- 1Department of Chemical Engineering, Universitat Politècnica de Catalunya, Campus Diagonal-Besòs, Barcelona, Spain
- 2Renewable and Sustainable Energy Institute (RASEI), University of Colorado Boulder, Boulder, CO, United States
The global economy is shifting toward more sustainable sources of energy. The transportation sector is a remarkable example of this fact, where biofuels have emerged as promising alternatives to traditional fossil fuels. This work presents a techno-economic and environmental assessment of existing liquid fuels in hard-to-decarbonize sectors and their emerging renewable substitutes. The comparison focuses on fossil-based, biomass-derived, and plastic waste-sourced fuel alternatives that can be used in spark-ignition (gasoline) and compression-ignition (diesel) engines. Results for diesel substitutes prove the superior performance of plastic waste pyrolysis oil in terms of production cost reduction (−25% compared to diesel) and “well-to-tank” life cycle impact reduction (−54% human health, −40% ecosystems, −98% resources). Consequently, research and development toward the conversion of plastic waste into fuels should be extended to make the technology more accessible and robust in terms of fuel quality. On the contrary, the results for gasoline alternatives are not as conclusive: bioethanol and ethanol from plastic pyrolysis have a considerably lower impact on resource scarcity than gasoline (−80% and −35% respectively) and higher on the other two life cycle endpoint categories, but they have higher production costs compared to gasoline (+57% and +130% respectively). While blends of gasoline with pyrolysis-sourced ethanol can reduce the impact on human health and ecosystems, blends with bioethanol have a lower impact on resource scarcity and increase economic profitability. This allows fuel providers to offer tradeoff solutions in the form of blends based on their priorities.
Introduction
Current economies are transitioning to carbon-free energy sources, but there is still a need for liquid fuels for “hard-to-decarbonize” sectors (mainly aviation, shipping, and road transportation). The demand for diesel and gasoline for road transportation increased between 2000 and 2017 by 11.4%, with a diesel to gasoline usage ratio of 2.5 (Fuels Europe, 2018). Aviation and marine shipping are even harder to decarbonize, for instance through electrification, due to the higher involved mass and range, thus they will continue to rely on the use of liquid fuels (Gray et al., 2021). Cleaner fuels will be key to the transition to a more sustainable future. Biofuels have been widely studied but pose some challenges, mainly on land use, as they compete with the food supply chain.
The upcycling of plastic waste to produce chemicals and fuels with identical properties to those obtained from fossil sources has gained public interest because it slows down resource depletion and diverts waste that would traditionally end up in landfills (Lopez et al., 2017; Miandad et al., 2016). The chemical recycling of plastic waste will play a vital role in closing material loops and shifting toward a circular economy paradigm. Among the different alternatives for the material recovery of plastics (e.g., pyrolysis, gasification, and cracking), pyrolysis stands out for offering the possibility of recovering valuable chemicals (Dahlbo et al., 2018). It even allows the recovery of the plastic monomer, thereby offering the possibility to close the concerning carbon loops in the plastic supply chain. However, these technologies have been mostly developed and tested at the lab and pilot scale and there are several barriers to their industrial implementation such as technological, financial, managerial, performance limitations, and social barriers, among others (Araujo Galvão et al., 2018). For instance, PLASTIC ENERGYTM operates a 5,000 t/year mixed plastic waste pyrolysis pilot plant in Seville, Spain. This pilot project has led to collaborations with big companies in the oil and plastics market. An example is the construction of a semi-commercial plastic waste recycling, and a pyrolysis oil refinery project is due to be constructed in 2021 in Gleen in the Netherlands (SABIC, 2019).
While fuel products ultimately end up as carbon dioxide (CO2) in the environment, other chemical products, such as plastics, have a variety of end-of-life alternatives, including incineration, landfill, and mechanical or chemical recycling. Recently, Somoza-Tornos et al. (2020) compared alternatives using techno-economic and Life Cycle Assessment (LCA). The authors concluded that recovering chemicals (specifically ethylene from polyethylene) from plastic waste offers an economic incentive and a positive environmental impact by avoiding the burden of direct incineration and especially dealing with landfill, which stands as the least preferable option where all the plastic value is lost in a non-degradable graveyard. Additionally, they applied similar methods to select the best alternatives from plastic waste upcycling into chemicals in recent contributions (Pacheco-López et al., 2020; Somoza-Tornos et al., 2021).
This study provides a techno-economic and environmental assessment of diesel and gasoline substitutes, namely biodiesel, bioethanol, plastic waste pyrolysis oil, and plastic waste pyrolysis ethanol. Through this assessment, the advantages and disadvantages of each pathway are revealed. The first part of this study assesses alternative feedstock and pathways to obtain a fuel that can be used as a diesel substitute. The second part deals with ways to produce ethanol as a gasoline substitute. To that end, literature data has been compiled to track the cost of already mature pathways while estimations of the cost of pyrolysis products are based on a techno-economic assessment. The environmental impact is compared through an LCA approach.
Materials and Methods
This section introduces the scope of the current work and presents the chosen functional unit used in the study. The proposed polypropylene (PP) pyrolysis process is explained along with the followed techno-economic and environmental assessment procedures.
Scope
The scope of this contribution lies in the production of fuels for the direct replacement of fossil fuels in non-modified engines. Figure 1 compares alternatives: fossil-based diesel and gasoline are compared against biodiesel and bioethanol as well as plastic waste pyrolysis oil and plastic waste pyrolysis ethanol, respectively.
Biomass has emerged as a promising alternative feedstock to produce fuels of similar quality as fossil-based products while creating a closed carbon cycle. However, adverse effects such as competition with the food and water sector as well as intense land usage and other kinds of environmental impact must be analyzed on a case-by-case basis to choose the most suitable feedstock (Herrmann et al., 2018).
Pyrolysis products from plastic waste can have a wide range of compositions and properties depending mainly on the following conditions: type of plastic, catalyst, and most importantly temperature (Lopez et al., 2017). High temperature pyrolysis breaks the polyolefin into smaller compounds, leading to higher gas yields. Low temperature pyrolysis on the other hand leads to higher oil yields with increasingly heavier compounds as the temperature is reduced.
It must be acknowledged that alternative fuels and engines for transportation purposes such as electricity (Glitman et al., 2019) or hydrogen (Sharma and Krishna Ghoshal, 2015) will play an important role in the transition to a more sustainable transportation sector. However, during this transition period, it will be a natural step to utilize the machines that are already available and predominantly produced nowadays, that is 52.3% gasoline and 29.9% diesel engines (ACEA, 2020). Therefore, the approach proposed will focus on targeting fuels suitable for traditional engine vehicles, complying with their technical requirements and exhaust emissions standards.
The full life cycle assessment of fuel is often referred to as “Well-to-Wheel” analysis and it comprises two phases: 1) the “Well-to-Tank” phase, which consist of all the steps from raw material procurement over processing until obtaining the final fuel, and 2) the “Tank-to-Wheel” phase, which describes the combustion of a fuel in an engine (Edwards et al., 2014; Brinkman et al., 2005). This work assesses the Well-to-Tank phase. This approach was chosen due to the variation of the emission values reported in the literature for different raw materials and conversion procedures in the pyrolysis path (Damodharan et al., 2019). Energy demand and emissions in the Well-to-Tank phase can be estimated more reliably due to the steadiness of the process, while the Tank-to-Wheels phase is more variable and the results depend on a big set of variables and conditions. It is important to remark that all data was collected or extrapolated to refer to the year 2019.
Diesel Substitutes
Diesel is a mixture of hydrocarbons in the boiling point range from 150 to 380°C, traditionally obtained from fractioning crude oil in refineries. Its use in car engines has been commercialized for over 100 years. The considered pathways and alternatives for diesel engines are represented in Figure 1A.
Oils obtained from the catalytic low temperature pyrolysis of plastic waste have proven usable in conventional diesel engines (Wong et al., 2015). Damodharan et al. (2019) present an exhaustive overview of the research that has been carried out in the field during the last 20 years. From their review, it is evident that detailed performance criteria (e.g., heat release, nitrogen oxides, smoke emissions, etc.) in engine tests vary significantly from study to study. However, a clear consensus is that the oils can be used without complications and engine modifications in low blending ratios with conventional low sulfur diesel. Chandran et al. (2020) concluded that a content of 30% volume (v/v) of Plastic Waste Pyrolysis Oil (WPO) in diesel allows for long-term operation without engine modifications while Singh et al. (2020) reported that ratios of up to 50% are feasible under a slight increase in carbon monoxide (CO) emissions at high loads.
