5.5% of electricity demand passing through storage. As a consequence, less iRES passing through storage would further reduce the climate change impact of the P-X-P systems by 7–8% (see also the sensitivity analysis in section Data Uncertainty). However, it would also reduce the capacity factor (e.g., to ~4 and 2%) of the electrolyser and fuel cell/NGCC/ACC, making the system even less economically attractive. This highlights a difficult trade-off between the total environmental impact and the economic feasibility for chemical energy storage used for net-zero electricity supply.
Data Uncertainty
A one-way sensitivity analysis was conducted to identify the sensitive system parameters and assumptions. The results are shown in Figure 11 for the four net-zero electricity supply systems for climate change. Other impact categories largely resemble climate change as most environmental impacts are concentrated in the renewable electricity supply and natural gas supply.
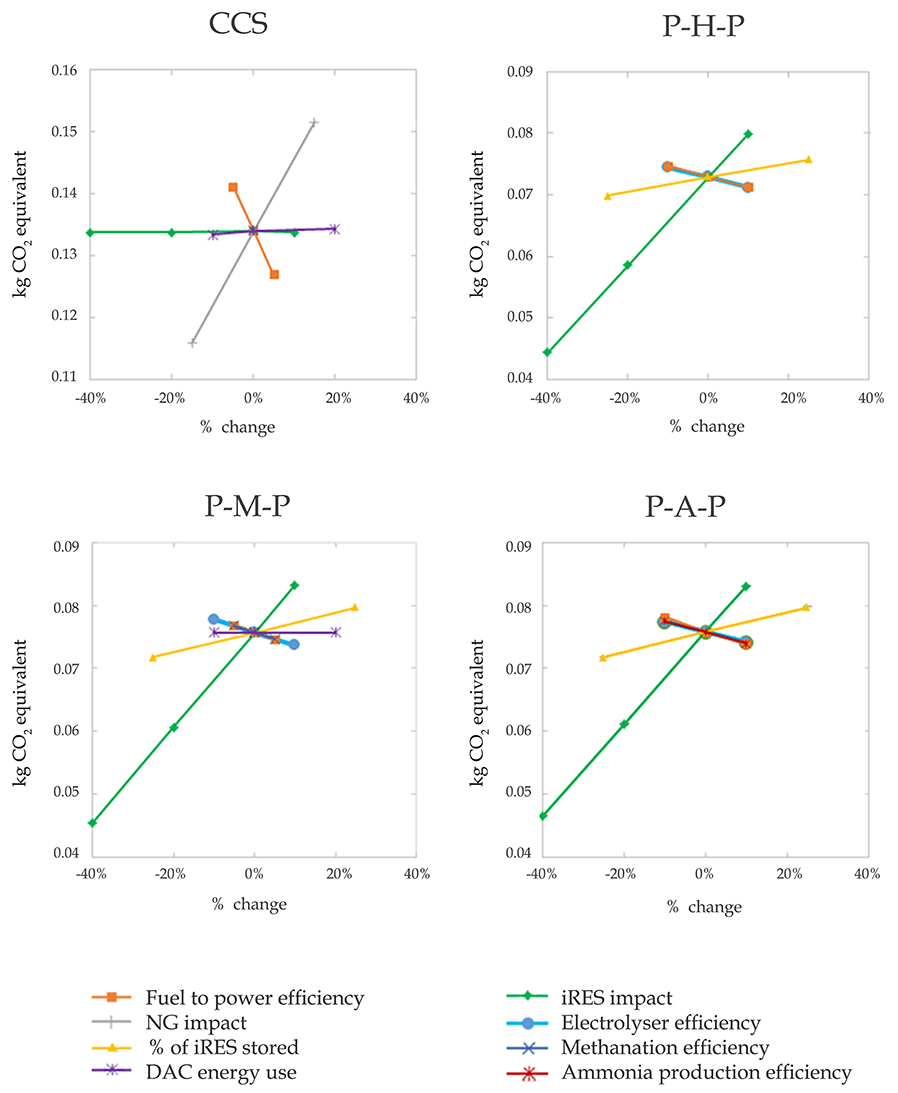
Figure 11. Sensitivity analysis of climate change impact for the CCS, P-H-P, P-M-P, and P-A-P systems to several system parameters.
