- 1Department of Forestry, Wildlife, and Fisheries, Center for Renewable Carbon, The University of Tennessee Institute of Agriculture, Knoxville, TN, United States
- 2Department of Chemical and Environmental Engineering, Bourns College of Engineering, University of California, Riverside, Riverside, CA, United States
- 3Center for Environmental Research and Technology (CE-CERT), Bourns College of Engineering, University of California, Riverside, Riverside, CA, United States
- 4Joint Institute for Biological Science, Biosciences Division, Oak Ridge National Laboratory, Oak Ridge, TN, United States
- 5The Center for Bioenergy Innovation (CBI), Oak Ridge National Laboratory, Oak Ridge, TN, United States
- 6Department of Chemical and Biomolecular Engineering, The University of Tennessee, Knoxville, Knoxville, TN, United States
Conversion of technical lignin into performance biopolymers such as polyurethane offers environmental and economic advantages when combined with production of biofuels from biomass sugars, presenting significant interest toward studying the role of pretreatment on lignin structure and functionality. Co-solvent enhanced lignocellulosic fractionation (CELF) pretreatment, employing acidic aqueous tetrahydrofuran (THF) mixtures, was developed to effectively break down the lignin-carbohydrate matrix and promote extraction of lignin from lignocellulosic biomass with desirable purity and yield. In this study, we report the effects of CELF pretreatment reaction severity on the molecular structure of CELF-extracted lignin and its impact toward the mechanical properties of the resulting lignin-based polyurethanes. Reaction temperature was found to play the most significant role, compared to reaction time and acidity, in manipulating structural features such as molecular weight, functionality and intra-polymer structure. At the severe reaction conditions at 180°C, the order of reactivity for primary lignin interlinkages characterized by semiquantitative HSQC NMR analysis were found to be β-ether > phenylcoumaran (β−5′) > resinol (β−β′) facilitating a high degree of depolymerization and yielding a high frequency of free phenolics and reduced aliphatic hydroxyl groups. All side-chain interlinkages were depleted converting guaiacyl subunits into condensed forms, while still retaining uncondensed syringyl subunits. Under the mild 150°C temperature reaction, CELF lignin had higher molecular weight and retained more β-ether interlinkages. The results from CELF lignin-based polyurethane synthesis indicated that the tensile properties depended on the miscibility of CELF lignin with other components and low molecular weight cuts improved the dispersion of lignin in the polyurethane network. Pre-mixing of CELF with poly(ethylene glycol) (PEG) reduced the brittleness and improved the ductility of the CELF lignin-PEG polyurethanes.
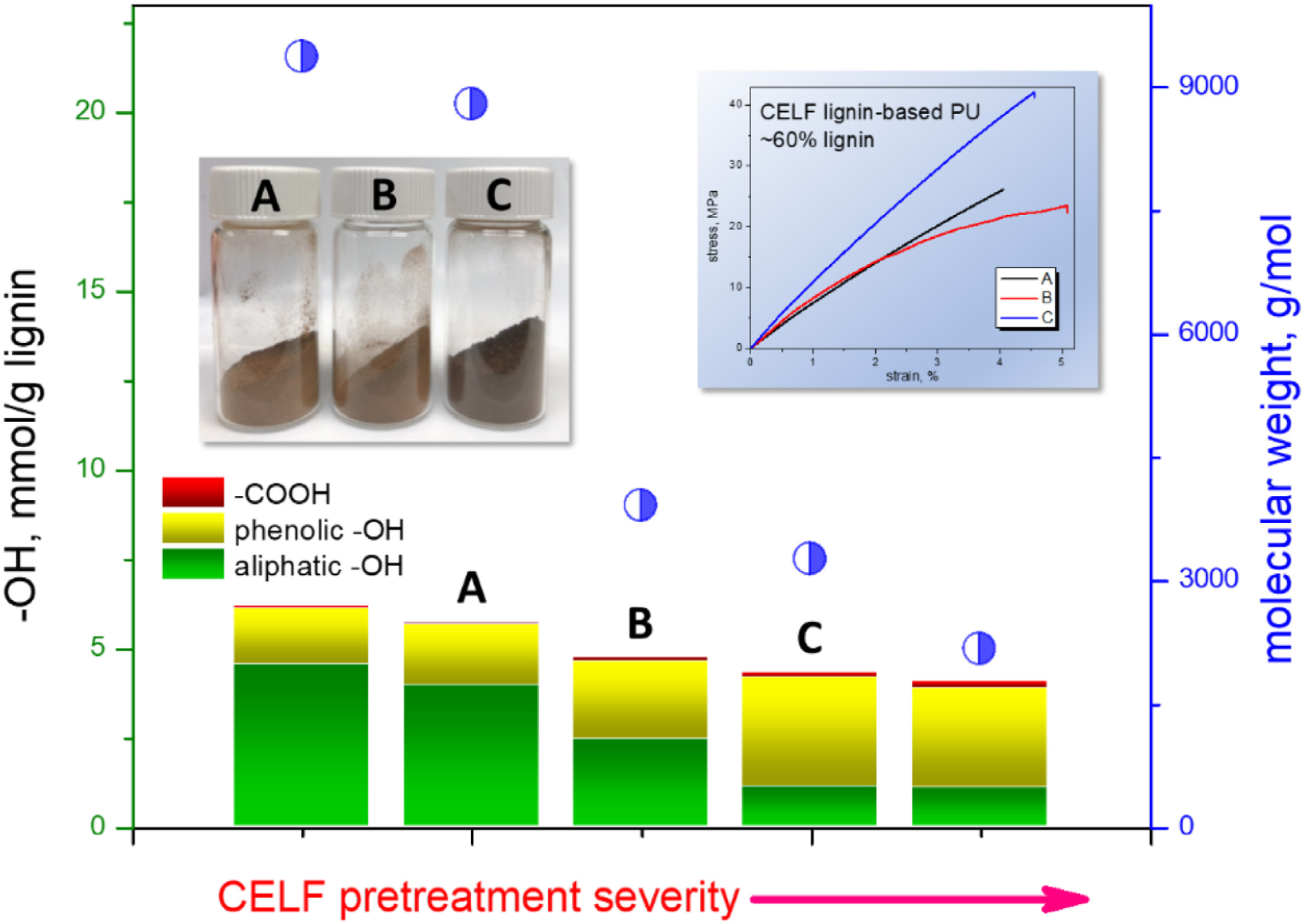
Graphical Abstract. The impact of pretreatment severity on the structure of CELF lignin and tensile properties of CELF lignin-based PU.
Introduction
Lignin found in lignocellulosic biomass is a class of heterogeneous biopolymers typically derived from three types of phenylpropanoid subunits: guaiacyl (G), syringyl (S), and p−hydroxylphenyl (H) (Higuchi, 2003). Angiosperm poplar lignin is composed of S, G with a varying S/G ratio depending on the species and a small amount of H subunits which are connected by six predominant interlinkages: β−O−4′, β−β′, β−5′, 5−5′, β–1′ and 4–O–5′ (Sannigrahi et al., 2010). In the plant cell wall, about 3% of the lignin subunits are covalently bonded with hemicelluloses to form lignin-hemicellulose matrix and lignin provides drought-resistance and a protective barrier against pathogen invasion (Balakshin et al., 2007; Giummarella et al., 2019). The recalcitrance of plant cell wall is designed by nature to be resistant to biological and chemical degradation. Therefore, in order to reduce the costs associated with processing lignocellulosic biomass to biofuels and biochemicals, pretreatment is often employed to modify the plant cell wall to improve accessibility of cellulolytic enzymes to the crystalline cellulose domains from which fermentable glucose can be released (Mostofian et al., 2016).