Biodiesel obtained from vegetable oils, animal fats or waste cooking oil appears as another sustainable alternative to fossil-based diesel. The basic principle of producing biodiesel is the transesterification of oils and fats to obtain smaller esters and glycerin, a mixture that has been used commercially in diesel engines since the early 1990s (Pahl, 2009). Similar to WPO, pure biodiesel can be used as-is in modified diesel engines and usually in blends up to 20% v/v with fossil-based diesel without any modifications to the engine (Alleman et al., 2016).
Gasoline Substitutes
A widely employed gasoline substitute is ethanol. It is the second aliphatic alcohol (C2H5OH) and has a wide range of applications: it can act as a solvent, conservative, disinfectant, and precursor to various other chemical products (e.g., acetic acid, ethyl esters, etc.). This study focuses on its application as a fuel substitute in internal combustion engines (Ilves et al., 2018). It can be blended with diesel at low ratios but its most common use is blended with gasoline in spark ignition engines.
The path to obtaining ethanol from plastic waste is depicted in Figure 1B. Somoza-Tornos et al. (2020) presented a detailed techno-economic and life cycle assessment of an 18.9 t/h polyethylene (PE) waste pyrolysis plant with high purity (99.5%) and ethylene yield of 46%. The gas mixture leaving the furnace is subsequently separated into its compounds through heat integrated cryogenic distillation. Ethanol can then be obtained from ethylene through hydration. Ethanol obtained from this route is referred to from here on as Plastic Waste Pyrolysis Ethanol (WPE).
Traditionally, ethanol is obtained from biomass (i.e., bioethanol). A variety of feedstock can be used for that purpose: for instance, while sugarcane is widely employed in Brazil, the main feedstock for bioethanol in the United States is corn. Obtaining ethanol from these feedstock follows in principle the same steps: pretreatment (milling), fermentation, and distillation (Canilha et al., 2012). As a renewable resource, bioethanol contributes to sustainability in the transportation sector. However, the main controversies surrounding bioethanol are associated with its land usage and competition with the food sector. Additionally, in many cases, its cost exceeds those of fossil-based fuel.
Pathway Selection
The business as usual pathways considered in this study are fossil-based fuels sourced from crude oil. The assessed costs and impacts cover the extraction and refining processes based in Europe.
Biofuels can be obtained from a variety of raw materials that are grouped as first-, second-, and third-generation. The pathways selected in this study are the first-generation fuels that are obtained from generally edible biomass. They were chosen because of their high maturity and easy to gather, reliable data for comparison. The biodiesel pathway studied herein covers acquisition and processing based on the mixture of raw materials employed in Europe, that is dominated by rapeseed (European Commission, 2020). For bioethanol also, the European mixture is studied, which mostly consists of maize, wheat, and sugar beet (European Commission, 2020).
Plastic waste pyrolysis oil to substitute diesel can be obtained from different types of plastic. Here, PP is chosen as raw material because its derived oils were shown to perform better in engines than those obtained from other plastics (Mangesh et al., 2020). The pyrolysis process is simulated in this study to obtain equipment dimensions for the techno-economic assessment and energy and emission values for the life cycle assessment. As a substitute of a gasoline additive, the pathway to produce ethanol from the pyrolysis of polyethylene (PE) is chosen due to its favorable ethylene yield, as reported by Kannan et al. (2014). The costs and impacts of the complete pathway are estimated using references for the pyrolysis process and the subsequent hydration steps. All calculations assume the costs and impacts in Europe.
Functional Unit
Among all data found in the literature and the results obtained from commercial simulators and LCA tools for techno-economic and environmental assessment, there are properties defined through different functional units. Some properties refer to the amount of fuel such as liters or kilograms, however, not all fuels store the same amount of energy and therefore different fuels can deliver different amounts of energy. For that reason, an energy dimension is chosen as a functional unit, in particular Gigajoules (GJ). In order to adapt fuel properties to this functional unit, fuel density is used to convert liters to kilograms when necessary, and Lower Heating Values to convert kilograms to GJ. The conversion factors considered are shown in Table 1.
Process Simulation: Polypropylene Pyrolysis
This work presents a process simulation of low temperature polypropylene pyrolysis. Figure 2 depicts the flowsheet of the process including material and energy balances. The polymer pyrolysis heat demand is determined using Aspen Plus V11 with the POLYNRTL package. The group contribution-based property model allows an estimation of the polymer properties and thus the necessary heat for running the reaction. The condensation of the pyrolysis products is simulated in Aspen Hysys V11 using the Peng-Robinson equation of state to obtain accurate values of the hydrocarbon equilibrium and cooling demand.
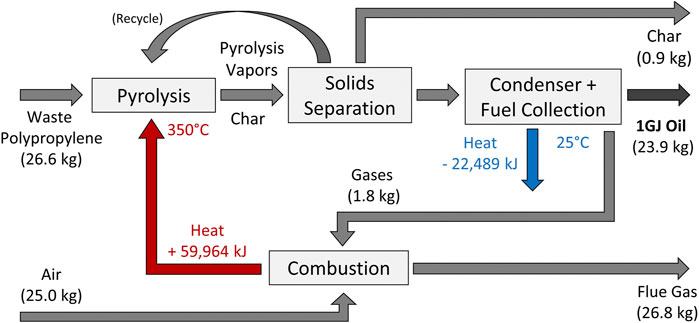
FIGURE 2. Schematic representation of material and energy balances of the PP pyrolysis process. A possible recycling structure is indicated but not utilized in this work.
Polypropylene is modeled as a 20-segment isotactic oligomer of propylene (C63H128, Molar mass 885.7 g/mol). A pseudo-stoichiometry has been obtained from the pyrolysis product composition and oil yields reported by Mangesh et al. (2020). The cited study uses colorless 2 mm sized shreds of polypropylene obtained from bottles, cans, and containers. Conversion of 100 g samples took place in the presence of 25 g of micro-mesoporous zeolite ZSM-5 catalyst in a 250 mm stainless-steel reactor under vacuum. Reaction time is 30 min at 350°C. Further details on catalyst preparation and product characterization can be found in Mangesh et al. (2020). The pyrolysis product is a complex mixture of hydrocarbons. These products have been grouped into classes according to their characteristics and each class has been represented by a single component (Table 2). Further details on the estimation of pyrolysis oil properties and simulation in Aspen software can be found in the Supplementary Material.
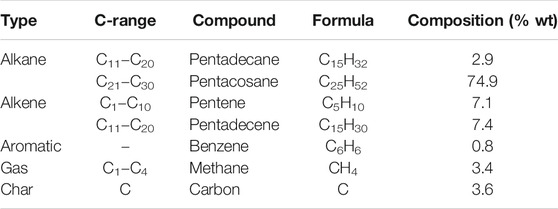
TABLE 2. Polypropylene pyrolysis products adapted from Mangesh et al. (2020).
A 710 GJ/h production plant capacity was chosen, which corresponds to the plastic waste output (450 t/day) of a city with around 8 million people such as London. The process is simulated in steady-state mode. The plastic feed enters the pyrolysis reactor at 25°C and 1 bar. A heat of 14.7 MW is required to drive the decomposition reaction at 350°C and 1 bar. To that end, the 1.3 t/h product gas stream is burned in a furnace with an 80% efficiency and 20% air excess. After leaving the reactor, the solid char phase is separated from the liquid and gas phase through mechanical separation (e.g., filtering). The fluid products are cooled down to 25°C in an adiabatic condenser with a 5.6 MW cooling demand that is supplied by a cooling water utility. Heat and material losses in piping and the solid separation are neglected. For further details about this process, refer to the Supplementary Material.
Techno-Economic Assessment
The techno-economic assessment performed herein compares the production costs of the different pathways. Breakdowns of these costs into capital expenses (CapEx), operational expenses (OpEx), and raw material costs are obtained to determine major cost drivers in each pathway. Market prices are collected to show the possible profit margin of each pathway and blends of fuels. Tables 3, 4 detail the gathered data and its sources along with the results of the techno-economic assessment for each alternative. When available, price averages were taken over the period ranging from 2016 to 2020. All economic data for fossil fuels and biofuels were collected from literature sources.