The CCS system is the most sensitive to changes related to the natural gas supply and consumption, given that the natural gas supply chain is the largest source of GHG emissions in the background system. The uncertainties lie mainly in the fugitive methane emissions that take place during production and transport, and in the efficiency with which natural gas is converted to power in the NGCC. For the supply chain, the origin of the gas plays an important role. There is a large potential to improve, since for example Russian gas is known to lose 0.23% per 1,000 km during transport (Dones et al., 2005). The efficiency of gas turbines is also a sensitive parameter for the CCS system (see Figure 11). However, the NGCC is a mature technology and it is not expected that the efficiency of gas turbine will substantially improve (i.e., more than single percentage points) unless breakthrough technologies reach the market.
The P-X-P systems are most sensitive to changes in the environmental burden of the renewable electricity input (iRES impact), as also evident by the breakdown results that renewable electricity production is the most important contributor (section Breakdown by Key Processes). Interestingly, an increase in the “percentage of iRES stored” increases the climate change impact. This is expected as the storage system increases the renewable electricity requirement because of the increased energy losses in the conversion processes and the need for more infrastructure. It is therefore key to limit the need for electricity storage to limit the life cycle impacts, if possible, e.g., by means of demand side management.
Combustion of Ammonia
One further uncertainty that was highlighted in the results is the large scale combustion of ammonia for energy purposes (see Section Breakdown by Key Processes). This is a concept recently received much attention but not yet extensively researched (Institute for Sustainable Process Technology, 2017; Yapicioglu and Dincer, 2019). Many key technical and environmental performances of ammonia combustion such as the direct emissions of NOx and unburned ammonia are still uncertain. Estimates for NOx emissions in flue gas from ammonia combustion range from 20 to 2,300 ppm, depending on various parameters such as equivalence ratio's1, inlet temperatures, fuel additives and combustion pressures (Nozari and Karabeyoǧlu, 2015; Valera-Medina et al., 2018). We here chose a value of 300 ppm NOx, six times higher than in a natural gas combined cycle, because it is expected that the different burning characteristics of ammonia will always lead to relatively high NOx emissions (Nozari and Karabeyoǧlu, 2015). For the amount of unburned ammonia we chose 30ppm, which is based on research from Kobayashi et al. (2019) and Kurata et al. (2019). Many studies about ammonia combustion do not report on unburned ammonia emissions at all (Valera-Medina et al. and Nozari et al.).
Background Inventory for Future Production Systems
This paper analyzed future energy supply systems and used predictions of future electricity demand, renewable electricity supply and technological parameters. However, the database used for the background inventory, EcoInvent database version 3.5, is based on the current average technology level The impacts from the background inventories are expected to reduce in the future on the path of our energy transition toward more sustainable resources. The CO2 emissions associated with PV panel production and natural gas supply will hopefully change for the better as we move to a low carbon world. To analyze a future energy system by using dynamic projections of life cycle inventory data is outside the scope of this research.
Net-Zero Direct CO2 Emissions vs. Net-Zero Life Cycle Greenhouse Gas Emissions
In the above analysis, we looked at mitigating the direct CO2 emissions of electricity production and supply systems. However, we also showed that even in the case of net-zero-direct-CO2-emissions, there were still substantial GHG emissions in the background of our systems. Figure 10 also shows that many of these background emissions stem from outside the region of study (in this case Central West Europe). This raises the question of what net-zero really means, which also relates to the question of stewardship. That is, if a country or region wants to reach net-zero at a given moment in time, should it then also compensate for indirect emissions, and should it also compensate for emissions outside its own region, but attributable to its power supply system? Given the nature of this contribution, we will not speculate on the political or legal aspects of such questions, but we can show how the full value chain could be made climate neutral over its life cycle, and what this implies for other environmental impacts.
To completely rid our electricity production systems of all indirect CO2-eq. emissions, an immense amount of supply chain improvements would need to be realized. In the CCS system, it would be necessary to completely eliminate methane losses during transport and natural gas production and use renewable electricity to power compression stations in both the EU as Russia. In the P-X-P systems, as materials are increasingly imported from outside of the EU, mainly China, the effort of the replacement of coal power plants with wind and PV in China becomes an essential measure in order to achieve a true net zero-emission of our electricity system. However, it would be difficult for the EU to implement or enforce such energy transition strategy outside the EU (other than through global multilateral agreements following the Paris Agreement).