In order to improve upon conventional aqueous biomass pretreatment methods, the addition of miscible co-solvents greatly improves the dissolution of lignin that is critical in maximizing utilization of all major biomass fractions by subsequent catalytic and biological conversion methods. Co-solvent-based pretreatment technologies employing tetrahydrofuran (THF), γ-valerolactone (GVL), and Cyrene in aqueous solutions have been shown to provide significant functional advantages in improving microbial and enzymatic accessibility of cellulose while also achieving clean extraction of lignin and high total sugar recovery, merits that are important toward improving the competitiveness of liquid fuels from biomass (Shuai et al., 2016; Smith et al., 2017; Liu et al., 2018; Petridis and Smith, 2018; Meng et al., 2020). The pretreatment method that employs THF as a co-solvent is known as Co-solvent enhanced lignocellulosic fractionation (CELF). THF has uniquely lower boiling point so that it can be simply boiled out of the solution after pretreatment in order to induce lignin precipitating out of solution and to recover THF. This avoids potentially more complicated and energy-intensive solvent recovery methods, such as CO2-induced phase modification or anti-solvent extraction, that have been proposed for the recovery of high boiling co-solvents (Wyman et al., 2016). In previous studies, CELF has demonstrated wide operating flexibility in terms of reaction conditions such as temperature, solvent ratio, duration, and acid loading to finely control the extent of cellulose and lignin dissolution independently to support sugar hydrolysis at lower severities and to support tandem sugar hydrolysis and dehydration to furfurals at higher severities (Cai et al., 2013; Nguyen et al., 2016; Seemala et al., 2018). THF is non-pernicious and is considered a toxicologically safer alternative to dioxane and can be classified as a green chemical if produced from furfural by catalytic decarbonylation followed by hydrogenation (Cai et al., 2013; Fowles et al., 2013; Meng et al., 2018). Recently, all-atom molecular-dynamics (MD) simulation studies have probed the functionality of THF-water mixtures to “relax” native lignin globules into non-aggregated random-coils under the CELF pretreatment reaction environment to facilitate both lignin solvation and depolymerization, offering a wider operating range to alter the structure and degree of polymerization of lignin during pretreatment (Mostofian et al., 2016; Smith et al., 2016). This high degree of lignin tunability opens a broad range of potential pathways for upgrading lignin such as biopolymers, carbon substrates, antioxidants, resins, and hydrocarbon fuels (Ragauskas et al., 2006, 2014). While structural characterization of CELF lignin resulting from reaction conditions identified for achieving optimal total sugar recovery or high furfural yields have been reported previously (Meng et al., 2018, 2019b; Wang et al., 2018), a systematic study focused on elucidating the impact of pretreatment temperature, reaction time, and acid loading on lignin structure is needed to understand the potential spectrum of chemical moieties and inter-unit components that would be available to serve future lignin valorization efforts. Herein, the correlation between CELF pretreatment severity and resultant CELF lignin characteristics from hardwood poplar was established quantitatively by using 31P nuclear magnetic resonance (NMR), heteronuclear Single Quantum Coherence (HSQC) NMR, gel permeation chromatography (GPC), thermal gravimetric analysis (TGA) and differential scanning calorimetry (DSC). To improve our understanding of lignin fragmentation by acidolysis under CELF conditions, we tracked potential side-reactions such as lignin condensation and loss of monosaccharides as well as the primary acidolysis reaction on the lignin β–O–4′ interlinkages. Lignin has been considered as a sustainable and low-cost replacement for petrochemical polyols in the production of commercial polyurethanes products (Wang et al., 2017a). In the study of Kraft lignin-based polyurethanes, it was found that the mechanical strength of the polyurethane network was dependent on the molecular weight of Kraft lignin cuts prepared by sequential precipitation, and the presence of long-chain polyethylene glycol was able to improve the ductility of the materials (Wang et al., 2019). The understanding of CELF lignin molecular features, in return, facilitated the screening of lignin species for producing CELF lignin-based polyurethane (CL-PU) products such as adhesives.
Materials and Methods
Materials
The poplar wood chips used for this study is known as BESC standard poplar. It was determined through compositional analysis (NREL protocol TP-510-42618) to contain 21.2% acid-insoluble lignin (Sluiter et al., 2012). Before pretreatment, the poplar chips were knife-milled and passed through a 1 mm particle screen. Chemicals reagents such as THF, sulfuric acid, poly[(phenyl isocyanate)-co-formaldehyde] (PMDI, Mn∼340) and dibutyltin dilaurate were purchased from Sigma-Aldrich and Fisher Scientific.
CELF Pretreatment
Poplar wood chips were loaded into a 1 L Hastelloy Parr autoclave reactor (236HC Series, Parr Instruments Co., Moline, IL, United States) equipped with twin pitched-blade Rushton impellers at a solid to liquid loading of 7.5 wt%. The chips were soaked overnight at 4°C in a 1:1 (w/w) THF-water solution containing dilute mixtures of sulfuric acid (0.025 M to 0.1 M or 0.25–1% in liquid). The pretreatment reactions were carried out at temperatures of 150, 160, and 180°C for durations of 15 and 30 min. All reactions were maintained at target temperature (±1°C) by convective heating by using a 4 kW fluidized sand bath (Model SBL-2D, Techne, Princeton, NJ, United States), and the reactor temperature was measured directly by using an internally fixed thermocouple (Omega, K-type). To arrest the reaction after the allotted duration, the reactor was submerged in a large room-temperature water bath. The pretreated solids were then vacuum filtered and separated from the pretreatment liquor at room temperature through a paper filter. Finally, the dry mass of the solids and the mass of the liquor was recorded.
CELF Lignin Recovery and Purification
The liquid fraction collected from post filtration was poured in a beaker and titrated to pH ∼7 using ammonium hydroxide. THF was then boiled out of solution at 80°C under a hot plate with continuous stirring at 130 rpm for about 4 h. The beaker and contents were then allowed to cool to room temperature overnight and the liquor was then poured out. Lignin that had precipitated onto the beaker after the removal of THF and liquor was rinsed with water and then placed in a dark oven at 65°C to dry overnight. The resulting lignin was collected and placed onto a glass fiber filter paper. The lignin was then washed with diethyl ether followed by a water wash to remove soluble impurities and placed in an oven at 65°C to dry overnight to a moisture content of <3% (determined by gravimetric analysis at 105°C). The lignin was then ground to a fine powder by a mortar and pestle.
Structural Characterization of CELF Lignin
Quantitative 31P NMR and the heteronuclear single quantum coherence (HSQC) NMR spectra were acquired on a Bruker Avance III HD 500-MHz spectrometer according to a previously published literature (Wang et al., 2018; Meng et al., 2019a). In the quantitative 31P NMR experiments, a 90° pulse width, 1.2 s acquisition time, 25 s pulse delay were used in collecting 64 scans. 20∼30 mg (accurately weighed) CELF lignin sample was dissolved in 700 μL pyridine/CDCl3 (1.6:1, v/v) with 1 mg/mL chromium(III) acetylacetonate and 2.5 mg/mL N-hydroxy-5-norbornene-2,3-dicarboximide (internal standard). The lignin sample was subjected to NMR analysis promptly after phosphitylating with 60 μL 2-chloro-4,4,5,5 tetramethyl-1,3,2-dixoaphospholane (TMDP). The obtained 31P NMR spectra were calibrated by using the TMDP-water phosphitylation product (δ132.2 ppm) as the internal reference. The HSQC NMR spectra were processed and analyzed by using TopSpin software (version 3.5pl7, Bruker).
Gel Permeation Chromatography
Dried CELF lignin sample (∼2 mg) was acetylated and processed according to a previous literature (Wang et al., 2018). The acetylated CELF lignin was dissolved and then incubated in tetrahydrofuran for 24 h. The molecular weight analysis was performed on an Agilent GPC SECurity 1200 system equipped with several Waters Styragel columns (Water Corporation, Milford, MA, United States), an Agilent UV detector (λ = 280 nm) at a flow rate of 1.0 mL/min at 30°C.
Thermal Analyses
The thermal gravimetric analysis (TGA) of lignin was operated on a TA Q50 thermogravimetric analyzer (TA Instruments) heating in a nitrogen atmosphere. The sample (∼10 mg) was initially incubated at 105°C for 15 min to remove the last trace of moisture and THF. Then, the temperature was raised from 105 to 900°C at 10°C/min. The differential scanning calorimetry (DSC) measurements were performed in heat-cool-heat mode on a TA Q2000 DSC (TA Instruments) with a heating/cooling rate of 20°C/min.
CL-PU Synthesis and Characterization
The CL-PUs were synthesized by polycondensation as described in a previous literature, and they were denoted according to the corresponding CELF lignin samples (Wang et al., 2019). In this work, the selected CELF lignin samples, CELF2, CELF3 and CELF4, were disolved in THF with or without poly(ethylene glycol) (PEG, Mw = 4,000, Alfa Aesar) (1:1, w/w). The polyol/THF mixture was incubated in a thermal shaker (Alkali Scientific Inc.) at 140 rpm, 60°C for 1 h, and then it was combined with a THF solution containing PMDI with NCO/OH ratio at 1:1 and 1.5% dibutyltin dilaurate. After 3-day curing at room temperature, the CL-PU samples were kept at 150°C for 3 h. The tensile testing was carried out on a dual column Instron 5567 universal testing system equipped with a 500 N static load cell. For each CL-PU sample, three dog-bone specimens were tested according to ASTM D638 standard (Type V) at a strain rate of 0.1 mm/min.