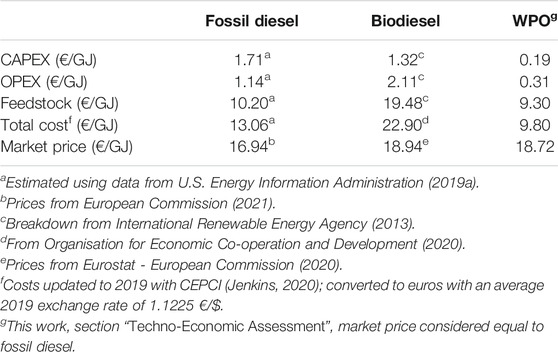
TABLE 3. Production cost breakdown of diesel and alternatives. Densities and Lower Heating Values used are shown in Table 1.
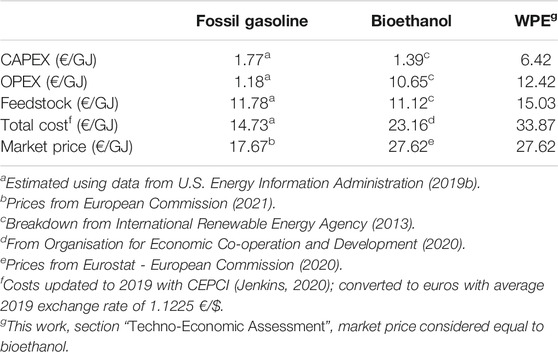
TABLE 4. Production cost breakdown of gasoline and alternatives. Densities and Lower Heating Values used are shown in Table 1.
WPO production cost is estimated following the method used in Gonzalez-Garay et al. (2017) and developed in chapter 6.2 from Sinnott and Towler (2020). The annual capital and operating costs are estimated. They are divided by the annual production to obtain unitary production costs. A detailed explanation of this cost estimation procedure is further developed in Section 1.3 of the Supplementary Material. Since currently WPO is not a traded product, its price has been estimated as equal to that of fossil diesel. The production costs of WPE are calculated using references for pyrolysis, separation (Somoza-Tornos et al., 2020), and catalytic hydration (Ayaou et al., 2020). In other words, the production cost of WPE is estimated as the sum of costs for the PE pyrolysis, the separation of the gas products, and the conversion of ethylene to ethanol processes.
When necessary, costs are extrapolated to 2019 using the Chemical Engineering Plant Cost Index (CEPCI) (Jenkins 2020) and converted from dollars into euros with the average exchange rate for 2019 (1€ = 1.1225$). Additionally, all prices and costs are adapted to refer to the chosen functional unit (i.e., €/GJ) using Table 1.
For the proposed blends between traditional fuels and their substitutes, the prices are estimated using Eq. 1, where
Environmental Assessment
The environmental assessment performed in this study is a cradle-to-gate, or as previously introduced, Well-to-Tank, LCA following the ISO 14040:2006 standards (ISO, 2016). To compare values between different fuels, the functional unit is set to the amount of fuel equivalent to 1 GJ of Lower Heating Values. For the fossil-based fuels and biofuels, the life cycle inventories from the EcoInvent database v3.4 (Wernet et al., 2016) were retrieved and the results of the final impact are analyzed through ReCiPe2016 methodology (Huijbregts et al., 2017) with the SimaPro software (Goedkoop et al., 2014) using the hierarchical approach.
For the plastic waste pyrolysis pathways, no pre-defined inventories are available in EcoInvent. The WPO inventory was obtained through the mass and energy balances from the process simulation. The complete inventory is shown in Table 5. A cut-off approach for the PP waste inlet is assumed (i.e., waste is free from primary material burden) (Gentil et al., 2010). Thus, the environmental impacts of PP waste are assumed to be only associated to the sorting process. The impacts of PP sorting are approximated to those of polyethylene sorting due to data availability and the similar properties of both polymers in terms of chemistry and application. The impact of cooling water is calculated as the electricity required for pumping assuming a conversion factor of 9.5 kWh/MWh of cooling energy and considering 10% extra water to compensate for evaporation and other losses. The environmental impact associated with the furnace and side equipment units is estimated using the corresponding steel requirements, considering a lifetime of 25 years. The environmental impact of WPO is compared to the impact obtained from:
• Diesel [“Diesel (Europe without Switzerland) | petroleum refinery operation”] and,
• Biodiesel [“Vegetable oil methyl ester (Europe without Switzerland) | esterification of rape oil”]
The entry for biodiesel production in Europe without Switzerland from only rape oil is selected because it is the dominating feedstock for biodiesel production in Europe and EcoInvent database offers no inventory for mixed production of the other raw materials.
Similar to the approach used in techno-economic assessment, the environmental impact from the pyrolysis of PE waste into ethylene were retrieved from Somoza-Tornos et al. (2020) and allocated according to the economic weight of each product. Then, the subsequent impact associated with the hydration of ethylene into ethanol is assumed to be equivalent to the same operation in the case of fossil-based ethanol. Considering the stoichiometric relation (0.61 kg ethylene/kg ethanol):
with:
• Fossil Ethanol [“Ethanol, without water, in 99.7% solution state, from ethylene (RER)| ethylene hydration”];
• Fossil Ethylene [“Ethylene, average (RER)| production”];
• Pyrolysis Ethylene (Somoza-Tornos et al. (2020)).
Once the impact of ethanol from plastic waste pyrolysis is calculated, it is compared to the impact of:
• Gasoline [“Petrol, unleaded (Europe without Switzerland) | petroleum refinery operation”].
• Bioethanol [“Ethanol, without water, in 99.7% solution state, from fermentation (Europe without Switzerland) | dewatering of ethanol from biomass, from 95 to 99.7% solution state”].
Results and Discussion
This section presents the results from the techno-economic and environmental assessment performed on diesel and gasoline alternatives, as well as a discussion on tradeoffs between all alternatives.
Techno-Economic Assessment
As described above, the study comprises two lines. First, the assessment of alternatives for diesel-based engines. Second, the comparison of gasoline alternatives, and then the assessment of possible blends.
Diesel Alternatives
Table 3 shows the cost breakdown and market prices for WPO, biodiesel, and diesel. It can be seen that the production of pure fossil-diesel and WPO are economically profitable (i.e., their production costs are below their market prices), while biodiesel production is not. This is due to the cost considered for biodiesel raw materials (mainly rapeseed oil, methanol/ethanol, and sodium hydroxide), which represents approximately 85% of production costs (22.90 €/GJ). This value is considerably higher than in the other two alternatives (9.81 €/GJ for PP waste and 13.06 €/GJ for crude oil). However, it is possible to obtain biodiesel using biomass waste or other non-edible biomass sources as feedstock, such as used cooking oil, waste animal fats, or other kinds of waste biomass, which are not assessed in this study.
It is worth noting that the calculated WPO total cost of 9.80 €/GJ (0.410 €/kg) is much larger than the cost of 1.07 €/GJ (0.045 €/kg) at the same plant scale (710 GJ/h), using household plastic waste estimated by Fivga and Dimitriou (2018). A conversion rate of 1.15 €/£ and the lower heating value of WPO of 0.04187 GJ/kg were used for this conversion. The difference stems from the assumed plastic waste feedstock cost (0.39 €/kg vs. 0 €/kg). This shows that the feedstock cost for plastic waste is a parameter that greatly influences the production cost and consequently the economic feasibility of the derived fuels. However, despite the conservative assumption taken in this work, WPO still stands as the most economically competitive alternative. Note that the price of plastic waste has been assumed to be equal to the cost of mixed plastic waste sorting and collection.
Figure 3A shows the range of blends between fossil diesel and biodiesel, and how blending affects the production costs and average market prices. According to these results, blends up to 50% v/v biodiesel are economically acceptable. This confirms that one of the most used biodiesel blends, B20 (total cost: 14.84 €/GJ vs. market price: 17.30 €/GJ), is still economically competitive overall, although the profit margin is significantly reduced compared to fossil diesel. Following the same procedure, production costs and market prices for blends of fossil diesel with WPO are estimated, leading to the results shown in Figure 3B. In this case, any blend would be economically profitable and the profit margin increases with the proportion of WPO used in the blend. A typical blend that could be used in current diesel vehicles is WPO30, which can be considered profitable from an economic standpoint.
