- 1Institute for Chemistry and Technology of Materials, Graz University of Technology (NAWI Graz), Graz, Austria
- 2Alistore – ERI European Research Institute, CNRS FR3104, Amiens, France
Fast Li+ solid ion conductors are a key component of all-solid-state batteries, a technology currently under development. The possible use of metallic lithium as active material in solid-state batteries warrants a quantum step improvement of battery specific energy, enabling further electric vehicles application. Hereby, we report the synthesis and ion conduction properties of a new solid hybrid electrolyte based on the MIL-121 metal organic framework (MOF) structure. After an ion exchange procedure that introduces Li+ in the structure, a known quantity of a soaking electrolyte is incorporated. The soaking electrolyte is based on the EMIM-TFSI ionic liquid, thus we can classify our formulation as a MOF–ionic liquid hybrid solid electrolyte. Electrical conductivity is investigated by impedance spectroscopy and preliminary studies of ion dynamics are conducted by 7Li NMR. The field of MOF-based ion conductors remains in incipient stages of research. Our report paves the way towards the rational design of new solid-state ion conductors.
1 Introduction
Energy storage is one of the key technological challenges of our society. Unsurprisingly, many research efforts are oriented towards advanced battery systems. (Kim et al., 2019). One critical component of electrochemical systems and devices is the electrolyte. Electrolytes must be conductive for electroactive species, such as Li+, and insulating with respect to electrons. There are major interests in finding solid electrolytes with high conductivity; (Zhang et al., 2018; Zhao et al., 2019); on the long term they are considered better alternatives to the current liquid electrolytes used in commercial Li-ion battery systems.
The motivation is at least two-fold. First, the current commercial liquid electrolyte formulations are flammable, which may lead to dangerous situations in case of an electrolyte leak. A solid electrolyte would obviously eliminate leakage problems and would be safer, although safety largely depends on the type of compound used. (Chung and Kang, 2017; Chen et al., 2020). Second, the use of metallic lithium as an active material would be desired as a high capacity replacement for graphite. Graphite has reached its maximum technological development limit. Higher capacity materials would be required to further improve the specific energy of batteries. In spite of 40 years of research, it remains hitherto impractical to use Li metal anodes with liquid electrolytes in rechargeable lithium cells. Li dendrite growth and pulverisation of the anode upon repeated stripping and plating cycles are preventing the use of metallic lithium in batteries with liquid electrolyte. (Zheng J. et al., 2020).
Nowadays, much hope is put on Li+ conducting solid electrolytes and composites; this is a highly dynamic and rapidly expanding field. (Zheng Y. et al., 2020). Solid electrolytes are expected to prevent lithium dendrite growth; yet it seems that this is not an a priori property of solid electrolytes. (Porz et al., 2017). However, with careful engineering and keeping the current density low, solid electrolytes can at least alleviate the issue of lithium dendrite growth, if not eliminating it completely. Finding suitable solid lithium ion conductors remains a challenging task, although there are several compounds showing high conductivity; the list is currently expanding. (Zheng et al., 2018).
Metal–organic frameworks (MOF), also called coordination polymers, are a class of microporous organic-inorganic compounds developed initially for their gas adsorption properties, especially for catalysis and for separation technologies. Since then, the range of MOF applications has been significantly extended. A MOF structure is built of metal centres or metal clusters connected to a bi- or multidentate organic molecule called a linker. The metal centres and the linkers may form 2D or 3D structures having long range crystalline ordering, very often with a well-defined pore size and a high specific surface area. One of the key characteristics of MOF structures is the large choice of ligands that can be used to build MOFs. The size and chemical structure of the MOF linker will determine the crystal structure as well as the shape and the volume of the pores. The chemical functionality of the ligand offers many possibilities for tuning the MOF’s physical and chemical properties. In addition, the number of combinations between suitable metal centres and linkers is enormous. Thanks to these features, MOFs emerged as a development platform currently used to create, to explore and to adjust functional materials.