Alternatively, a country or region could compensate for the emissions of its energy system outside its geographic boundaries. In the spirit of this work, one option would be to use DAC to negate all CO2-eq. emissions left in the system. Naturally, the other environmental impacts will then further increase, because the additional DAC also needs additional energy (thus PV and wind). Figure 12 shows the increase in environmental impacts if we increase DAC and CO2 storage to have a system with zero life cycle GHG emissions. The energy required for DAC was expected to come from within the system itself. The increase of other environmental impacts for the unabated NGCC system is the highest among the five systems studied, with an average increase of 112% (Figure 11). This is due to the fact that it had the highest GHG emissions in the original system, so a higher DAC capacity is needed to negate the remaining emissions. The other impacts of the CCS system increase on average by 21% and the P-X-P systems with 11% (meaning there is less environmental burden of making the P-X-P systems net-zero GHG over their life cycle).
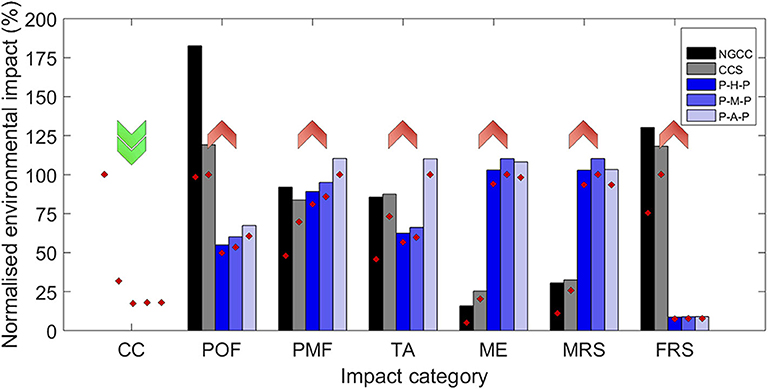
Figure 12. Change in environmental impact per kWh dispatchable electricity if DAC is also used to fully negate the GHG emissions of background processes. The bars show the increased environmental impacts of the zero GHG emission systems; the diamonds give original values from the net-zero-direct-CO2-emissions systems in Figure 8. Arrows show whether there is an increase or decrease of the impact compared to the case where only the foreground emissions were abated. A value of 100% represents the system with the highest environmental impact on that impact category.
There is a positive feedback loop when the GHG impact of the renewable supply is decreased, because reducing emissions in our system leads to cleaner electricity which improves our system again. This loop is taken into account and thus less direct air capture is needed compared with the situation were the background emissions of the electricity supply would have been kept stable. Battling climate change through the capture of CO2 with DAC will be technologically possible. However, implementing it on the scale that would be necessary to completely negate the remaining GHG emissions will require a massive deployment of this technology and introduce an increase in other environmental impact categories. In the P-X-P systems this increase on non-climate change categories is up to 11%, whereas for the CCS system impact increases by 21%.
Conclusions
This study addressed the system design, sizing and life-cycle assessment of three power-to-X-to-power systems for chemical energy storage of intermitted renewable electricity. Hydrogen, methane and ammonia were assessed as potential energy carriers and all systems were designed to adhere to the net-zero-direct-CO2-emission constraint. The LCA comparison between the P-X-P systems and two reference CCS systems (one fully abated, one unabated) on seven impact categories showed there was not a single clear winner as several trade-offs were found between them. Net-zero-direct-CO2 P-X-P systems result in a climate change impact that is up to 50% lower than the fully abated NGCC system and has lower impacts on both photochemical ozone formation and fossil resource scarcity. The fully abated NGCC system outperformed the P-X-P systems on marine eutrophication and mineral resource scarcity where their results were comparable for particulate matter formation. Among the three P-X-P systems assessed the hydrogen system has the lowest environmental impact in all categories due to its higher round trip efficiency.