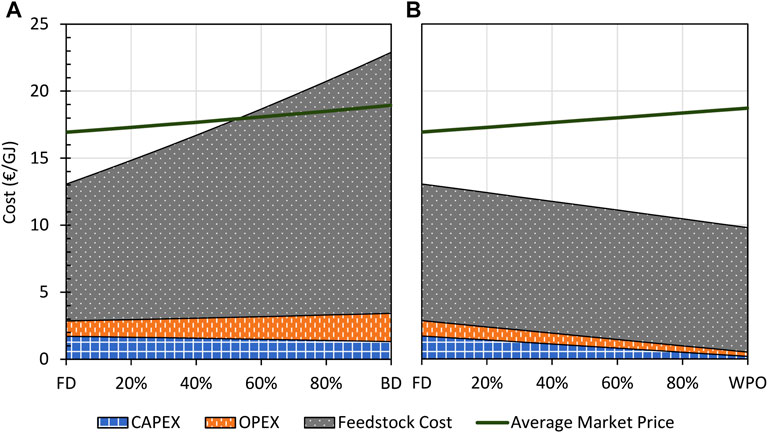
FIGURE 3. (A) Production cost breakdown for different blends of diesel and biodiesel and theoretical blend market price. Horizontal axis labels represent biodiesel weight percentage in the final blend. (B) Production cost breakdown for different blends of diesel and WPO and theoretical blend market price. Horizontal axis labels represent the WPO weight percentage in the final blend. FD: fossil diesel. BD: biodiesel. WPO: plastic waste pyrolysis oil (for references, see Table 3).
Figure 4 depicts the cost and market price comparison for traditional fossil diesel and two proposed blends with biodiesel and WPO. The blend with the best economic performance is WPO30 (total cost: 12.09 €/GJ vs. market price: 17.47 €/GJ) against fossil diesel (total cost: 13.06 €/GJ vs. market price: 16.94 €/GJ) and commercial blend with biodiesel (total cost: 14.84 €/GJ vs. market price: 17.30 €/GJ). The cost driver in these three cases is the feedstock cost.
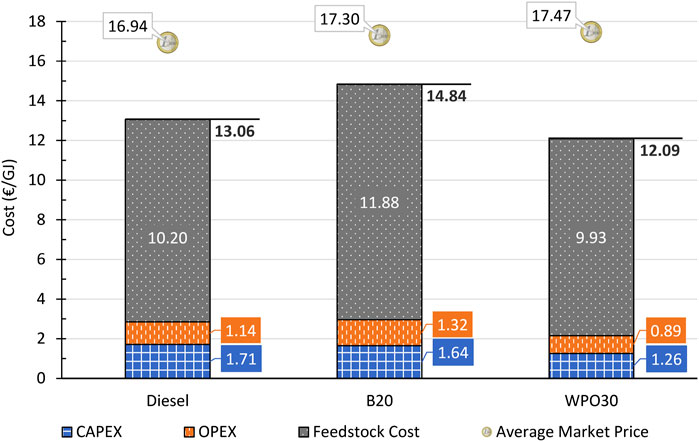
FIGURE 4. Economic comparison of traditional diesel against proposed blends with biodiesel (B20) and WPO (WPO30). B20: Diesel/Biodiesel blend 80/20% v/v. WPO30: Diesel/Plastic waste pyrolysis oils 70/30% v/v (for references, see Table 3).
From an economic point of view, the diesel and WPO standalone alternatives are profitable. Between these two alternatives, WPO is cheaper to produce. WPO is not a product available in the market; therefore, its price is estimated as equivalent to that for fossil diesel, which may add an important source of uncertainty to the study. However, its energy content is similar to fossil diesel, thus the price estimation is acceptable. Therefore, any blend between diesel and WPO would be economically profitable, with very similar results, and theoretically, a WPO100 would be the most profitable option.
Gasoline Alternatives
To assess the different fuel alternatives for gasoline engines, the production costs of gasoline are compared to European sourced bioethanol and plastic waste based ethanol. Cost breakdowns for these three routes are shown in Table 4, along with the reference market price for ethanol. Results show that the traditional way of obtaining bioethanol is economically competitive (total cost: 23.16 €/GJ vs. market price: 27.62 €/GJ). In contrast, the pyrolysis route is not competitive (total cost: 33.87 €/GJ). Capital and operational expenses are high due to complex and energy intensive gas separation and subsequent hydration steps.
Figure 5A shows the production cost breakdown and price for all tentative blends of gasoline and bioethanol. The results show that any blend would be economically competitive and feasible to use according to current market prices (17.67 €/GJ for gasoline and 27.62 €/GJ for ethanol), but the profit margin would grow slightly when the percentage of bioethanol is increased. Figure 5B depicts the production cost of blends of gasoline and WPE. In this case, WPE/gasoline blends are competitive up to approximately 40% v/v with a total cost equal to its market price of 20.60 €/GJ, showing that they would be feasible to implement for the unmodified engines at the recommended blending ratios (usually 25% v/v). Similar to the diesel case, in Figure 6, traditional gasoline is compared against two typically commercially available gasoline-bioethanol blends (E25 and E85), and an equivalent case is proposed using WPE instead (WPE25). Any of these alternatives would be economically feasible, but provided that pure bioethanol is the most profitable alternative, E85 is the most profitable bioethanol/gasoline blend (total costs: 23.16 and 21.36 €/GJ vs. market prices: 27.62 and 25.50 €/GJ respectively). To sum up, the larger the amount of bioethanol added to gasoline, the more economically profitable the blend would be, assuming the market price behaves as modeled herein.
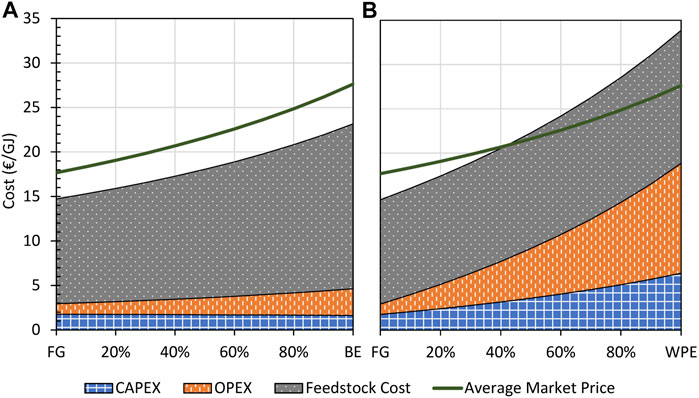
FIGURE 5. (A) Production cost breakdown for different blends of gasoline and bioethanol and theoretical blend market price. Horizontal axis labels represent bioethanol weight percentage in the final blend. (B) Production cost breakdown for different blends of gasoline and WPE and theoretical blend market price. Horizontal axis labels represent the WPE weight percentage in the final blend. FG: fossil gasoline. BE: bioethanol. WPE: plastic waste pyrolysis-sourced ethanol (for references, see Table 4).
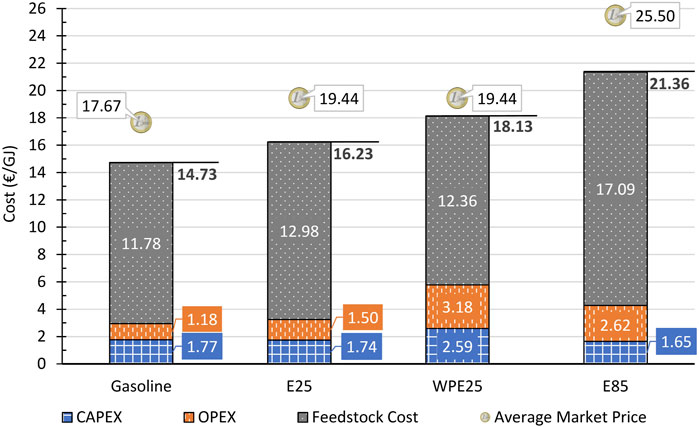
FIGURE 6. Economic comparison of traditional gasoline against commercial bioethanol blends and proposed WPE blend. E25: gasoline/bioethanol blend 75/25% v/v. WPE25: gasoline/plastic waste pyrolysis-sourced ethanol blend 75/25% v/v. E85: gasoline/bioethanol blend 15/85% v/v (for references, see Table 4).
Environmental Assessment
The environmental assessment is performed similarly to the economic assessment presented in the previous section. First, diesel alternatives are compared, and then the possible blends are evaluated. Subsequently, the ethanol obtained from two different routes are compared, and their blends with gasoline evaluated. They are assessed in terms of harmonized impact scores at midpoint and endpoint levels, which focus mainly on three areas: human health, ecosystem quality, and resource scarcity, as proposed in ReCiPe2016 methodology (Huijbregts et al., 2017). To facilitate the comparison and representation of the different impact scores, the endpoints and midpoints values are normalized with respect to the highest value among the alternatives.