Lithium ion conductors based on metal organic framework (MOF) structures are a relatively new research field. While MOF proton conductors are known for a while, (Sadakiyo and Kitagawa, 2021), the first Li+ MOF ion conductor was found only 10 years ago (Wiers et al., 2011). Although further developments were reported, (Ameloot et al., 2013; Park et al., 2017; Miner et al., 2019), the field of MOF Li+ conductors is in its infancy and there are plenty of possibilities to explore. One characteristic of the few MOF ion conductors reported so far, is the use of a soaking electrolyte or small solvent molecules that fill the pores of the MOF ion conductor. As the pores of MOF materials tend to be large with respect to the small size of Li+ ions, a small Li+ solvating molecule is necessary to enhance Li+ mobility. However, only few solvent molecules may actually reside in a typical MOF cage or pore as the space is very limited in a nanopore. Therefore, one cannot describe these small molecules embedded in the pores as a liquid phase; a closer description would be that of adsorbed molecules confined in the pores. Unfortunately, adsorption of chemical species on MIL-121 is an aspect more complicated than for other MOFs. Not all species may be adsorbed in the MIL-121 structure, even if they are certainly small. For instance, specific surface area determination of the MIL-121 by the Brunauer-Emmett-Teller (BET) method using nitrogen adsorption shows a very low specific surface area, simply because the non-polar N2 molecules cannot properly enter the pores that are obstructed by the free carboxylic units. (Chen et al., 2019). Nevertheless, it is not quite clear how the electric dipole moment of the adsorbing species influences the adsorption properties of MIL-121.
In a recent publication, we reported the functionalization of the MIL-121 MOF (Zettl et al., 2021) with Li+ and Na+ by ion exchange. With a soaking electrolyte containing propylene carbonate (PC) solvent, that is present in the pores in the nanofluid (or adsorbed) form mentioned above, we reached high conductivities for both Li+ and Na+. While the PC proved to be an excellent solvent that significantly increases Li+ mobility in the MOF structure, it also has a low, yet not negligible, vapour pressure and it is susceptible to slow evaporation from the structure. This property may raise additional issues during manufacturing, storage and operation of this hybrid solid electrolyte.
In this report we investigate the use of an ionic liquid, namely 1-ethyl-3-methylimidazolium bis(trifluoromethylsulfonyl)imide (EMIM-TFSI) as a soaking electrolyte in a MIL-121 MOF that was functionalized with Li+ cations. Ionic liquids are known for their extremely low vapour pressure, EMIM-TFSI is no exception. We hereby report on the ionic conduction properties of these materials and we take a glimpse into the ion dynamics of these materials by 7Li nuclear magnetic resonance (NMR) spectroscopy.
2 Materials and Methods
Lithiated MIL-121 was prepared by an ion exchange reaction with lithium acetate (LiAc). The solution was stirred overnight in a closed container. Details about the synthesis of MIL-121 and the post-synthetic modification with LiAc can be found elsewhere. (Zettl et al., 2021). Before the soaking electrolyte was added, all samples were activated at 300°C at a pressure of 10−3 mbar for 24 h. Elemental analysis was carried out using inductively coupled plasma mass spectrometry (ICPMS, Agilent 7700); we used a microwave heated pressurized autoclave digestion system (Ultraclave IV, MLS ICPMS 7700x Agilent Technologies). An aliquot (0.02 g) of the rinsed and dried (see above) MIL-121/Li sample was mixed with 5 ml HNO3 heated in a MLS UltraClave. The temperature program consisted of a ramp, heating the sample in 30 min from room temperature to 250°C and then holding for 30 min at 250°C. For the analysis, the samples were further diluted. The results are shown in Supplementary Table S1 (see Supplementary Material).