This publication has focused on the environmental impacts of net-zero CO2 energy systems. A full economic performance was not undertaken. The system design, however, indicates that the very low capacity factors for both electrolyser and the re-electrification modules are reason to believe that the economic attractiveness of the P-X-P systems as such might be limited. The same hurdle was found in the research by Götz et al. (2016), where it is shown that synthetic natural gas cannot be expected to compete with natural gas prices even when the electricity cost is 0 ct/kWh. In real power systems the P-X-P systems might be able to collaborate with different systems or modes of operation, thereby increasing the capacity factor and economics. An example of this could be cross-sector coupling of the energy and chemicals sector through methanol, ammonia, or another synthetically produced molecule.
Nevertheless, a system without climate change impact in its entire life cycle, including the background, was proposed and assessed. This was achieved by capturing an equal amount of CO2 from the atmosphere in order to cancel out any background emissions that are still present in the net-zero-direct-CO2 systems. If the systems are equipped with enough direct air capture to do this, it would logically lead to an increase in the other impact categories. This demonstrates the importance of using multiple indicators in (environmental) decision making, as it can show drawbacks of technologies otherwise overlooked. In this research we show that P-X-P systems can have a place in future zero carbon energy systems, but that it also has its trade-offs and hurdles to overcome. These trade-offs are only visible if decision making is not only focused on CO2 emissions but also takes other impacts into account.
Data Availability Statement
All datasets generated for this study are included in the article/Supplementary Material.
Author Contributions
The authors together conceived the original idea. JW undertook the quantitative analysis under guidance of MS and LS. All authors contributed to authoring the manuscript and agree to its final content.
Conflict of Interest
The authors declare that the research was conducted in the absence of any commercial or financial relationships that could be construed as a potential conflict of interest.
Acknowledgments
This work was undertaken without external funding. JW would like to express his gratitude to Xiaojin Zhang and Christian Bauer for helping to set up the life cycle inventory for water electrolysis and methane synthesis and for their very helpful advice.
Supplementary Material
The Supplementary Material for this article can be found online at: https://www.frontiersin.org/articles/10.3389/fenrg.2020.00104/full#supplementary-material
Abbreviations
P-X-P, Power-to-Fuel-to-Power systems; P-H-P, Power-to-Hydrogen-to-Power system; P-M-P, Power-to-Methane-to-Power system; P-A-P, Power-to-Ammonia-to-Power system; NGCC, Natural Gas Combined Cycle; CCS, Carbon Capture and Storage (system); DAC, Direct Air Capture of CO2; ACC, Ammonia Combined Cycle; LCA, Life Cycle Assessment; GHG, Green House Gasses; iRES, intermittent Renewable Electricity Supply; CWE, Central West Europe; ASU, Air Separation Unit; PEMFC, Polymer Electrolyte Membrane Fuel Cell; PEMEC, Polymer Electrolyte Membrane Electrolysis Cell.
Footnotes
1. ^Equivalence ratio: the ratio of fuel vs. oxidizer. An equivalence ratio > 1 means that the blend has more fuel than can be burned based on the stoichiometric ratio.
References
Arpagaus, C., Bless, F., Uhlmann, M., Schiffmann, J., and Bertsch, S. S. (2018). High temperature heat pumps: market overview, state of the art, research status, refrigerants, and application potentials. Energy 152, 985–1010. doi: 10.1016/j.energy.2018.03.166
Bai, M., Zhang, Z., and Fu, X. (2016). A review on well integrity issues for CO2 geological storage and enhanced gas recovery. Renew. Sustain. Energy Rev. 59, 920–926. doi: 10.1016/j.rser.2016.01.043
Brouwer, A. S., van den Broek, M., Seebregts, A., and Faaij, A. (2015). Operational flexibility and economics of power plants in future low-carbon power systems. Appl. Energy 156, 107–128. doi: 10.1016/j.apenergy.2015.06.065
Budt, M., Wolf, D., Span, R., and Yan, J. (2016). A review on compressed air energy storage: basic principles, past milestones and recent developments. Appl. Energy 170, 250–268. doi: 10.1016/j.apenergy.2016.02.108
Bussar, C., Stöcker, P., Cai, Z., Moraes, L. Jr., Magnor, D., Wiernes, P., et al. (2016). Large-scale integration of renewable energies and impact on storage demand in a European renewable power system of 2050—sensitivity study. J. Energy Storage 6, 1–10. doi: 10.1016/j.est.2016.02.004
Carmo, M., Fritz, D. L., Mergel, J., and Stolten, D. (2013). A comprehensive review on PEM water electrolysis. Int. J. Hydrogen Energy 38, 4901–4934. doi: 10.1016/j.ijhydene.2013.01.151
Corsten, M., Ramírez, A., Shen, L., Koornneef, J., and Faaij, A. (2013). Environmental impact assessment of CCS chains - Lessons learned and limitations from LCA literature. Int. J. Greenhouse Gas Control. 13, 59–71. doi: 10.1016/j.ijggc.2012.12.003
Davis, S. J., Lewis, N. S., Shaner, M., Aggarwal, S., Arent, D., Azevedo, I. L., et al. (2018). Net-zero emissions energy systems. Science 360:9793. doi: 10.1126/science.aas9793
DBI-GUT (2017). The Effects of Hydrogen Injection in Natural Gas Networks for the Dutch Underground Storages, 74. Retrieved from at: https://www.rvo.nl/sites/default/files/2017/07/The%20effects%20of%20hydrogen%20injection%20in%20natural%20gas%20networks%20for%20the%20Dutch%20underground%20storages.pdf (accessed May 27, 2020).