To assess midpoint impacts, the focus is set on the ones driving the endpoints impacts results:
• The human health endpoint is mainly driven by global warming and fine particulate matter formation impacts.
• Ecosystems quality is driven by global warming, land use, and terrestrial acidification.
• Resource scarcity is led by fossil resource scarcity.
Diesel Alternatives
For the three analyzed diesel alternatives, Figure 7 depicts the LCA results on radar plots with normalized impact at midpoint and endpoint levels. As expected, the use of fossil diesel involves a higher impact on resource scarcity than any other alternative (13.04 vs. 3.54 and 0.31 USD2013/GJ for biodiesel and WPO respectively). Biodiesel, on the other hand, has a higher impact on human health and ecosystems (18.67 × 10−5 DALY/GJ and 17.58 × 10−7 species·yr vs. 3.50 × 10−5 DALY/GJ and 0.66 × 10−7 species·yr for diesel). By contrast, the production of WPO from polypropylene via the proposed process has a lower impact in all categories compared to the other two alternatives (0.31 USD2013/GJ, 1.6 × 10−5 DALY/GJ and 0.39 × 10−7 species yr). This is because waste is valorized, producing a valuable fuel and the process is comparatively simpler and environmentally friendlier.
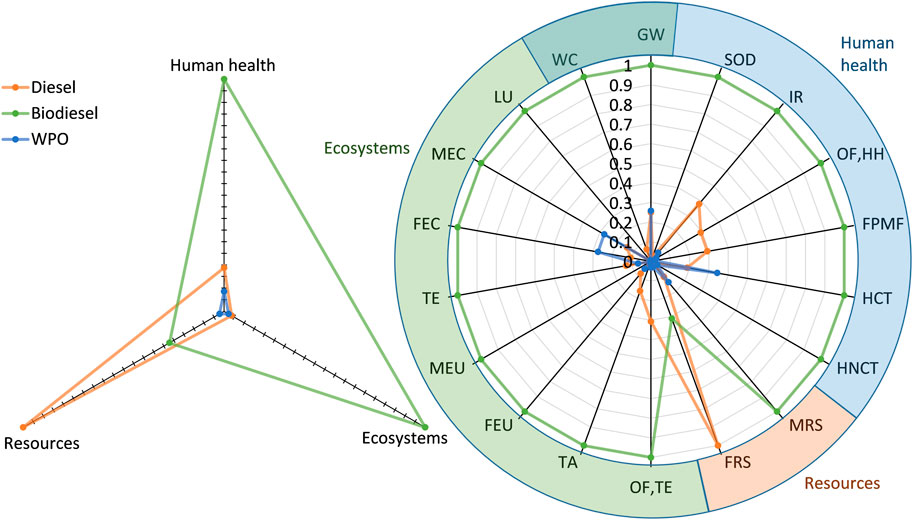
FIGURE 7. Normalized LCA endpoint and midpoint indicators comparison for diesel, biodiesel, and WPO production pathways. GW: global warming; SOD: stratospheric ozone depletion; IR: ionizing radiation; OF, HH: ozone formation, human health; FPMF: fine particulate matter formation; OF, TE: ozone formation, terrestrial ecosystems; TA: terrestrial acidification; FEU: freshwater eutrophication; MEU: marine eutrophication; TE: terrestrial ecotoxicity; FEC: freshwater ecotoxicity; MEC: marine ecotoxicity; HCT: human carcinogenic toxicity; HNCT: human non-carcinogenic toxicity; LU: land use; MRS: mineral resource scarcity; FRS: fossil resource scarcity; WC: water consumption. WPO: plastic waste pyrolysis oil. Endpoint and midpoint values are available in Supplementary Table S2.
The impact of biodiesel is significantly higher than for fossil-based diesel and WPO on human health and ecosystems, which are driven by global warming (55.34 vs. 12.15 and 12.70 kg equivalent carbon dioxide (CO2eq)/GJ respectively), fine particulate matter formation (136 × 10−3 vs. 35 × 10−3 and 2.4 × 10−3 kg PM2.5eq/GJ respectively), and land use (155.2 vs. 0.111 and 0.106 m2 crop eq/GJ respectively). On the other hand, fossil resource scarcity is considerably higher for fossil diesel (29.0 kg oil eq/GJ) than the other alternatives (10.21 kg oil eq/GJ for biodiesel and 0.90 kg oil eq/GJ for WPO).
It is important to highlight that, even though the biofuel alternative in principle closes the carbon cycle by depleting the CO2 from the atmosphere (1.98 kg CO2/kg rape seed) that is emitted during fuel combustion, its whole production phase entails considerable global warming impact and impact on ecosystems in general. These stem from intense land usage, water consumption and the use of fertilizers for harvesting energy crops. A significant amount of global warming impact comes from dinitrogen monoxide (N2O) emissions that have a 298 times higher greenhouse gas effect than CO2 (Solomon et al., 2007). First-generation fuel feedstock in principle entails an additional carbon debt from cutting down forests and grasslands that act as carbon storage, which will eventually be released over a period of time. This kind of carbon debt is referred to as land use change and is considered a major source of emission in the life cycle of first-generation fuels. Adding this impact to the assessment leads to most first-generation fuels having higher global warming potential than fossil fuels (Jeswani et al., 2020).
Gasoline Alternatives
Figure 8 shows the endpoint and midpoint indicators for the three proposed ways of obtaining ethanol and gasoline. In the same line to the findings for diesel alternatives, the biomass-sourced ethanol has a higher impact on human health and ecosystems than the other alternatives and the lowest on resources (10.14 × 10−5 DALY/GJ, 6.19 × 10−7 species·yr and 2.31 USD2013/GJ). Gasoline has the highest impact on resources but lowest on the other two endpoints (4.70 × 10−5 DALY/GJ, 0.90 × 10−7 species·yr and 13.93 USD2013/GJ) and pyrolysis-sourced ethanol have intermediate impact values on human health, ecosystems, and resources (5.81 × 10−5 DALY/GJ, 1.45 × 10−7 species·yr and 9.00 USD2013/GJ).
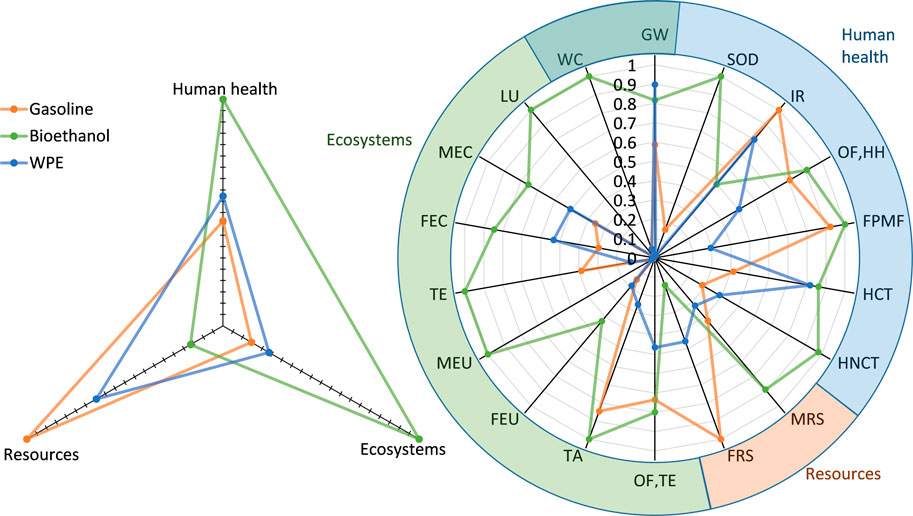
FIGURE 8. Normalized LCA endpoint and midpoint indicators comparison for gasoline, bioethanol and WPE production pathways. GW: global warming; SOD: stratospheric ozone depletion; IR: ionizing radiation; OF,HH: ozone formation, human health; FPMF: fine particulate matter formation; OF,TE: ozone formation, terrestrial ecosystems; TA: terrestrial acidification; FEU: freshwater eutrophication; MEU: marine eutrophication; TE: terrestrial ecotoxicity; FEC: freshwater ecotoxicity; MEC: marine ecotoxicity; HCT: human carcinogenic toxicity; HNCT: human non-carcinogenic toxicity; LU: land use; MRS: mineral resource scarcity; FRS: fossil resource scarcity; WC: water consumption. WPE: plastic waste pyrolysis-sourced ethanol. Endpoint and midpoint values are available in Supplementary Table S3.