Soaking of MIL-121/Li was done with a 0.4 M solution of LiTFSI (lithium bis(trifluoromethanesulfonyl)imide) in EMIM-TFSI (1-ethyl-3-methylimidazolium-bis(trifluormethylsulfonyl)imide). EMIM-TFSI ionic liquid is further abbreviated as IL. The addition of the soaking electrolyte was carried out on the ion exchanged MOF in powder form, followed by vigorous mixing of the powder to homogenize the mixture. After resting for at least 24 h in a closed container in the drying oven, this powder was further used for pressing pellets and for NMR measurements. The soaking electrolyte was added in such an amount that the liquid content equalled 30 wt% of the solid content. This corresponds to a content of 23 wt% ionic liquid or soaking electrolyte, while the MOF fraction of the samples with IL or soaking electrolyte was always 77 wt%.
In order to check the influence of Li+ at the carboxylic groups of MIL-121 and the role of the Li salt (i.e. LiTFSI), on the ionic conductivity, different pellet samples were prepared for impedance spectroscopy. MIL-121/Li, MIL-121/Li + IL, MIL-121/Li + IL + LiTFSI, MIL-121 + IL + LiTFSI and MIL-121 + IL were pressed into pellets at a load of 0.3 tons and sputtered with Au electrodes that act as blocking electrodes for Li ions. The pellets had a diameter of 5 mm with a thickness of about 1 mm. Measurements were carried out in coin cells in order to keep samples free from moisture and air. A Concept 80 impedance spectrometer (Novocontrol) equipped with an Alpha-A impedance analyzer in combination with an active ZGS sample cell was used. Frequencies covered a range from 107 to 10−2 Hz; the amplitude of the applied AC voltage was 0.1 V and the temperature domain was between –90 and 110°C. Conductivity data points for the calculation of the activation energy were taken from the DC plateaus of conductivity isotherms. Isotherms were recorded every 20°C during the cooling run, which was started after the temperature reached 110°C.
Variable-temperature 7Li NMR lines were recorded with a spectrometer from Bruker (Avance III 300). A 7.0 T cryomagnet field strength results in a resonance frequency of 116.59 MHz for 7Li nucleus. The lines were recorded under static conditions, with 90° pulse lengths in the order of 2–3 μs and a recycle delay of 10 s 128 FIDs (free induction decays) were accumulated to achieve an acceptable signal-to-noise ratio. Prior to NMR measurements, the various samples were filled in Duran tubes and fire sealed to keep them free of moisture and air. A summary of the sample compositions and the abbreviations used in this report is shown in Table 1.
3 Results and Discussion
The MIL-121 MOF is formed by the coordination of aluminum metal centres with 1,2,4,5-benzenetetracarboxylic acid, also known as pyromellitic acid. Only two of the four carboxylic functional groups participate in building the MOF structure, the other two are free and situated along the linear pores characteristic to the MIL-121 structure, as shown in Figure 1. The protons of these free carboxylic units can be exchanged partially with Li+ ions. With the lithium acetate solution (see Materials and Methods), we reach a degree of ion exchange of approximately one third, the other two thirds of the carboxylic units remain protonated.