Results and Discussion
Delignification in Acidic CELF Pretreatment
Poplar wood meal was pretreated under five CELF pretreatment conditions varying in catalyst dosage, temperature and duration time as summarized in Table 1. The THF-water content was fixed at 1:1 (w/w) which has been determined to be the minimum THF needed to achieve high delignification (Cai et al., 2013). The resultant CELF lignin samples were denoted “CELF1 – CELF5” referring to the degree of pretreatment severity. During the CELF pretreatment, the macromolecular lignin was degraded into fragments and dissolved in the THF-water mixture. Below 180°C, the removal of lignin increased steadily when the poplar biomass was pretreated at elevated temperature or with higher catalyst dosage. However, total CELF lignin yield after 180°C reaction was significantly higher for CELF5 (142.1% of total lignin in poplar biomass) as compared to CELF4 (94.4% of total lignin in poplar biomass). The mass in excess of 100% for the CELF5 sample was likely due to cross-polymerization reactions between soluble sugars and lignin during pretreatment and the formation of pseudo-lignin, a polyphenolic compounds derived from carbohydrates subjected to dilute acid reaction (Sannigrahi et al., 2011; Hu et al., 2012; Shinde et al., 2018). For example, Hu et al. found that up to 87% of the holocellulose was converted into acid insoluble pseudo-lignin including approximately 30% aqueous-dioxane-soluble pseudo-lignin after a severe two-step dilute acid pretreatment at 180°C (Hu et al., 2012). Pseudo-lignin preferentially forms via polymerization or polycondensation of carbohydrate degradation products at high temperature in the presence of oxygen during acid pretreatment (Hu and Ragauskas, 2014). It consumes valuable fuel precursors such as furfural, 5-hydxoylmethylfurfural, and levulinic acid; moreover, similar to lignin, pseudo-lignin absorbs on the surface of biomass and creates a recalcitrant barrier against cellulosic enzymatic hydrolysis (Hu et al., 2012). Therefore, the generation of pseudo-lignin should be suppressed during CELF pretreatment to provide higher yields of reactive intermediates from both the sugars and lignin in biomass.
Another index to evaluate the efficiency of CELF pretreatment is the molecular weight of the CELF lignin. The GPC profiles presented in Figure 1 showed the typical bimodal molecular weight distribution pattern for all five CELF lignin samples, and the impact of pretreatment severity on lignin depolymerization can be visualized by the intensity changes of high- and low-molecular weight peaks. At low pretreatment temperature (150°C), the high molecular-weight peaks were found to be predominant for CELF1 and CELF2, and mild degradation of lignin occurred as its Mw was reduced by 20∼25% compared with the reported value of poplar cellulolytic enzyme lignin (CEL) (Mw ∼ 12,000) (Meng et al., 2018). The most dramatic changes in molecular weights and polydispersity (PDI) were observed between CELF2 and CELF3: more than 50% reduction of Mw was achieved by increasing the pretreatment temperature from 150 to 160°C while other variables remained the same. However, Mw of CELF4 obtained at 180°C was decreased by an additional 17% compared with CELF3 (160°C). At 180°C, the reduction in molecular weight was caused by β–O–4′ acidolysis which was, however, partially compensated by the repolymerization of degraded lignin fragments via condensation.
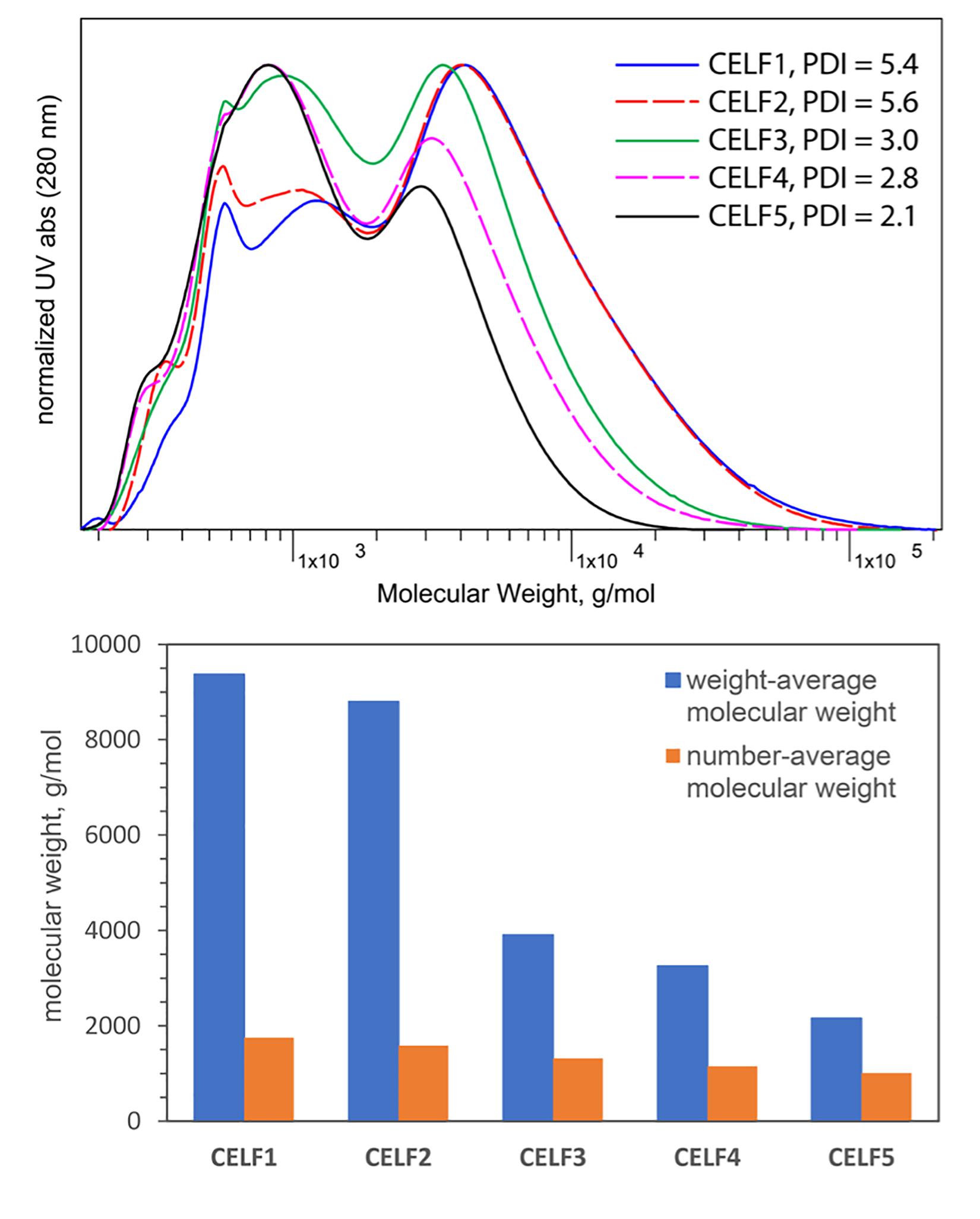
Figure 1. GPC profiles, weight-average and number average molecular weights of CELF lignin obtained under different pretreatment conditions.
Impacts of Pretreatment Severity on the Structural Features of CELF Lignin
Acid-catalyzed delignification preferentially starts from β–O–4′ linked alkyl aryl ethers with a free phenolic end, and the cleavage reaction proceeds along the polymer chain until reaching more recalcitrant bonds (Sturgeon et al., 2014). Under the acidic condition, the Cα position of beta ether loses a water molecule and forms a benzylic carbocation. The beta ether cleavage involves two pathways giving two end products: phenylacetaldehyde and Hibbert ketone (Scheme 1A), when sulfuric acid is used as the catalyst, forming Hibbert ketone is thermodynamically favored (Lundquist and Lundquist, 1972; Imai et al., 2011; Sturgeon et al., 2014). In addition to lignin depolymerization at low pH, C–C crosslinking between lignin components occurs via condensation reactions such as the one occurring on Cα as depicted in Scheme 1B (Li et al., 2018).