When compared to gasoline, bioethanol has a considerably higher impact on human health and ecosystems and a lower impact on resources. WPE shows a similar tendency but smaller difference with gasoline, that is, slightly higher impact on human health and ecosystems and slightly lower on resources.
The highest impact on global warming corresponds to WPE and bioethanol (41.95 and 38.14 vs. 17.78 kg CO2eq/GJ respectively for WPE, bioethanol, and gasoline), additionally, the highest impact on fine particulate matter formation corresponds to bioethanol (75 × 10−3 vs. 45 × 10−3 and 22 × 10−3 kg PM2.5eq/GJ respectively for bioethanol, gasoline, and WPE), which drives it to the highest human health endpoint impact among the other alternatives. The highest impact on ecosystems corresponds to bioethanol, driven mostly by its high impact on land use (42.6 vs. 0.23 and 0.14 m2 crop eq/GJ respectively for WPE and gasoline). On the other hand, fossil resource scarcity is higher on gasoline (31.1 vs. 22.1 and 7.2 kg oil eq/GJ respectively for WPE and bioethanol).
Remarks on Engine Performance
After the production phase, the fuels enter the use phase where they generate an additional impact on the environment. Engine tests for different blends at varying operating conditions are commonly used to identify the performance and emission values of fuel in a test engine.
In the case of diesel, biodiesel, and WPO the literature findings vary vastly from study to study. Adaileh and Alqdah (2012) report that biodiesel obtained from waste cooking oil provided a significant reduction in carbon monoxide and unburned hydrocarbons, but increases in nitrogen oxide emissions and brake-specific fuel consumption. Similar differences can be observed in the case of WPO, as described in the review by Damodharan et al. (2019) and more recent studies by Singh et al. (2020) and Singh et al. (2021).
The differences observed between biodiesel and WPO engine performance can be explained by the different composition and properties of the fuels under study, stemming from different feedstock types and quality, the catalyst used during conversion, experimental procedure, etc. As previously mentioned, more in-depth studies are required to assess the final environmental impact for each case individually.
Bioethanol is commonly found to enhance engine performance while also reducing emission values (Thangavelu, 2016). This implies that the inclusion of the use phase would favor ethanol over gasoline in terms of environmental impact. There is a consensus that ethanol fuel has no considerable variations in composition from study to study.
Economic and Environmental Tradeoffs
In order to devise the potential of each of the alternatives and their potential blends, a tradeoff evaluation has been performed attending to economic and environmental results. First, diesel alternatives and second, gasoline alternatives were studied, and the resulting proposals are discussed from a global point of view.
Diesel Alternatives
Considering global economic and environmental performance, any blending of diesel and WPO would be more profitable than fossil diesel alone, and any biodiesel/diesel blend. Indeed, WPO appears to be a very promising alternative, with outstanding performance in comparison to biodiesel as a diesel alternative, and it is recommended that the highest blending ratio that internal combustion engines can admit are used (without compromising performance or stability).
Gasoline Alternatives
Bioethanol has better economic performance than any other alternative considered in this study. Regarding gasoline/ethanol blends, bioethanol is economically competitive at any ratio because of the specific market prices of these blends, while WPE should not exceed 40% v/v to ensure economic feasibility. From the environmental point of view, WPE shows better overall results than other choices and blends with gasoline would entail a lower impact on human health and ecosystems than gasoline-bioethanol blends.
To find a tradeoff between economic and environmental performance, and considering typical blending ratios (25% v/v), WPE seems to be a good choice. When compared individually to gasoline, its environmental impact on human health is 20% lower, while for bioethanol it is 40% higher; on ecosystems, its impact is 5% higher while for bioethanol it is 350% higher; and on resources, both WPE and bioethanol have a lower impact than gasoline (58 and 89% lower respectively). In reverse, the profit margin of WPE25 vs. E25 would be around 59% lower.
Global Analysis of Alternatives
From a global perspective and bearing in mind the kinds of fuel considered in this work, one alternative could potentially be devised as better than the rest. The results show that the use of WPO as a fuel is a very promising option to replace fossil fuels and biofuels, since it has shown the best economic and environmental results among the considered options. Although it is important to underline that this technology is not yet as developed as the rest and it needs to be scaled up.
Conclusion
This work presents an overview and assessment of the most widely used fuel sources for “hard-to-decarbonize” sectors and compares them with emerging solutions. On one hand, biofuels have gained wide attention due to their potential to close the carbon cycle and preserve fossil resources. However, first-generation biofuels can have a considerable impact on ecosystems and human health, as has been confirmed in this study for the case of biodiesel and bioethanol in Europe. On the other hand, fuels derived from plastic waste sources are gaining increasing interest due to their capability to displace the problem of waste management. The comparative techno-economic and life cycle assessment between fossil-, bio- and plastic waste pathways in this study yield the following insights:
• A contribution is made to waste-to-resource modeling through the simulation of a low temperature waste pyrolysis process of polypropylene.
• Techno-economic assessment reveals that the production (Well-to-Tank) of oils derived from waste polypropylene (namely WPO) are more economically competitive (9.80 vs 13.06 €/GJ for diesel and 22.90 €/GJ for biodiesel).
• Life cycle assessment shows that WPO performs better in all three ReCiPe2016 impact categories (ecosystems, human health, and resources) than the fossil and biodiesel alternatives due to its low emissions stemming from procedural simplicity and low energy demand.
• Converting the pyrolysis products of polyethylene into high purity ethanol (WPE) to be used in gasoline engines is economically non-competitive (33.8 €/GJ) compared to bioethanol (23.2 €/GJ) and fossil gasoline itself (14.7 €/GJ). This comes from the more complex processing including the separation of gaseous pyrolysis products and subsequent catalytic hydration.
• Similar to biodiesel, bioethanol performs worse in ecosystems and human health but better in resources than fossil gasoline for the aforementioned reasons. As opposed to WPO, WPE production is more complex and energy intensive, thus, it is placed in between gasoline and bioethanol in all categories.
Based on these findings, the products from plastic waste pyrolysis appear as a very promising alternative and, more specifically, the use of PP waste pyrolysis oil (WPO) as a diesel substitute shows great potential to partially replace fossil fuels. Additionally, it would help to deal with the growing problem of waste disposal. WPE, on the other hand, is more costly to produce and less environmentally friendly due to the additional separation and hydration steps. Although it offers a certain profit margin and tradeoff solutions in the impact categories between gasoline and bioethanol, it should be considered only if the conversion from a valuable chemical product (ethylene) to ethanol for the sake of fuel production is reasonable. Thus, research efforts should focus on the direct utilization of plastic waste oils in engines due to the aforementioned reasons. Real data from emerging commercial projects should be collected and assessed to further validate the findings of this study.
Future work must consider the extension of the system boundaries to the use phase of the alternative fuels since fuel quality can vary heavily depending on the used feedstock. Moreover, material and energy balances, thus costs and impacts will change depending on the type and quality of plastic waste. Accordingly, not only alternative feedstock for plastics, but also biomass based pathways might be included in the assessment, with a special focus on identifying weak spots to increasing economic competitiveness and the environmental friendliness of each alternative.
Data Availability Statement
The original contributions presented in the study are included in the article/Supplementary Material, further inquiries can be directed to the corresponding author.
Author Contributions
Conceptualization: AS-T, AP-L, FL; Methodology: AS-T, AP-L, FL; Software: AS-T, FL; Validation: MG, AE; Formal Analysis: AS-T, AP-L, FL; Investigation: AS-T, AP-L, FL; Resources: AS-T, AP-L, FL; Data Curation: AS-T, AP-L, FL; Writing—Original Draft: AS-T, AP-L, FL; Writing—Review and Editing: AP-L, FL; Visualization: AP-L, FL; Supervision: MG, AE; Project Administration: MG, AE; Funding Acquisition: MG, AE.
Funding
This study received financial support from the Spanish Competitiveness, Industry, and Economy Ministry and the European Regional Development Fund, both funding the research Project AIMS (DPI 2017-87435-R).
Conflict of Interest
The authors declare that the research was conducted in the absence of any commercial or financial relationships that could be construed as a potential conflict of interest.
Acknowledgments
AP-L thankfully acknowledges the financial support of the Spanish Ministry of Science, Innovation, and Universities (grant ref. PRE 2018-087135). FL gratefully acknowledges the Universitat Politècnica de Catalunya and Banco Santander for the financial support of his predoctoral grant FPI-UPC. Figure 1 was made with icons designed by Freepik, Smashicons and DinosoftLabs from www.flaticon.com.