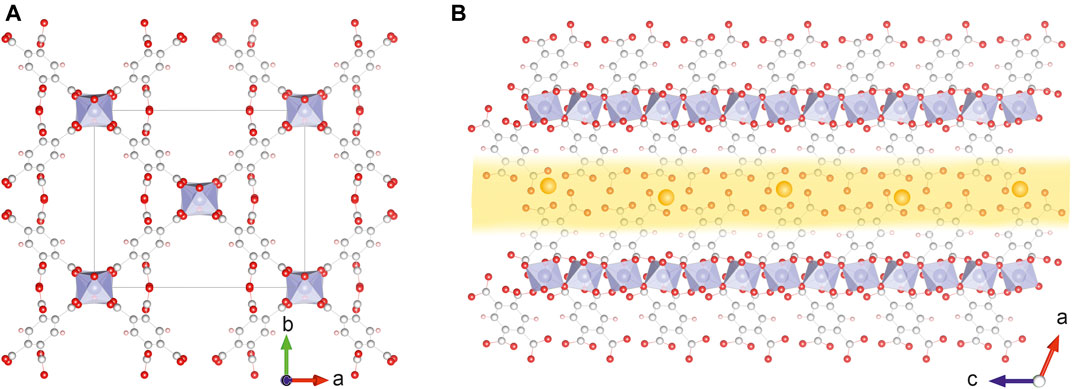
FIGURE 1. (A) The MIL-121 unit cell viewed along the crystallographic c-direction and drawn with the pores empty and fully opened. The protons of carboxylic units are omitted for the sake of clarity. (B) Section along one channel, extended for 6 unit cells in the c-direction, illustrating the infinite aluminium octahedra chains, the approximate lumen of the linear pore (yellow shade) and the free carboxylic units lining the pores where the ion exchange with Li+ (large spheres) occurs. The protons of the carboxylic groups are omitted.
Conductivity isotherms of the lithiated MIL-121 sample, thoroughly dried and without any ionic liquid, are presented in Figure 2A). MIL-121/Li is a very poor conductor, it behaves like an insulator up to 90°C. Only at 100°C and above there are indications of very poor ion transport. Yet, at a conductivity of 10−12 S cm−1 measured at the very faint DC plateau seen at 110°C, the material can hardly be classified as an ionic conductor. Very likely, the Li+ in the structure is too strongly bonded to the carboxylic group, resulting in negligible mobility. One option would be the addition of a solvent or a soaking electrolyte that may enter the pores and solvate the Li+ ions. For this purpose, we investigated the addition of EMIM-TFSI ionic liquid-based soaking electrolyte.
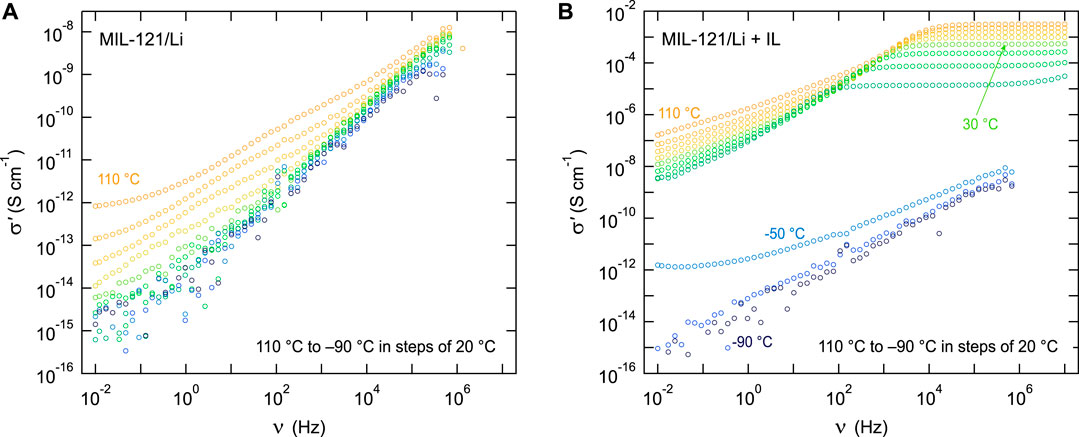
FIGURE 2. (A) Conductivity isotherms of the lithiated MIL-121/Li sample. Approximately one third of the protons of the carboxylic groups are exchanged with Li+ ions. Without any ionic liquid, the dry MIL-121/Li behaves like an insulator up to 110°C, where it shows an extremely poor conductivity at low frequencies. (B) Conductivity isotherms of the lithiated MIL-121/Li + IL showing a conductivity that is eight orders of magnitude higher than that of MIL-121/Li. This sample contains 23 wt% EMIM-TFSI ionic liquid and 77 wt% MIL-121/Li.