The multifunctionality of lignin macromolecules includes aliphatic, phenolic and carboxylic –OH groups. The phenolic -OH group can be classified into guaiacyl, C5-substituted and p-hydroxyphenyl. The hydroxyl contents of CELF1∼5 determined by 31P NMR analysis and the corresponding spectra are shown in Table 2 and Figure 2A, respectively. As the CELF pretreatment adopts more severe conditions, more free phenolic hydroxyl groups were released as a result of acid-catalyzed β–O–4′ cleavage, and they became the major functional groups (∼70%) found in CELF lignin when the pretreatment temperature was raised to 180°C (Figure 2B). Meanwhile, the relative content of aliphatic hydroxyl groups decreased from 74 to 26%. In the predominant lignin substructure, β–O–4′ alkyl aryl ether, the loss of aliphatic -OH groups arises from several factors including the cleavage of the monomeric components, oxidation of hydroxyl groups, and dehydration of side chain Cα and Cγ leading to Cα condensation or formation of stilbene structures (Hallac et al., 2010; Meng et al., 2018). The contents of syringyl and guaiacyl phenolic -OH groups increased comparably with the release of free phenolic ones (Table 2 and Figure 2C). However, unlike the former two, the p-hydroxyphenyl end units mainly derived from p-hydroxybenzoate were found to be more vulnerable to cleavage as its corresponding hydroxyl content decreased from ∼20 to ∼5% of the phenolic hydroxyl content.
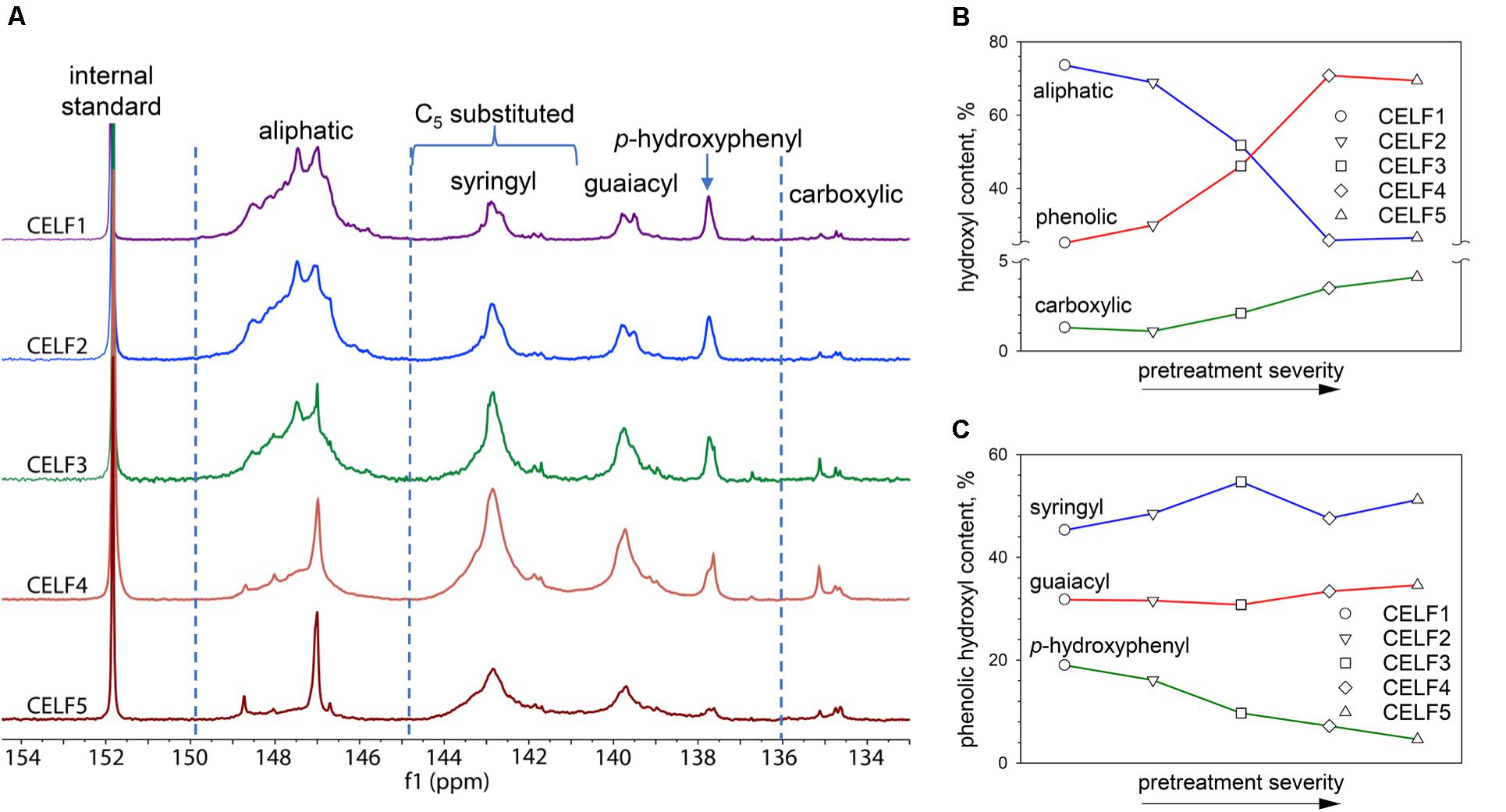
Figure 2. (A) Quantitative 31P NMR spectra of TMDP phosphitylated CELF lignin samples. (B) The relative quantities (%) of aliphatic, phenolic and carboxylic hydroxyl groups in total lignin hydroxyl groups. (C) The relative contents (%) of syringyl, guaiacyl and p-hydroxyphenyl hydroxyl groups in total lignin phenolic hydroxyl groups.
Detailed structural evolution of CELF lignin in relation to the pretreatment severity can be mapped by semiquantitative HSQC NMR analysis. As shown in Figure 3, the 2D HSQC spectra of CELF1, 3 and 5 prepared under mild, medium and harsh pretreatment conditions, were distinguishably different based upon the appearance and disappearance of some specific structural features. In the aliphatic region, CELF1 possessed clear and intensive characteristic cross-peaks such as β–O–4′ alkyl aryl ether (A), β–β′ resinol (B) and β–5′ phenylcoumaran (C) substructures, but it showed much fewer traces of carbohydrate signals compared with the poplar CEL (Meng et al., 2018). In the aromatic region, in addition to those well-defined cross-peaks of S and G subunits, and p-hydroxybenzoate substructure (PB), new cross-peaks of Scondensed and G2,condensed representing condensed S and G subunits, can be found around δ105.7∼106.9/δ6.46∼6.53 ppm and δ112.8/δ6.78 ppm, respectively. In the G subunits, condensation reactions can occur on open aromatic C5 or C6 and cause chemical shift migration of C2–H2 in the HSQC spectrum. In the spectrum of CELF3, the peak areas of Scondensed and G2,condensed expanded, and a weak cross-peak of Cγ–Hγ in lignin-bound Hibbert ketone (HK) end group can be observed. Under extreme pretreatment condition (1 wt% H2SO4, 180°C and 30 min duration time), CELF5 lost all side-chain interlinkages. The missing G2 and G6, remaining G5 cross-peaks indicated that all G subunits were in a condensed form and substitution on C6 was preferred at 180°C. Moreover, 5-hydroxymethylfurfural, a dehydration product of glucose, was found in CELF lignin, given that the cross-peaks of its C3–H3 (δ122.8∼124.2/δ7.50∼7.55 ppm), C4–H4 (δ109.6∼110.1/δ6.43∼6.62 ppm) and C6–H6 (δ55.8/δ4.55 ppm) can be clearly observed in the spectra of CELF4 and CELF5 (Constant et al., 2016).