Supplementary Material
The Supplementary Material for this article can be found online at: https://www.frontiersin.org/articles/10.3389/fenrg.2021.676233/full#supplementary-material
Abbreviations
B0-B100, Blends of biodiesel with fossil diesel. The number indicates the volumetric percentage of biodiesel; CEPCI, Chemical Engineering Plant Cost Index; E0-E100, Blends of bioethanol with fossil gasoline. The number indicates the volumetric percentage of bioethanol; LCA, Life Cycle Assessment; PE, Polyethylene; PP, Polypropylene; TTW, Tank-to-Wheels; WPE, Plastic Waste Pyrolysis Ethanol; WPE0-WPE100, Blends of WPE with fossil gasoline. The number indicates the volumetric percentage of WPE; WPO, Plastic Waste Pyrolysis Oil; WPO0-WPO100, Blends of WPO with fossil diesel. The number indicates the volumetric percentage of WPO; WTT, Well-to-Tank; WTW, Well-to-Wheels.
References
ACEA (2020). Fuel Types of New Cars. European Automobile Manufacturers’ Association. Available at: https://www.acea.be/press-releases/article/fuel-types-of-new-cars-petrol-52.3-diesel-29.9-electric-6.8-market-share-fi (Accessed March 1, 2021)
Adaileh, W. M., and Alqdah, K. S. (2012). Performance of Diesel Engine Fuelled by a Biodiesel Extracted from a Waste Cocking Oil. Energ. Proced. 18, 1317–1334. doi:10.1016/j.egypro.2012.05.149
Alleman, T. L., McCormick, R. L., Christensen, E. D., Fioroni, G., Moriarty, K., and Yanowitz, J. (2016). Biodiesel Handling and Use Guide. 5th Edn, Golden, Colorado; Cincinnati, OH, United States: National Renewable Energy Laboratory; Eco Engineering. doi:10.2172/1332064
Araujo Galvão, G. D., De Nadae, J., Honorato Clemente, D., Chinen, G., and de Carvalho, M. M. (2018). Circular Economy: Overview of Barriers. Proced. CIRP 73, 79–85. doi:10.1016/j.procir.2018.04.011
Ayaou, B., Bodson, A., Dehottay, L., Farai, R., Farcy, A., Hendrickx, D., et al. (2020). Ethanol Production by Catalytic Hydration of Ethylene, Liege, Belgium: Liège Université Library Press, 1–21.
Brinkman, N., Wang, M., Weber, T., and Arlington, T. (2005). Well-to-Wheels Analysis of Advanced Fuel/Vehicle Systems - A North American Study of Energy Use, Greenhouse Gas Emissions, and Criteria Pollutant Emissions. Energy, no. May. Available at: http://www.transportation.anl.gov/pdfs/TA/339.pdf (Accessed April 18, 2021). doi:10.2172/1218344
Canilha, L., Chandel, A. K., Dos Santos. Milessi, T. S., Fernandes Antunes, F. A., Antunes, F. A. F., da Costa Freitas, W. L., et al. (2012). Bioconversion of Sugarcane Biomass into Ethanol: An Overview about Composition, Pretreatment Methods, Detoxification of Hydrolysates, Enzymatic Saccharification, and Ethanol Fermentation. J. Biomed. Biotechnol. 2012, 1–15. doi:10.1155/2012/989572
Chandran, M., Tamilkolundu, S., and Murugesan, C. (2020). Characterization Studies: Waste Plastic Oil and its Blends. Energy Sourc. A: Recovery, Utilization, Environ. Effects 42 (3), 281–291. doi:10.1080/15567036.2019.1587074
Dahlbo, H., Poliakova, V., Mylläri, V., Sahimaa, O., and Anderson, R. (2018). Recycling Potential of Post-Consumer Plastic Packaging Waste in Finland. Waste Manage. 71, 52–61. doi:10.1016/j.wasman.2017.10.033
Damodharan, D., Rajesh Kumar, B., Gopal, K., De PouresDe Poures, M. V., and Sethuramasamyraja, B. (2019). Utilization of Waste Plastic Oil in Diesel Engines: A Review. Rev. Environ. Sci. Bio/Technol. 18, 681–697. doi:10.1007/s11157-019-09516-x
Edwards, R., Larivé, J. F., Rickeard, D., and Werner, W. (2014). WELL-TO-TANK (WTT) Report. Version 4a. Ispra, Italy: Joint Research Center of the EU (JRC). doi:10.2790/95629
Edwards, R., Larive, J. F., Vincent, M., and Rounveirolles, P. (2007). WELL-TO-WHEELS ANALYSIS of FUTURE AUTOMOTIVE FUELS and WELL-To-WHEELS Report. Europe Version 2c (March): 88. Available at: www.co2star.eu/publications/Well_to_Tank_Report_EU.pdf (Accessed February 22, 2021).
European Commission (2020). EU Agricultural Outlook for Markets, Income and Environment 2020 - 2030, Brussels: European Commission, DG Agriculture and Rural Development Available at: https://ec.europa.eu/info/sites/default/files/food-farming-fisheries/farming/documents/agricultural-outlook-2020-report_en.pdf (Accessed April 22, 2020). doi:10.2762/252413
European Commission (2021). Weekly Oil Bulletin | Energy. Available at: https://ec.europa.eu/energy/data-analysis/weekly-oil-bulletin_en (Accessed February 22, 2021).
Eurostat - European Commission (2020). Statistical Classification of Economy Activity in the European Union (NACE 2). 2019 Annual Detailed Data by PRODCOM List. Available at: http://ec.europa.eu/eurostat/web/prodcom/data/excel-files-nace-rev.2 (Accessed November 26, 2020).
Fivga, A., and Dimitriou, I. (2018). Pyrolysis of Plastic Waste for Production of Heavy Fuel Substitute: A Techno-Economic Assessment. Energy 149 (April), 865–874. doi:10.1016/j.energy.2018.02.094
Fuels Europe (2018). Statistical Report. Available at: https://www.fuelseurope.eu/wp-content/uploads/FuelsEurope-Statistical-Report-2018.pdf (Accessed February 28, 2021).
Gentil, E. C., Damgaard, A., Hauschild, M., Finnveden, G., Eriksson, O., Thorneloe, S., et al. (2010). Models for Waste Life Cycle Assessment: Review of Technical Assumptions. Waste Manage. 30 (12), 2636–2648. doi:10.1016/j.wasman.2010.06.004
Glitman, K., Farnsworth, D., and Hildermeier, J. (2019). The Role of Electric Vehicles in a Decarbonized Economy: Supporting a Reliable, Affordable and Efficient Electric System. Electricity J. 32 (7), 106623. doi:10.1016/j.tej.2019.106623
Goedkoop, M., Ponsioen, T., and Meijer, E. (2014). Introduction to LCA with SimaPro. Available at: https://www.pre-sustainability.com/introduction-to-lca (Accessed December 16, 2020).
Gonzalez-Garay, A., Gonzalez-Miquel, M., and Guillen-Gosalbez, G. (2017). High-Value Propylene Glycol from Low-Value Biodiesel Glycerol: A Techno-Economic and Environmental Assessment under Uncertainty. ACS Sustain. Chem. Eng. 5 (7), 5723–5732. doi:10.1021/acssuschemeng.7b00286
Gray, N., McDonagh, S., O'Shea, R., Smyth, B., and Murphy, J. D. (2021). Decarbonising Ships, Planes and Trucks: An Analysis of Suitable Low-Carbon Fuels for the Maritime, Aviation and Haulage Sectors. Adv. Appl. Energ. 1 (February), 100008. doi:10.1016/j.adapen.2021.100008
Herrmann, R., Jumbe, C., Bruentrup, M., and Osabuohien, E. (2018). Competition between Biofuel Feedstock and Food Production: Empirical Evidence from Sugarcane Outgrower Settings in Malawi. Biomass Bioenergy 114 (July), 100–111. doi:10.1016/j.biombioe.2017.09.002
Huijbregts, M. A. J., Steinmann, Z. J. N., Elshout, P. M. F., Stam, G., Verones, F., Vieira, M., et al. (2017). ReCiPe2016: A Harmonised Life Cycle Impact Assessment Method at Midpoint and Endpoint Level. Int. J. Life Cycle Assess. 22 (2), 138–147. doi:10.1007/s11367-016-1246-y
Ilves, R., Küüt, A., and Olt, J. (2019). Ethanol as Internal Combustion Engine Fuel. Ethanol: Sci. Eng., 215–229. doi:10.1016/B978-0-12-811458-2.00008-0
International Renewable Energy Agency (2013). Road Transport: The Cost of Renewable Solutions. IRENA Report, Preliminary Findings. Available at: https://irena.org/-/media/Files/IRENA/Agency/Publication/2013/Road_Transport.pdf. (Accessed February 25, 2020).