Dones, R., Heck, T., Emmenegger, M. F., and Jungbluth, N. (2005). Life cycle inventories for the nuclear and natural gas energy systems, and examples of uncertainty analysis. Int. J. Life Cycle Assess 10, 10–23. doi: 10.1065/lca2004.12.181.2
European Commission (2012). Energy Roadmap 2050? Luxembourg: Publications Office of the European Union.
European Commission (2016). EU Reference Scenario 2016 - Energy, Transport and GHG Emissions - Trends to 2050. Energy, Transport and GHG Emissions - Trends to 2050. doi: 10.2833/9127
European Commission (2018). European Commission Quarterly Report on European Electricity Markets. European Commission.
European Commission (2019). The European Green Deal. European Commission. doi: 10.1017/CBO9781107415324.004
Gas Infrastructure Europe (2018). NG Storage Database. Available online at: https://www.gie.eu/index.php/gie-publications/databases/storage-database (accessed on December 15, 2019).
Götz, M., Lefebvre, J., Mörs, F., McDaniel Koch, A., Graf, F., Bajohr, S., et al. (2016). Renewable power-to-gas: a technological and economic review. Renew. Energy 85, 1371–1390. doi: 10.1016/j.renene.2015.07.066
Grinberg Dana, A., Elishav, O., Bardow, A., Shter, G. E., and Grader, G. S. (2016). Nitrogen-based fuels: a power-to-fuel-to-power analysis. Angew. Chem. Int. Ed. 55, 8798–8805. doi: 10.1002/anie.201510618
Haeseldonckx, D., and D'haeseleer, W. (2007). The use of the natural-gas pipeline infrastructure for hydrogen transport in a changing market structure. Int. J. Hydrogen Energy 32, 1381–1386. doi: 10.1016/j.ijhydene.2006.10.018
Hertwich, E. G., Gibon, T., Bouman, E. A., Arvesen, A., Suh, S., Heath, G. A., et al. (2014). Integrated life-cycle assessment of electricity-supply scenarios confirms global environmental benefit of low-carbon technologies. Proc. Natl. Acad. Sci. U.S.A. 112, 6277–6282. doi: 10.1073/pnas.1312753111
Heuberger, C. F., Staffell, I., Shah, N., and Mac Dowell, N. (2016). Quantifying the value of CCS for the future electricity system. Energy Environ. Sci. 9, 2497–2510. doi: 10.1039/C6EE01120A
Huijbregts, M. A. J., Steinmann, Z. J. N., Elshout, P. M. F., Stam, G., Verones, F., Vieira, M. D. M., et al. (2016). ReCiPe 2016: A harmonized life cycle impact assessment method at midpoint and endpoint level - Report 1 : characterization. National Institute for Public Health and the Environment. doi: 10.1007/s11367-016-1246-y
Hutchings, G. J. (2002). Methanation of carbon dioxide. Appl. Catal. A Gen. 84, 103–190. doi: 10.1016/0926-860X(92)80119-W
HyUnder (2014). Assessment of the Potential, the Actors and Relevant Business Cases for Large Scale and Seasonal Storage of Renewable Electricity by Hyrdogen Undrground Storage in Europe. HyUnder.