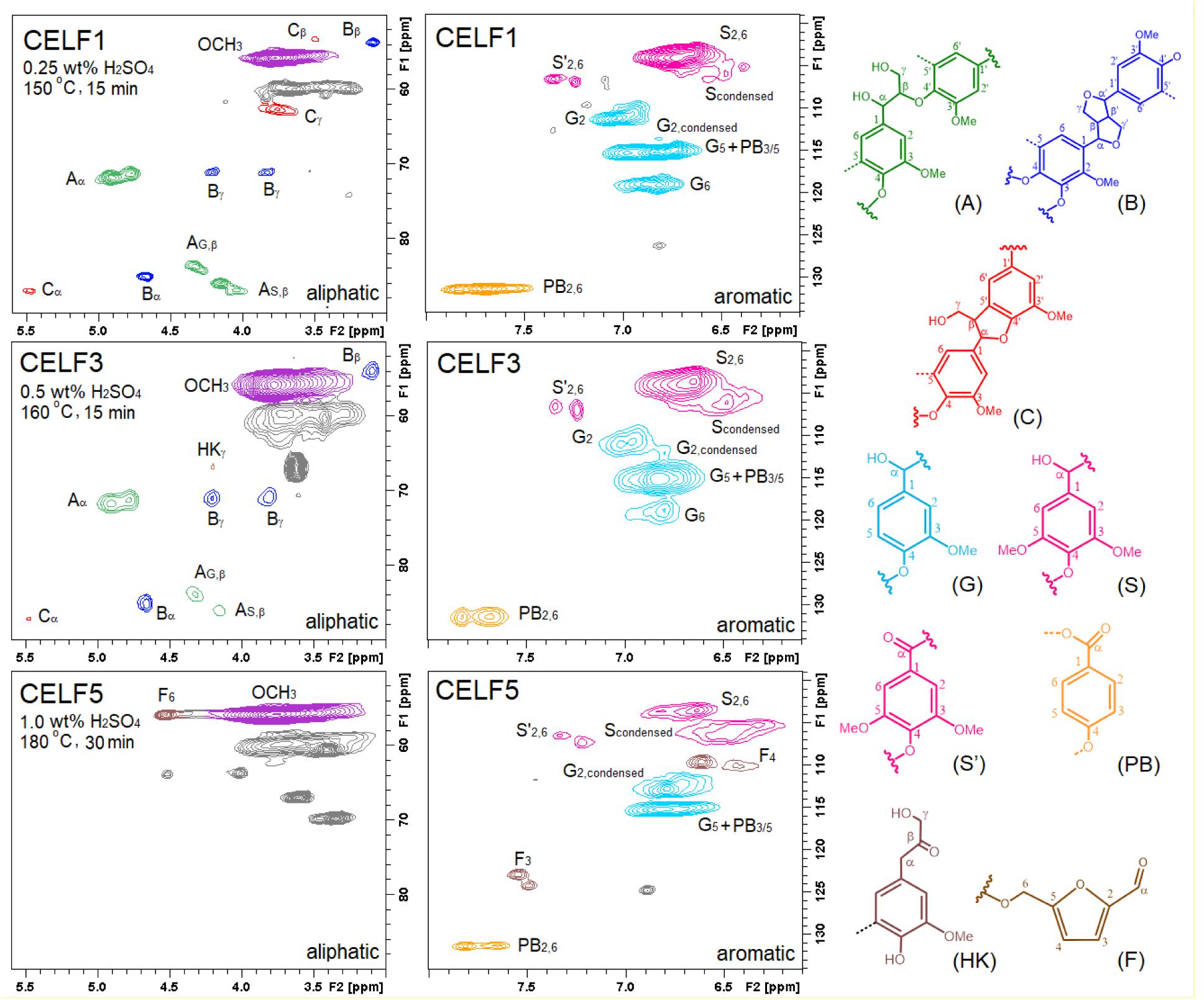
Figure 3. HSQC spectra of CELF1, 3 and 5. Structure (A) β–O–4′ linked alkyl aryl ether substructure; (B) β–β′ linked resinol substructure; (C) β–5′ and α–O–4′ linked phenylcoumaran substructure; (G) guaiacyl unit; (S) syringyl unit; (S′) oxidized syringyl unit; (PB) p–hydroxybenzoate substructure; (HK) Hibbert ketone; (F) etherified 5-(hydroxylmethyl) furfural.
The quantified impacts of pretreatment severity on lignin structure is summarized in Figure 4. Compared with other CELF lignin samples, CELF1 underwent minimal structural modification and preserved most of the native lignin structural features such as high frequency of β–O–4′ interlinkages [41 per 100 (S + G) units] and high molecular weight (Figure 4A). The content of β–O–4′ decreased rapidly from CELF1 to CELF5; on the other hand, β–β′ and β–5′ interlinkages were more resistant to acidolysis, but they were eventually cleaved or transformed at 180°C, and β–5′ that can only be formed from G subunits was removed more rapidly. Due to the presence of C5-methoxyl group, S subunits are favorably linked through β–O–4′. It was found that the transgenic poplar lignin composed of ∼98% S subunits possessed similar β–O–4′, but higher β–β′ content compares with wild poplar species (Stewart et al., 2009). Interestingly, in this work, the change of S/G with increasing pretreatment severity indicated that only at 180°C the loss of S subunits started to surpass the G ones accompanying with the removal of β–β′ (Figure 4B). Below 180°C, S2,6condensed/S was higher than G2condensed/G, but under harsh pretreatment conditions (180°C), the trend was reversed, and less than 80% of the S subunits were in condensed form. In the HSQC spectra of CELF lignin samples (Figure 3), the cross-peak at δ106.3/δ7.25 ppm is assigned to C2,6 in the oxidized S subunits, and Sox/S was hardly affected by pretreatment severity (Figure 4B). It has been reported that oxidation of Cα or Cγ–OH in β–O–4′ substructure can lower the C–O–aryl bond strength, and consequently facilitate lignin depolymerization (Guo et al., 2018). Therefore, the oxidized S subunits were presumably located at the end of the CELF lignin polymer chain.
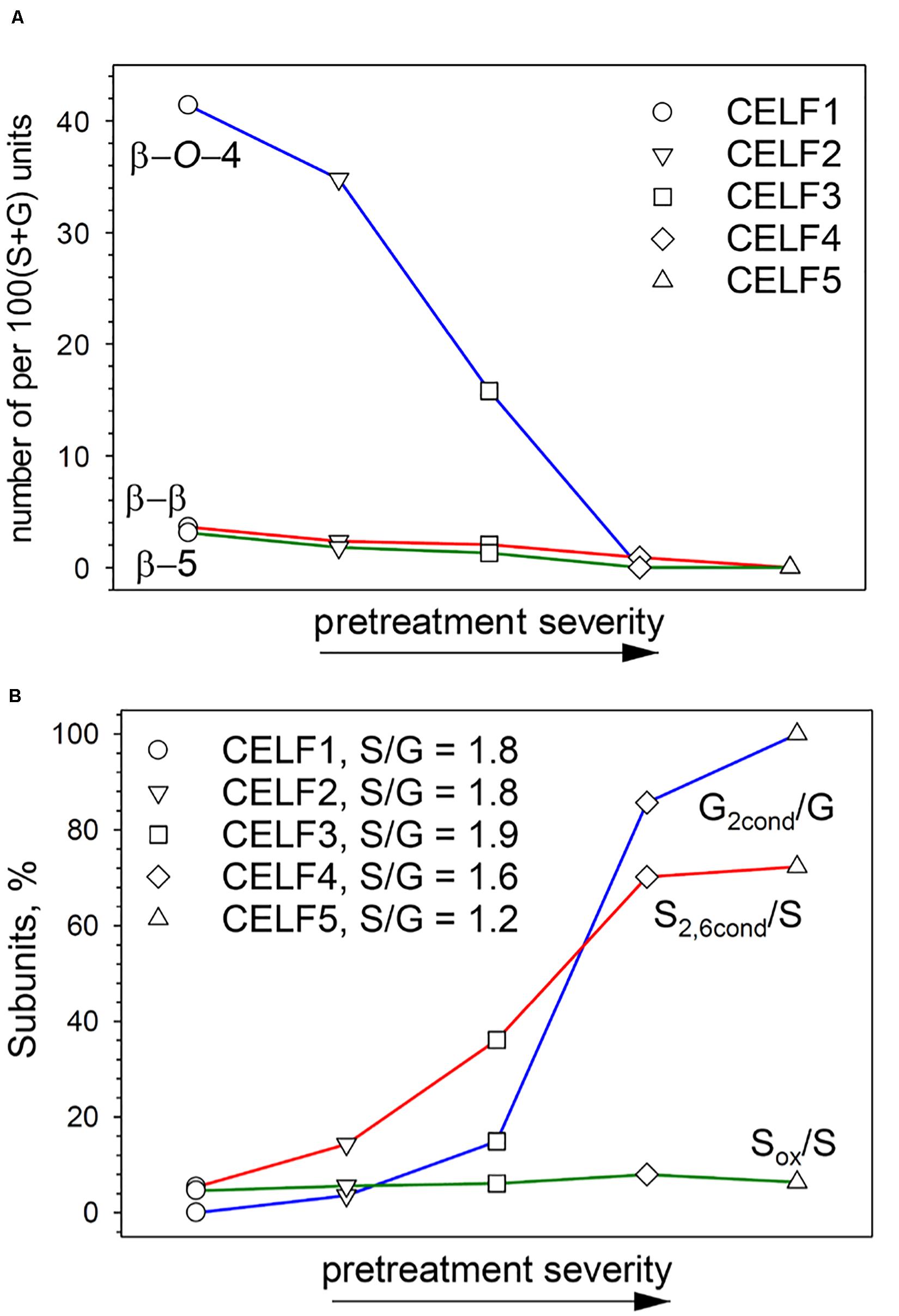
Figure 4. Semiquantitative HSQC analyses of CELF lignin interunit linkages and subunits. (A) Changes of β–O–4′, β–β′ and β–5′ interunit linkage contents [per 100 (S + G) units] with increasing pretreatment severity; (B) Changes of condensed guaiacyl (G2cond), condensed syringyl (S2,6cond) and oxidized syringyl (Sox) subunit contents with increasing pretreatment severity.