ISO (2016). ISO 14040:2006 - Environmental Management — Life Cycle Assessment — Principles and Framework. Available at: https://www.iso.org/standard/37456.html (Accessed March 1, 2021).
Jenkins, S. (2020). Chemical Engineering Plant Cost Index. Chemical Engineering. Available at: https://www.chemengonline.com/pci-home (Accessed April 18, 2021).
Jeswani, H. K., Chilvers, A., and Azapagic, A. (2020). Environmental Sustainability of Biofuels: A Review. Proc. R. Soc. A 476, 20200351. doi:10.1098/rspa.2020.0351.
Kannan, P., Al Shoaibi, A., and Srinivasakannan, C. (2014). Temperature Effects on the Yield of Gaseous Olefins from Waste Polyethylene via Flash Pyrolysis. Energy Fuels 28 (5), 3363–3366. doi:10.1021/ef500516n
Kavalov, B. (2005). Biofuel potentials in the EU. European Commission, Directorate-General Joint Research Centre, Institute for Prospective Technological Studies (IPTS), EUR 21012 EN. Available at: http://ftp.jrc.es/EURdoc/eur21012en.pdf (Accessed April 18, 2021).
Lopez, G., Artetxe, M., Amutio, M., Bilbao, J., and Olazar, M. (2017). Thermochemical Routes for the Valorization of Waste Polyolefinic Plastics to Produce Fuels and Chemicals. A Review. Renew. Sustain. Energ. Rev. 73 (November 2016), 346–368. doi:10.1016/j.rser.2017.01.142
Mangesh, V. L., Padmanabhan, S., Tamizhdurai, P., and Ramesh, A. (2020). Experimental Investigation to Identify the Type of Waste Plastic Pyrolysis Oil Suitable for Conversion to Diesel Engine Fuel. J. Clean. Prod. 246, 119066. doi:10.1016/j.jclepro.2019.119066
Miandad, R., Barakat, M. A., Aburiazaiza, A. S., Rehan, M., and Nizami, A. S. (2016). Catalytic Pyrolysis of Plastic Waste: A Review. Process Saf. Environ. Prot. 102, 822–838. doi:10.1016/j.psep.2016.06.022
Organisation for Economic Co-operation and Development (2020). OECD Statistics (OECD-FAO Agricultural Outlook 2020-2029 - EU - Biofuels Available at: https://stats.oecd.org/. (Accessed April 22, 2021).
Pacheco-López, A., Somoza-Tornos, A., Muñoz, E., Capón-García, E., Graells, M., and Espuña, A. (2020). Synthesis and Assessment of Waste-To-Resource Routes for Circular Economy. Computer Aided Chem. Eng. 48, 1933–1938. doi:10.1016/B978-0-12-823377-1.50323-2
Pahl, G. (2009). Biodiesel: Growing a New Energy Economy. Choice Reviews Online Vol. 46, 46-3271. doi:10.5860/choice.46-3271
SABIC (2019). Sustainable Developent Goals Roadmap. Available at: https://www.sabic.com/assets/en/Images/Sustainable-Development-Goals-2019-Roadmap_tcm1010-21094.pdf (Accessed March 1, 2021).
Sharma, S., and Ghoshal, S. K. (2015). Hydrogen the Future Transportation Fuel: From Production to Applications Renew. Sustain. Energ. Rev. 43, 1151–1158. doi:10.1016/j.rser.2014.11.093
Singh, R. K., Ruj, B., Sadhukhan, A. K. P., Gupta, P., and Tigga, V. P. (2020). Waste Plastic to Pyrolytic Oil and its Utilization in CI Engine: Performance Analysis and Combustion Characteristics. Fuel 262 (November 2019), 116539. doi:10.1016/j.fuel.2019.116539
Singh, T. S., Rajak, U., Dasore, A., Muthukumar, M., and Verma, T. N. (2021). Performance and Ecological Parameters of a Diesel Engine Fueled with Diesel and Plastic Pyrolyzed Oil (PPO) at Variable Working Parameters. Environ. Tech. Innovation 22, 101491. doi:10.1016/j.eti.2021.101491
Sinnott, R., and Towler, G. (2020). Costing and Project Evaluation. Chem. Eng. Des., 275–369. doi:10.1016/b978-0-08-102599-4.00006-0
Solomon, S. D., Qin, M., Manning, R. B., Alley, T., Berntsen, N. L., and Bindoff, N. L. (2007). Technical Summary. in Climate Change 2007: The Physical Science Basis. Contribution of Working Group I to the Fourth Assessment Report of the Intergovernmental Panel on Climate Change, Cambridge, United Kingdom; New York, NY, USA: Cambridge University PressAvailable at: https://www.ipcc.ch/site/assets/uploads/2018/05/ar4_wg1_full_report-1.pdf. (Accessed April 1, 2021)
Somoza-Tornos, A., Gonzalez-Garay, A., Pozo, C., Graells, M., Espuña, A., and Guillén-Gosálbez, G. (2020). Realizing the Potential High Benefits of Circular Economy in the Chemical Industry: Ethylene Monomer Recovery via Polyethylene Pyrolysis. ACS Sustain. Chem. Eng. 8 (9), 3561–3572. doi:10.1021/acssuschemeng.9b04835
Somoza-Tornos, A., Pozo, C., Graells, M., Espuña, A., and Puigjaner, L. (2021). Process Screening Framework for the Synthesis of Process Networks from a Circular Economy Perspective. Resour. Conservation Recycling 164 (August 2020), 105147. doi:10.1016/j.resconrec.2020.105147
Thangavelu, S. K., Ahmed, A. S., and Ani, F. N. (2016). Review on Bioethanol as Alternative Fuel for Spark Ignition Engines. Renew. Sustain. Energ. Rev. 56, 820–835. doi:10.1016/j.rser.2015.11.089
U.S. Energy Information Administration (2019a). Diesel Prices and Outlook. Available at: https://www.eia.gov/energyexplained/diesel-fuel/prices-and-outlook.php (Accessed February 23, 2021).
U.S. Energy Information Administration (2019b). Factors Affecting Gasoline Prices. Available at: https://www.eia.gov/energyexplained/gasoline/factors-affecting-gasoline-prices.php (Accessed February 23, 2021).
Wernet, G., Bauer, C., Steubing, B., Reinhard, J., Moreno-Ruiz, E., and Weidema, B. (2016). The Ecoinvent Database Version 3 (Part I): Overview and Methodology. Int. J. Life Cycle Assess. 21 (9), 1218–1230. doi:10.1007/s11367-016-1087-8
Keywords: techno-economic assessment, life cycle assessement, plastic waste management, biofuels, energy sustainability, pyrolysis oil, transport decarbonization
Citation: Pacheco-López A, Lechtenberg F, Somoza-Tornos A, Graells M and Espuña A (2021) Economic and Environmental Assessment of Plastic Waste Pyrolysis Products and Biofuels as Substitutes for Fossil-Based Fuels. Front. Energy Res. 9:676233. doi: 10.3389/fenrg.2021.676233
Received: 04 March 2021; Accepted: 06 May 2021;
Published: 24 June 2021.
Edited by:
Valerie Eveloy, Khalifa University, United Arab EmiratesReviewed by:
Tuan Amran Tuan Abdullah, University of Technology Malaysia, MalaysiaThokchom Subhaschandra Singh, National Institute of Technology, Manipur, India
Copyright © 2021 Pacheco-López, Lechtenberg, Somoza-Tornos, Graells and Espuña. This is an open-access article distributed under the terms of the Creative Commons Attribution License (CC BY). The use, distribution or reproduction in other forums is permitted, provided the original author(s) and the copyright owner(s) are credited and that the original publication in this journal is cited, in accordance with accepted academic practice. No use, distribution or reproduction is permitted which does not comply with these terms.
*Correspondence: Antonio Espuña, YW50b25pby5lc3B1bmFAdXBjLmVkdQ==
†These authors have contributed equally to this work and share first authorship