As shown in Figure 2B), adding 23 wt% EMIM-TFSI ionic liquid to the lithium exchanged MOF increases the conductivity to 0.5 mS cm−1 at 30°C. Compared to MIL-121/Li, this jump in DC conductivity corresponds to an increase by more than eight orders of magnitude. While the MIL-121/Li + IL contains 23% by mass EMIM-TFSI ionic liquid, the sample keeps a true solid appearance, is not waxy and can be pressed into pellets.
The dry MIL-121/Li presents a faint second DC plateau in the region 104–102 Hz. This may be attributed to some local electrical relaxation processes, for instance localized ion dynamics, such as ion motion within the boundaries of a confining potential energy well, or cavity, of the structure. This weak contribution also shows up on the electric modulus plot, see Supplementary Figure S1 D). The absence of a corresponding perturbation on the imaginary part of the impedance (Z”) confirms the localized nature of the electrical relaxation process.
Nyquist plots of MIL-121/Li + IL are shown in Figures 3A,B) which correspond to temperatures of –30°C and 30°C, respectively. The semicircles can be fitted with a simple equivalent circuit consisting of a constant phase element in parallel to a resistor. We may remind that the impedance of a constant phase element is given by Z = 1/[Cp(jω)a], where j is the imaginary unit, ω = 2πν is the angular frequency, Cp is the capacitance of the constant phase element and a is the exponent of the constant phase element. For a = 1, the constant phase element reverts to an ideal capacitor. For a < 1, the behaviour deviates from that of a capacitor and the “capacitance” of the constant phase element is correctly expressed in F/s(1−a). However, in practice, for a ≈ 1, the behaviour of the constant phase element would be very close to that of a capacitor and we may express the capacitance in F (Farads).
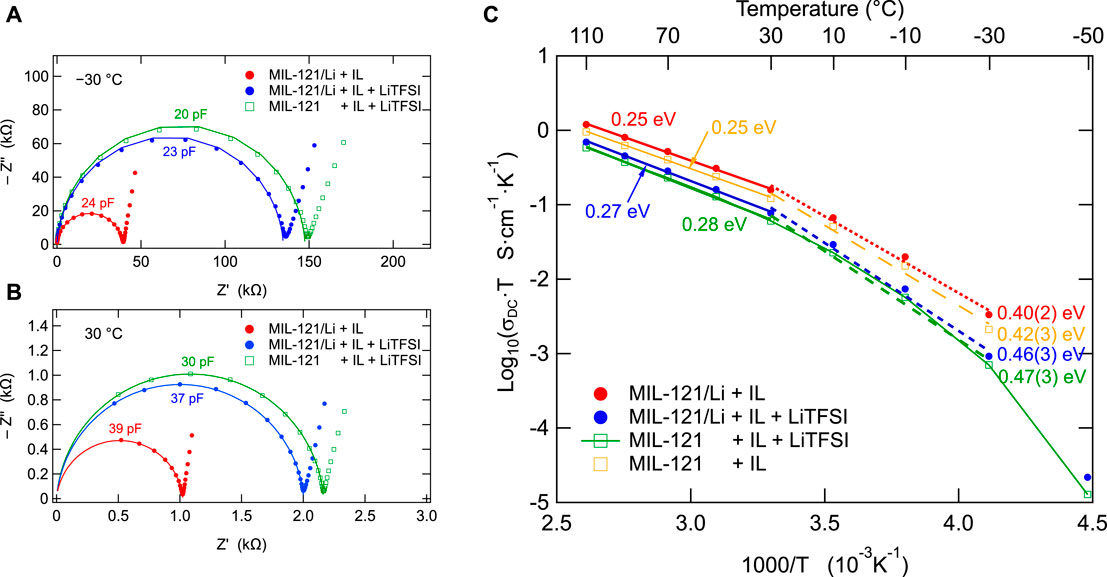
FIGURE 3. (A), (B) Nyquist plots of the high conductivity MIL-121 hybrid electrolytes at –30°C and at 30°C. (A) The solid lines are obtained by fitting the impedance data points on the shown semicircles. (B) The solid lines are obtained by fitting the data points of the partially seen semicircles followed by simulation over an extended frequency domain up to 200 MHz. (C) Arrhenius plots and the activation energies corresponding to ion conduction in the investigated materials. Straight lines are linear data fits. The thin green line, corresponding to MIL-121 + IL + LiTFSI is a guide to the eye, drawn to illustrate the non-linear Arrhenius behaviour. At temperature above 30°C the Arrhenius plot is linear. Below 30°C we see a departure from linearity indicating the additional occurrence of electrical relaxation phenomena other than simple elementary ion jumps.