Institute for Sustainable Process Technology (2017). Power to Ammonia Report. Institute for Sustainable Process Technology. Available online at: https://ispt.eu/media/DR-20-09-Power-to-Ammonia-2017-publication.pdf (accessed May 27, 2020)
IPCC (2014). “Summary for policy makers,” in Climate Change 2014: Synthesis Report. Contribution of Working Groups I, II and III to the Fifth Assessment Report of the Intergovernmental Panel on Climate Change, eds R. K. Core Writing Team Pachauri and L. A. Meyer (Geneva: IPCC), 151.
IPCC (2018). “Summary for policymakers,” in Global Warming of 1.5°C. An IPCC Special Report on the Impacts of Global Warming of 1.5°C Above Pre-Industrial Levels and Related Global Greenhouse Gas Emission Pathways, in the Context of Strengthening the Global Response to the Threat of Climate Change, eds T. W. V. Masson-Delmotte et al. (Geneva:IPCC), 32.
ISO (2006). LCA Standards. Available online at: https://www.iso.org/obp/ui/#iso:std:iso:14044:ed-1:v1:en (accessed May 27, 2020).
Kobayashi, H., Hayakawa, A., Somarathne, K. D. K. A., and Okafor, E. C. (2019). Science and technology of ammonia combustion. Proc. Combust. Inst. 37, 109–133. doi: 10.1016/j.proci.2018.09.029
Kurata, O., Iki, N., Inoue, T., Matsunuma, T., Tsujimura, T., Furutani, H., et al. (2019). Development of a wide range-operable, rich-lean low-NOx combustor for NH3 fuel gas-turbine power generation. Proc. Combust. Inst. 37, 4587–4595. doi: 10.1016/j.proci.2018.09.012
Mac Dowell, N., Fennell, P. S., Shah, N., and Maitland, G. C. (2017). The role of CO2 capture and utilization in mitigating climate change. Nat. Clim. Change 7, 243–249. doi: 10.1038/nclimate3231
Michalski, J., Bünger, U., Crotogino, F., Donadei, S., Schneider, G.-S., Pregger, T., et al. (2017). Hydrogen generation by electrolysis and storage in salt caverns: potentials, economics and systems aspects with regard to the German energy transition. Int. J. Hydrogen Energy 42, 13427–13443. doi: 10.1016/j.ijhydene.2017.02.102
Mletzko, J., Ehlers, S., and Kather, A. (2016). Comparison of natural gas combined cycle power plants with post combustion and oxyfuel technology at different CO2 capture rates. Energy Procedia 86, 2–11. doi: 10.1016/j.egypro.2016.01.001
Müller, B., Müller, K., Teichmann, D., and Arlt, W. (2011). Energiespeicherung mittels Methan und energietragenden Stoffen ein thermodynamischer Vergleich. Chem. Ingenieur-Technik 83, 2002–2013. doi: 10.1002/cite.201100113
Niaz, S., Manzoor, T., and Pandith, A. H. (2015). Hydrogen storage: materials, methods and perspectives. Renew. Sustain. Energy Rev. 50, 457–469. doi: 10.1016/j.rser.2015.05.011
Nozari, H., and Karabeyoǧlu, A. (2015). Numerical study of combustion characteristics of ammonia as a renewable fuel and establishment of reduced reaction mechanisms. Fuel 159, 223–233. doi: 10.1016/j.fuel.2015.06.075
Oliveira, L., Messagie, M., Mertens, J., Laget, H., Coosemans, T., and van Mierlo, J. (2015). Environmental performance of electricity storage systems for grid applications, a life cycle approach. Energy Conversion Manage. 101, 326–335. doi: 10.1016/j.enconman.2015.05.063
Pfenninger, S., and Staffell, I. (2016). Long-term patterns of European PV output using 30 years of validated hourly reanalysis and satellite data. Energy 114, 1251–1265. doi: 10.1016/J.ENERGY.2016.08.060
Reiter, G., and Lindorfer, J. (2015). Global warming potential of hydrogen and methane production from renewable electricity via power-to-gas technology. Int. J. Life Cycle Assess. 20, 477–489. doi: 10.1007/s11367-015-0848-0
Rodríguez, R. A., Becker, S., Andresen, G. B., Heide, D., and Greiner, M. (2014). Transmission needs across a fully renewable European power system. Renew. Energy 63, 467–476. doi: 10.1016/j.renene.2013.10.005