Correlation Between Thermal Behaviors and Molecular Structure of CELF Lignin
One of the pathways to the valorization of lignin isolated from CELF process is to incorporate them into polymeric materials; therefore, it is essential to have a deep fundamental understanding of their thermal behaviors. The DSC profiles in Figure 5A exhibited two distinct glass transition patterns for CELF lignin depending on the molecular structure that can be tuned by pretreatment severity. Below 180°C (CELE1∼3), glass transition temperature (Tg) of CELF lignin is positively correlated to molecular weight. The CELF lignin samples obtained at 180°C are highly condensed and crosslinked through rigid C–C bonds rather than C–O bonds. Although their molecular weights were significantly lower than CELF1∼3, no clear glass transition state can be observed for CELF4 and CELF5 within the experimental temperature range. In Figure 5B, the TGA thermograms indicated that the CELF lignin samples underwent three degradation steps. The most prominent peak arising from breaking C–C interlinkages and demethoxylation of aromatic rings at 350∼400°C can be observed for all CELF lignin samples (Wang et al., 2018). However, the peak was shrinking as the pretreatment becomes harsher, and such phenomenon is consistent with the decreasing S/G ratio caused by demethoxylation. The peak around 280°C is mainly caused by the bond rupture of ether interlinkages and aliphatic side chains, which release phenolic compounds, aldehydes, and carboxylic acids (Zhao et al., 2014). Its decay reflected lignin molecular structure evolving from flexible and native-like to a rigid and highly condensed under elevated pretreatment severity. The mass loss around 150°C is mainly attributed to dehydration of aliphatic hydroxyl groups (Hirose et al., 1998).
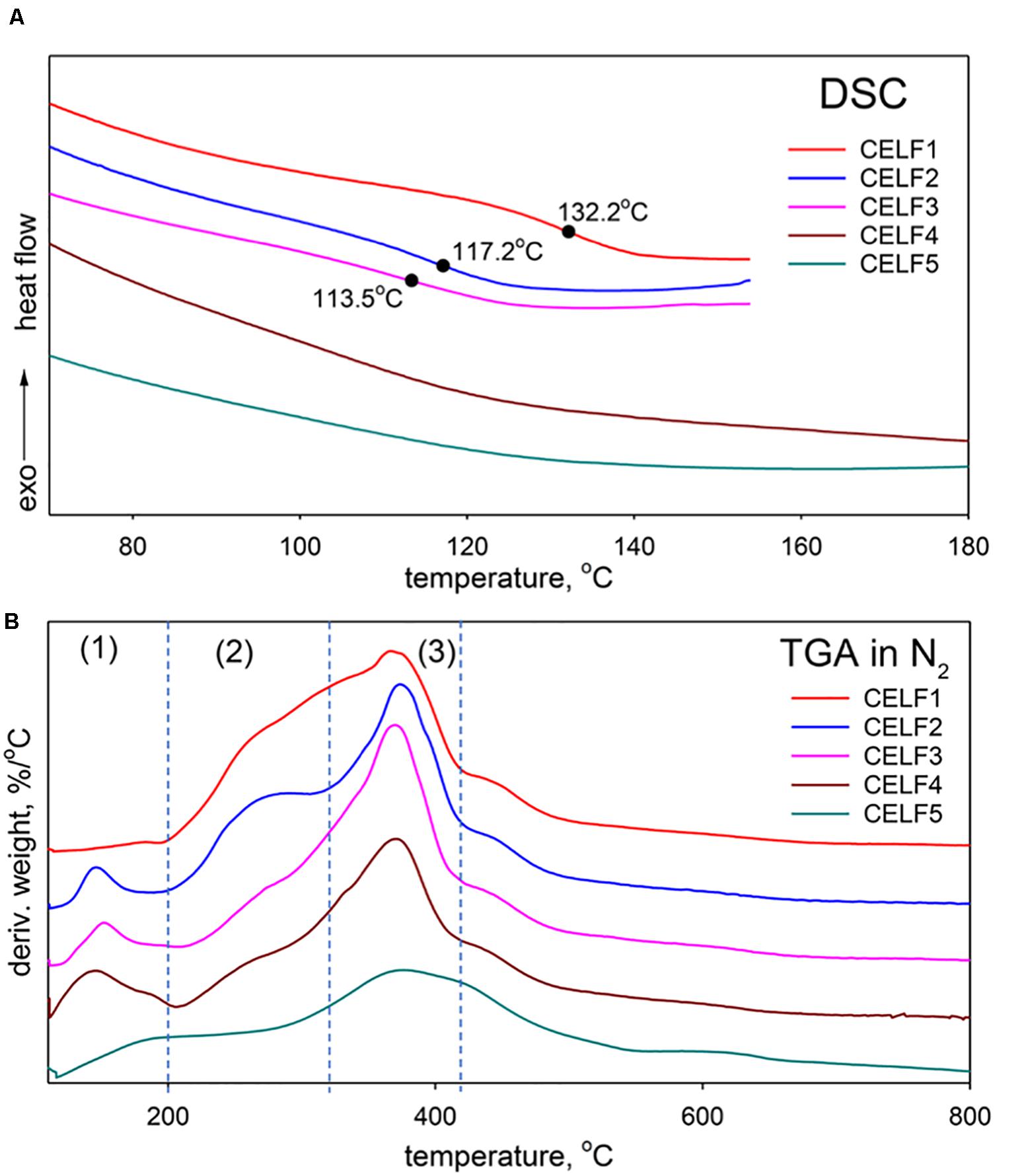
Figure 5. (A) DSC and (B) TGA analyses of CELF lignin samples obtained under different pretreatment conditions.
Screening CELF Lignin for CL-PUs
The CELF lignin samples, CELF2, CELF3 and CELF4 prepared under 150, 160, and 180°C pretreatment temperatures, were selected for producing CL-PUs. As shown in Table 3, the CL-PUs (CL2, CL3, and CL4) using lignin as the solo polyol were brittle materials with elongation at break (ϵb) hardly exceeded 5%, and their Young’s modulus (E), ultimate stress (σmax) increased as higher pretreatment temperature was employed. The aliphatic -OH groups in lignin are found more reactive in polyurethane synthesis, and urethane formation on the aromatic ring are less favorable due to steric hindrance effect and acidic character of phenolic -OH groups (Cateto et al., 2011). However, in this work, the mechanical properties of CL-PUs were determined by the miscibility between CELF lignin and PMDI in THF. In the sequential precipitation study, it was found that CELF lignin cuts with higher molecular weight inclined to precipitate out from the THF-methanol co-solvent as the solvent polarity decreased (Wang et al., 2018). Similarly, in this work, the solvation behavior of CELF lignin was manipulated by its molecular weight. CELF2 (Mw = 8800 g/mol) and CELF3 (Mw = 3900 g/mol) were not completely soluble in THF at 60°C, and further precipitation occurred when they were mixing with PMDI in THF. Compared to CELF3, CELF 4 (Mw = 3250 g/mol) possessed higher proportion of lower-molecular-weight lignin species as shown in the GPC profiles (Figure 1). CELF4 was fully soluble in THF at room temperature, and as a result, CL4 exhibited better E and σmax given the fact that CELF4 is structurally highly condensed and rigid. It was reported that PEG was able to form strong hydrogen bonds with lignin aliphatic and phenolic -OH groups, and thus disrupt the non-covalent intermolecular interaction between macromolecular lignin species (Kadla and Kubo, 2003; Wang et al., 2017b). Herein, 50% (w/w) PEG was pre-mixed with CELF lignin samples aiming to promote the solvation of the latter ones in THF. In general, the soft segments formed by PEG reduced the brittleness and improved the ductility of the CL-PEG PUs (Table 3). Consistent with the control set, the variation of ϵb for CL-PEG PUs indicated that the efficacy of PEG depended on the solvation behavior of CELF lignin.