Indeed, the Nyquist plots shown in Figures 3A,B) show almost perfectly shaped semicircles, indicating an almost ideal Debye behaviour. The values of the constant phase element exponent, a, are close to 1 (see table 2), which indicates a behaviour very close to that of an ideal capacitor. In addition, capacitances ranging from 20–40 pF reveal that the electrical relaxation phenomena involved are bulk processes, in accordance to the model of Irvine and West. (Irvine et al., 1990).
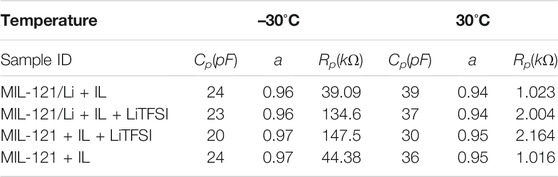
TABLE 2. Parameters obtained by fitting the impedance semicircles in Figures 3B,C) with a constant phase element (CPE) in parallel to a resistor Rp. Cp is the capacitance and a is the exponent of the CPE.
The Arrhenius plots of all samples containing ionic liquids are shown in Figure 3C). Above 30°C, σDCT follows an Arrhenius behaviour characterized by activation energies ranging from 0.25 to 0.28 eV for all the samples that contain EMIM-TFSI ionic liquid. An interesting feature is the appearance of a kink at 30°C. At lower temperatures the situation changes significantly. Not only that the activation energies sensibly increase, but also the electric relaxation mechanism very likely changes. Indeed, we see a small, but clear departure from linear Arrhenius behaviour, indicating that, at lower temperatures, electric relaxation may occur also by other phenomena than ion transport in the material. Such a behaviour is known for some polymer electrolytes, where the Vogel-Fulcher-Tammann (VFT) equation is commonly used instead of the Arrhenius equation. (Ratner and Shriver, 1988). Here, this non-linear behaviour may also be an expression of the increasing viscosity with the decreasing temperature, (Tammann and Hesse, 1926), although this aspect requires further clarifying investigations.
Surprisingly, there is no significant variation of conductivity when more Li+ ions are included in the system by dissolving LiTFSI in EMIM-TFSI. Moreover, modifying the MOF, i.e. introducing Li+ ions into the structure via an ion exchange reaction, does not lead to a decisive enhancement in conductivity. If we compare the MIL-121/Li + IL with MIL-121/Li + IL + LiTFSI and with MIL-121 + IL + LiTFSI we see a change by a factor of two, the conductivity of MIL-121/Li + IL turned out to be slightly higher. The conductivity isotherms of MIL-121 + IL + LiTFSI and MIL-121/Li + IL + LiTFSI are shown in Supplementary Figure S1A, B. Even more surprising is the absence of clear differences between a sample that contains a lithiated MOF and a sample that does not contain a lithiated MOF, while both contain the same amount of 0.4 M LiTFSI in EMIM-TFSI electrolyte.