Schmidt, O., Gambhir, A., Staffell, I., Hawkes, A., Nelson, J., and Few, S. (2017). Future cost and performance of water electrolysis: an expert elicitation study. Int. J. Hydrogen Energy 42, 30470–30492. doi: 10.1016/j.ijhydene.2017.10.045
Singh, B., Strømman, A. H., and Hertwich, E. (2011). Life cycle assessment of natural gas combined cycle power plant with post-combustion carbon capture, transport and storage. Int. J. Greenhouse Gas Control 5, 457–466. doi: 10.1016/j.ijggc.2010.03.006
Staffell, I., and Pfenninger, S. (2016). Using bias-corrected reanalysis to simulate current and future wind power output. Energy 114, 1224–1239. doi: 10.1016/J.ENERGY.2016.08.068
Sutter, D., van Der Spek, M., and Mazzotti, M. (2019). 110th Anniversary: evaluation of CO2-based and CO2-free synthetic fuel systems using a net-zero-CO2-emission framework. Ind. Eng. Chem. Res. 58, 19958–19972. doi: 10.1021/acs.iecr.9b00880
US Department of Energy (2017). Fuel Cell Technology Offices Multi-Year Research, Development, and Demonstration Plan: US Department of Energy.
Valera-Medina, A., Xiao, H., Owen-Jones, M., David, W. I. F., and Bowen, P. J. (2018). Ammonia for power. Progress Energy Combust. Sci. 69, 63–102. doi: 10.1016/j.pecs.2018.07.001
van Der Spek, M., Bonalumi, D., Manzolini, G., Ramirez, A., and Faaij, A. (2018). Techno-economic comparison of combined cycle gas turbines with advanced membrane configuration and monoethanolamine solvent at part load conditions. Energy Fuels 32, 625–645. doi: 10.1021/acs.energyfuels.7b02074
Vojvodic, A., Medford, A. J., Studt, F., Abild-Pedersen, F., Khan, T. S., Bligaard, T., et al. (2014). Exploring the limits: a low-pressure, low-temperature Haber–bosch process. Chem. Phys. Lett. 598, 108–112. doi: 10.1016/j.cplett.2014.03.003
Wohland, J., Witthaut, D., and Schleussner, C. F. (2018). Negative emission potential of direct air capture powered by renewable excess electricity in Europe. Earth's Future 6, 1380–1384. doi: 10.1029/2018EF000954
Yapicioglu, A., and Dincer, I. (2019). A review on clean ammonia as a potential fuel for power generators. Renew. Sustain. Energy Rev. 103, 96–108. doi: 10.1016/j.rser.2018.12.023
Zappa, W., Junginger, M., and van den Broek, M. (2019). Is a 100% renewable European power system feasible by 2050? Appl. Energy 233–234, 1027–1050. doi: 10.1016/j.apenergy.2018.08.109
Keywords: energy storage, net-zero-CO2-emissions, direct air capture, intermittent renewable energy supply, CO2 capture and storage, chemical energy carriers, hydrogen, CO2 based fuels
Citation: Wevers JB, Shen L and van der Spek M (2020) What Does It Take to Go Net-Zero-CO2? A Life Cycle Assessment on Long-Term Storage of Intermittent Renewables With Chemical Energy Carriers. Front. Energy Res. 8:104. doi: 10.3389/fenrg.2020.00104
Received: 17 January 2020; Accepted: 08 May 2020;
Published: 23 June 2020.
Edited by:
Carlos Pozo, University of Girona, SpainReviewed by:
Ralf Peters, Julich-Forschungszentrum, Helmholtz-Verband Deutscher Forschungszentren (HZ), GermanyEdward J. Rode, DNV GL, Norway
Copyright © 2020 Wevers, Shen and van der Spek. This is an open-access article distributed under the terms of the Creative Commons Attribution License (CC BY). The use, distribution or reproduction in other forums is permitted, provided the original author(s) and the copyright owner(s) are credited and that the original publication in this journal is cited, in accordance with accepted academic practice. No use, distribution or reproduction is permitted which does not comply with these terms.
*Correspondence: Li Shen, TC5zaGVuQHV1Lm5s