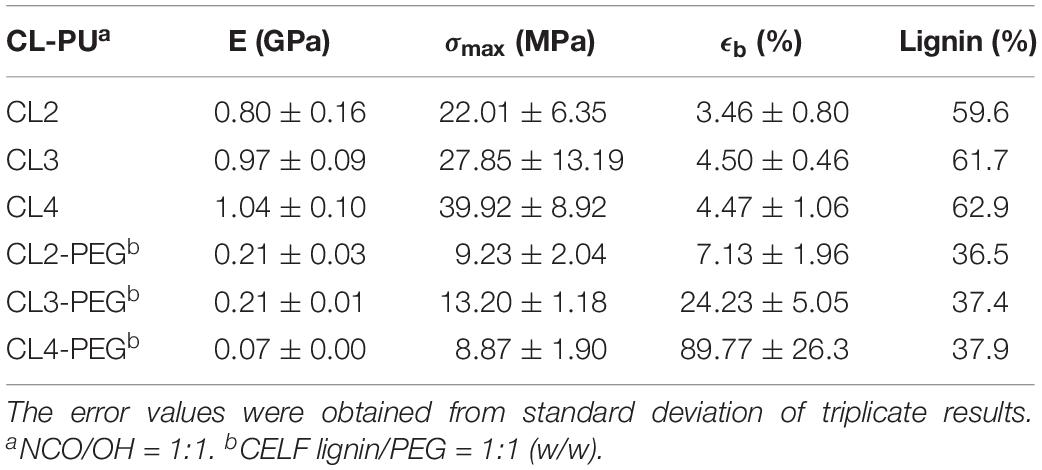
Table 3. The tensile properties of CL-PUs: Young’s modulus (E), ultimate stress (σmax) and elongation at break (ϵb).
Conclusion
Poplar biomass was pretreated in the CELF process under different conditions, in which lignin was depolymerized and extracted with acidic aqueous THF. The pretreatment severity strongly influenced the molecular weight, multifunctionality and intra-polymer structure characteristics of the co-product lignin. Mild CELF pretreatment at low temperature was conducted to reduce the changes on lignin chemical structure and preserve high molecular weight, high β–O–4′, and aliphatic hydroxyl contents. When the pretreatment temperature was increased from 150 to 180°C, the content of aliphatic hydroxyl groups was reduced 4-fold, which had a negative impact on the multifunctionality of CELF lignin. The studies of CELF lignin thermal behaviors confirmed that CELF lignin isolated from high-severity pretreatment was composed of hetero-oligomers with rigid and highly condensed molecular structure. Considering the efficiency of CELF process, high temperature (180°C) should be avoided given that monosaccharides can be wasted on the massive side-reactions forming pseudo-lignin and etherification between lignin and 5-hydroxymethylfurfural during the pretreatment. On the other hand, the synthesis of CL-PU indicated that the tensile properties depended on the miscibility of CELF lignin with other components such as PMDI, and the presence of PEG would disrupt the strong hydrogen bonding between lignin macromolecules and improve the dispersion of CELF lignin in the PU network. Therefore, for CELF lignin prepared under mild pretreatment conditions such as at 150 and 160°C, fractionation to separate out high molecular weight cuts will be required to improve its dispersion in the CELF lignin-based polyurethanes.
Author’s Note
The views and opinions of the authors expressed herein do not necessarily state or reflect those of the United States Government or any agency thereof. Neither the United States Government nor any agency thereof, nor any of their employees, makes any warranty, expressed or implied, or assumes any legal liability or responsibility for the accuracy, completeness, or usefulness of any information, apparatus, product, or process disclosed, or represents that its use would not infringe privately owned rights. The publisher, by accepting the article for publication, acknowledges that the United States Government retains a non-exclusive, paid-up, irrevocable, worldwide license to publish or reproduce the published form of this manuscript, or allow others to do so, for United States Government purposes. The Department of Energy will provide public access to these results of federally sponsored research in accordance with the DOE Public Access Plan (http://energy.gov/downloads/doe-public-access-plan).
Data Availability Statement
All datasets generated for this study are included in the article/Supplementary Material.
Author Contributions
Y-YW experiment design, data analyses, wrote and revised the manuscript. PS and BS conducted the pretreatment experiments and prepared CELF lignin samples. YP conducted HSQC NMR and data interpretation, revised the manuscript. CW, CC, and AR initiated the project, responsible for getting the funds, revised the manuscript. All authors read and approved the final manuscript.
Funding
We acknowledge the support through the U.S.D.A. National Institute of Food and Agriculture Grant 9008-004957 titled “Integrated Biorefinery to Produce Ethanol, High-Value Polymers, and Chemicals from Lignocellulosic Biomass.” Oak Ridge National Laboratory is managed by UT-Battelle, LLC under Contract DE-AC05-00OR22725 with the U.S. Department of Energy (DOE). This study was supported, in part, by the Center for Bioenergy Innovation (CBI), a U.S. Department of Energy Bioenergy Research Center supported by the Office of Biological and Environmental Research in the DOE Office of Science.
Conflict of Interest
The authors declare that the research was conducted in the absence of any commercial or financial relationships that could be construed as a potential conflict of interest.
Supplementary Material
The Supplementary Material for this article can be found online at: https://www.frontiersin.org/articles/10.3389/fenrg.2020.00149/full#supplementary-material
References
Balakshin, M. Y., Capanema, E. A., and Chang, H.-M. (2007). MWL fraction with a high concentration of lignin-carbohydrate linkages: isolation and 2D NMR spectroscopic analysis. Holzforschung 61, 1–7. doi: 10.1515/HF.2007.001
Cai, C. M., Zhang, T., Kumar, R., and Wyman, C. E. (2013). THF co-solvent enhances hydrocarbon fuel precursor yields from lignocellulosic biomass. Green Chem. 15, 3140–3145. doi: 10.1039/C3GC41214H
Cateto, C. A., Barreiro, M. F., Rodrigues, A. E., and Belgacem, M. N. (2011). Kinetic study of the formation of lignin-based polyurethanes in bulk. React. Funct. Poly. 71, 863–869. doi: 10.1016/j.reactfunctpolym.2011.05.007
Constant, S., Wienk, H. L. J., Frissen, A. E., Peinder, P. D., Boelens, R., and van Es, D. S. (2016). New insights into the structure and composition of technical lignins: a comparative characterisation study. Green Chem. 18, 2651–2665. doi: 10.1039/C5GC03043A
Fowles, J., Boatman, R., Bootman, J., Lewis, C., Morgott, D., Rushton, E., et al. (2013). A review of the toxicological and environmental hazards and risks of tetrahydrofuran. Crit. Rev. Toxicol. 43, 811–828. doi: 10.3109/10408444.2013.836155
Giummarella, N., Pu, Y., Ragauskas, A. J., and Lawoko, M. (2019). A critical review on the analysis of lignin carbohydrate bonds. Green Chem. 21, 1573–1595. doi: 10.1039/C8GC03606C
Guo, H., Miles-Barrett, D. M., Neal, A. R., Zhang, T., Li, C., and Westwood, N. J. (2018). Unravelling the enigma of ligninOX: can the oxidation of lignin be controlled? Chem. Sci. 9, 702–711. doi: 10.1039/C7SC03520A
Hallac, B. B., Pu, Y., and Ragauskas, A. J. (2010). Chemical transformations of buddleja davidii lignin during ethanol organosolv pretreatment. Energy Fuels 24, 2723–2732. doi: 10.1021/ef901556u
Higuchi, T. (2003). Pathways for monolignol biosynthesis via metabolic grids: coniferyl aldehyde 5-hydroxylase, a possible key enzyme in angiosperm syringyl lignin biosynthesis. Proc. Jpn. Acad. Ser. B 79B, 227–236. doi: 10.2183/pjab.79B.227
Hirose, S., Kobashigawa, K., Izuta, Y., and Hatakeyama, H. (1998). Thermal degradation of polyurethanes containing lignin studied by TG-FTIR. Polym. Int. 47, 247–256.
Hu, F., Jung, S., and Ragauskas, A. (2012). Pseudo-lignin formation and its impact on enzymatic hydrolysis. Bioresour. Technol. 117, 7–12. doi: 10.1016/j.biortech.2012.04.037
Hu, F., and Ragauskas, A. (2014). Suppression of pseudo-lignin formation under dilute acid pretreatment conditions. RSC Adv. 4, 4317–4323. doi: 10.1039/C3RA42841A
Imai, T., Yokoyama, T., and Matsumoto, Y. (2011). Revisiting the mechanism of β-O-4 bond cleavage during acidolysis of lignin IV: dependence of acidolysis reaction on the type of acid. J. Wood Sci. 57, 219–225. doi: 10.1007/s10086-010-1166-6
Kadla, J. F., and Kubo, S. (2003). Miscibility and Hydrogen Bonding in Blends of Poly(ethylene oxide) and Kraft Lignin. Macromology 36, 7803–7811. doi: 10.1021/ma0348371
Li, Y., Shuai, L., Kim, H., Motagamwala, A. H., Mobley, J. K., Yue, F., et al. (2018). An “ideal lignin” facilitates full biomass utilization. Sci. Adv. 4:eaau2968. doi: 10.1126/sciadv.aau2968
Liu, E., Li, M., Das, L., Pu, Y., Frazier, T., Zhao, B., et al. (2018). Understanding lignin fractionation and characterization from engineered switchgrass treated by an aqueous ionic liquid. ACS Sustain. Chem. Eng. 6, 6612–6623. doi: 10.1021/acssuschemeng.8b00384
Lundquist, K., and Lundquist, R. (1972). Acid degradation of lignin part VII. The cleavage of ether bonds. ACTA Chem. Scand. 26, 2005–2023.