To estimate the self-diffusivity of Li+ in the materials we carried out nuclear magnetic resonance (NMR) measurements. The 7Li NMR line-width depends on the mobility of Li+ in the material and thus on temperature. A narrow NMR line is a clear indication of rapid lithium ion exchange processes. This phenomenon, called motional line narrowing, can be used to estimate the relative number of ion jumps per second, also known as the jump rate. Thus, by looking at the relative widths of the NMR lines it is possible to distinguish between slow and fast ion conductors. (Wilkening et al., 2008; Kuhn et al., 2011).
7Li NMR line measurements are shown in Figure 4 for all the samples containing Li+. Please note that, in these samples, there are two sources of Li+. The first is the ion exchange process, see MIL-121/Li, Figure 4A). The second is the Li+ -containing ionic liquid that was added to both the ion-exchanged sample, see MIL-121/Li + IL + LiTFSI on Figure 4B), and also to the Li-free MOF structure, that was not previously ion-exchanged, see MIL-121 + IL + LiTFSI Figure 4C).
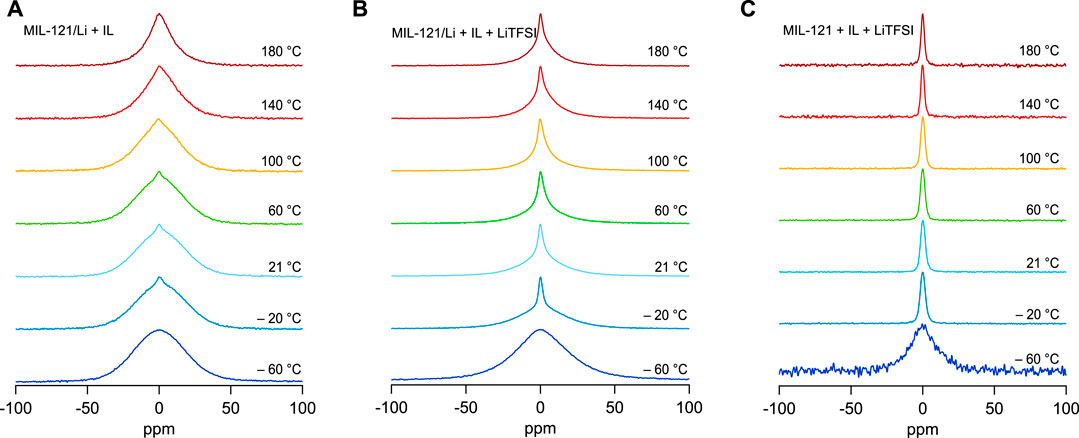
FIGURE 4. (A) 7Li NMR lines acquired in a magnetic field strength B0 = 7 T for which the 7Li NMR central resonance frequency is 116.59 MHz. (A) The 7Li NMR line width of MIL-121/Li + IL shows little motional narrowing with increasing temperature. However, a small narrower line that can be assigned to faster jumping Li+ ions is visible on top of the broad 7Li signal. The broad line can probably be assigned to the slow jumping of Li+ contained in the functionalized MOF. (B) The 7Li NMR lines at various temperature of a sample that contains 77 wt% MIL-121/Li and 23 wt% solution of 0.4 M LiTFSI in EMIM-TFSI. (C) The 7Li NMR lines at various temperature of a sample that contains 77 wt% MIL-121 (pure activated MOF) and 23 wt% solution of 0.4 M LiTFSI in EMIM-TFSI.
We see in Figure 4C) that, for MIL-121 + IL + LiTFSI, the 7Li NMR line is fully narrowed already at − 20°C, with negligible narrowing above this temperature. At − 60°C a broad line is recorded because of the solidification of the 0.4 M LiTFSI in EMIM-TFSI solution. This sample has no lithium from the ion-exchange procedure, all lithium originates from the 23 wt% Li+ containing ionic liquid soaking electrolyte that was added. This indicates that all Li+ species are already mobile above −20°C.