Meng, X., Crestini, C., Ben, H., Hao, N., Pu, Y., Ragauskas, A. J., et al. (2019a). Determination of hydroxyl groups in biorefinery resources via quantitative 31P NMR spectroscopy. Nat. Protoc. 14, 2627–2647. doi: 10.1038/s41596-019-0191-1
Meng, X., Parikh, A., Seemala, B., Kumar, R., Pu, Y., Christopher, P., et al. (2018). Chemical transformations of poplar lignin during cosolvent enhanced lignocellulosic fractionation process. ACS Sustain. Chem. Eng. 6, 8711–8718. doi: 10.1021/acssuschemeng.8b01028
Meng, X., Parikh, A., Seemala, B., Kumar, R., Pu, Y., Wyman, C. E., et al. (2019b). Characterization of fractional cuts of co-solvent enhanced lignocellulosic fractionation lignin isolated by sequential precipitation. Bioresour. Technol. 272, 202–208. doi: 10.1016/j.biortech.2018.09.130
Meng, X., Pu, Y., Li, M., and Ragauskas, A. J. (2020). A biomass pretreatment using cellulose-derived solvent Cyrene. Green Chem. 22, 2862–2872. doi: 10.1039/D0GC00661K
Mostofian, B., Cai, C. M., Smith, M. D., Petridis, L., Cheng, X., Wyman, C. E., et al. (2016). Local phase separation of co-solvents enhances pretreatment of biomass for bioenergy applications. J. Am. Chem. Soc. 138, 10869–10878. doi: 10.1021/jacs.6b03285
Nguyen, T. Y., Cai, C. M., Osman, O., Kumar, R., and Wyman, C. E. (2016). CELF pretreatment of corn stover boosts ethanol titers and yields from high solids SSF with low enzyme loadings. Green Chem. 18, 1581–1589. doi: 10.1039/C5GC01977J
Petridis, L., and Smith, J. C. (2018). Molecular-level driving forces in lignocellulosic biomass deconstruction for bioenergy. Nat. Rev. Chem. 2, 382–389. doi: 10.1038/s41570-018-0050-6
Ragauskas, A. J., Beckham, G. T., Biddy, M. J., Chandra, R., Chen, F., Davis, M. F., et al. (2014). Lignin valorization: improving lignin processing in the biorefinery. Science 344, 709–720.
Ragauskas, A. J., Williams, C. K., Davison, B. H., Britovsek, G., Cairney, J., Eckert, C. A., et al. (2006). The path forward for biofuels and biomaterials. Science 311:484. doi: 10.1126/science.1114736
Sannigrahi, P., Kim, D. H., Jung, S., and Ragauskas, A. (2011). Pseudo-lignin and pretreatment chemistry. Energy Environ. Sci. 4, 1306–1310. doi: 10.1039/C0EE00378F
Sannigrahi, P., Ragauskas, A. J., and Tuskan, G. A. (2010). Poplar as a feedstock for biofuels: a review of compositional characteristics. Biofuels Bioprod. Biorefin. 4, 209–226. doi: 10.1002/bbb.206
Seemala, B., Meng, X., Parikh, A., Nagane, N., Kumar, R., Wyman, C. E., et al. (2018). Hybrid catalytic biorefining of hardwood biomass to methylated furans and depolymerized technical lignin. ACS Sustain. Chem. Eng. 6, 10587–10594. doi: 10.1021/acssuschemeng.8b01930
Shinde, S. D., Meng, X., Kumar, R., and Ragauskas, A. J. (2018). Recent advances in understanding the pseudo-lignin formation in a lignocellulosic biorefinery. Green Chem. 20, 2192–2205. doi: 10.1039/C8GC00353J
Shuai, L., Questell-Santiago, Y. M., and Luterbacher, J. S. (2016). A mild biomass pretreatment using γ-valerolactone for concentrated sugar production. Green Chem. 18, 937–943. doi: 10.1039/C5GC02489G
Sluiter, A., Hames, B., Ruiz, R., Scarlata, C., Sluiter, J., Templeton, D., et al. (2012). “Determination of structural carbohydrates and lignin in biomass,” in Laboratory Analytical Procedure (LAP), (Golden, CO: National Renewable Energy Laboratory).
Smith, M. D., Cheng, X., Petridis, L., Mostofian, B., and Smith, J. C. (2017). Organosolv-Water Cosolvent phase separation on cellulose and its influence on the physical deconstruction of cellulose: a molecular dynamics analysis. Sci. Rep. 7:14494. doi: 10.1038/s41598-017-15048-7
Smith, M. D., Mostofian, B., Cheng, X., Petridis, L., Cai, C. M., Wyman, C. E., et al. (2016). Cosolvent pretreatment in cellulosic biofuel production: effect of tetrahydrofuran-water on lignin structure and dynamics. Green Chem. 18, 1268–1277. doi: 10.1039/C5GC01952D
Stewart, J. J., Akiyama, T., Chapple, C., Ralph, J., and Mansfield, S. D. (2009). The effects on lignin structure of overexpression of Ferulate 5-hydroxylase in hybrid poplar. Plant Physiol. 150, 621–635. doi: 10.1104/pp.109.137059
Sturgeon, M. R., Kim, S., Lawrence, K., Paton, R. S., Chmely, S. C., Nimlos, M., et al. (2014). A mechanistic investigation of acid-catalyzed cleavage of aryl-ether linkages: implications for lignin depolymerization in acidic environments. ACS Sustain. Chem. Eng. 2, 472–485. doi: 10.1021/sc400384w
Wang, Y.-Y., Cai, C. M., and Ragauskas, A. J. (2017a). Recent advances in lignin-based polyurethanes. Tappi J. 16, 203–207.
Wang, Y.-Y., Chen, Y.-R., and Sarkanen, S. (2017b). Blend configuration in functional polymeric materials with a high lignin content. Faraday Discuss. 202, 43–59. doi: 10.1039/C7FD00083A
Wang, Y.-Y., Li, M., Wyman, C. E., Cai, C. M., and Ragauskas, A. J. (2018). Fast fractionation of technical lignins by organic cosolvents. ACS Sustain. Chem. Eng. 6, 6064–6072. doi: 10.1021/acssuschemeng.7b04546
Wang, Y.-Y., Wyman, C. E., Cai, C. M., and Ragauskas, A. J. (2019). Lignin-based polyurethanes from unmodified kraft lignin fractionated by sequential precipitation. ACS Appl. Poly. Mater. 1, 1672–1679. doi: 10.1021/acsapm.9b00228
Wyman, C. E., Cai, C. M., and Kumar, R. (2016). “Bioethanol from lignocellulosic biomass,” in Encyclopedia of Sustainability Science and Technology, ed. R. A. Meyers (New York, NY: Springer), 1–27.
Keywords: poplar lignin, NMR, lignin-based polyurethanes, pretreatment conditions, mechanical properties
Citation: Wang Y-Y, Sengupta P, Scheidemantle B, Pu Y, Wyman CE, Cai CM and Ragauskas AJ (2020) Effects of CELF Pretreatment Severity on Lignin Structure and the Lignin-Based Polyurethane Properties. Front. Energy Res. 8:149. doi: 10.3389/fenrg.2020.00149
Received: 11 March 2020; Accepted: 16 June 2020;
Published: 08 July 2020.
Edited by:
Junyong Zhu, United States Forest Service (USDA), United StatesReviewed by:
Peter Deuss, University of Groningen, NetherlandsSomnath D. Shinde, Sandia National Laboratories (SNL), United States
Copyright © 2020 Wang, Sengupta, Scheidemantle, Pu, Wyman, Cai and Ragauskas. This is an open-access article distributed under the terms of the Creative Commons Attribution License (CC BY). The use, distribution or reproduction in other forums is permitted, provided the original author(s) and the copyright owner(s) are credited and that the original publication in this journal is cited, in accordance with accepted academic practice. No use, distribution or reproduction is permitted which does not comply with these terms.
*Correspondence: Arthur J. Ragauskas, YXJhZ2F1c2tAdXRrLmVkdQ==
†ORCID: Yun-Yan Wang, orcid.org/0000-0002-0866-4640; Yunqiao Pu, orcid.org/0000-0003-2554-1447; Charles M. Cai, orcid.org/0000-0002-5047-0815; Arthur J. Ragauskas, orcid.org/0000-0002-3536-554