For the sample where all the Li+ originates from the ion-exchange procedure, i.e. MIL-121/Li + IL, see Figure 4A), we see a much different behaviour. 7Li NMR lines are broad over the entire domain and a very small narrowed line appears on top of the broad contribution at temperatures above −20°C. This indicates the existence of two lithium spin reservoirs in the material. One lithium spin reservoir shows low Li+ jump rates, whereas the other is very likely a faster ion conductor characterized by much higher Li+ jump rates. However, the fraction of fast Li+ ions appears small in comparison to the slow, poorly mobile Li+ species.
Thus, most of Li+ ions from the MIL-121/Li structure do not change their ion dynamics properties by the addition of EMIM-TFSI ionic liquid. Nevertheless, for few of the Li+ there is a significant increase in jump rates when pure EMIM-TFSI ionic liquid is added. This is a plausible indication that only Li+ from the surface, or near-surface, regions of the MIL-121/Li particles interact significantly with the ionic liquid.
For the sample MIL-121/Li + IL + LiTFSI, where lithium originates from both the ion-exchange procedure and from the addition of 0.4 M LiTFSI in EMIM-TFSI soaking electrolyte, we see an intermediary situation. On top of a broad 7Li NMR line we have a significantly narrower line, corresponding to a higher fraction of fast Li+ species, see Figure 4B). Of course, as the Li+ content in the ionic liquid is high and comparable to the Li+ content in the MOF structure, the area fraction under the narrow line is large.
Therefore, the high conductivity of the samples can be assigned mostly to the ionic liquid added to the MOF. The Li+ species originating from the ion exchange procedure do not appear to contribute significantly to the total conductivity of the samples. In spite of MIL-121/Li containing a significant quantity of Li+ , these ions are not mobile and they cannot be made mobile by simply adding EMIM-TFSI ionic liquid. It would appear then, that the high conductivity of the MIL-121/Li + IL sample does not stem from the high Li+ ion mobility in the sample, but from the inherent high conductivity of the EMIM-TFSI ionic liquid itself, see the conductivity of MIL-121 + IL shown in Supplementary Figure S1C).
The bulk conduction process that appears in impedance measurements of all samples (see Figures 3A,B) and table 2) does not correspond to conduction in the MOF, but to conduction on the surface of the MOF or in the ionic liquid only. While further investigations are necessary, we note that the occurrence of a capacitance contribution that closely approaches the behaviour of a capacitor would be unusual for a highly porous sample characterized by nano-domains and uniformly filled with mobile adsorbed molecules or mobile ions. Thus, it may happen that the ionic liquid does not enter the linear pores of the MIL-121 structure.
Moreover, the decrease in conductivity when LiTFSI is added to the MIL-121/Li + IL, as seen in Figures 3A,B), is typical to the behaviour of a salt dissolved in an ionic liquid. In fact, the conductivity of the pure ionic liquid (EMIM-TFSI) is known to be higher than the conductivity of a LiTFSI solution in EMIM-TFSI. The LiTFSI solution in EMIM-TFSI has a higher viscosity than the pure ionic liquid and hence the solution has a lower conductivity than the pure ionic liquid. (Wu et al., 2013). Thus, we see a typical ionic liquid solution behaviour, which does not appear to be visibly altered by the presence of lithiated MIL-121 particles. This behaviour could be very well explained if the ionic liquid species do not enter the pores of the MOF.
The MIL-121 samples consist of well grown and crystallized particles that are in the order of 10 μm. If the ionic liquid does not go significantly inside the pores, then it must reside on the outer surface area of the MOF and in the voids between MOF particles. Thus, the bulk electrical relaxation process seen for all the samples containing an ionic liquid, very likely corresponds almost exclusively to the bulk conductivity of the ionic liquid phase itself.
4 Conclusion
MIL-121 metal organic frameworks were prepared and their ion conduction properties were investigated. Following an ion-exchanged procedure, one third of the protons on the free carboxylic groups in the MOF were exchanged with Li+ ions. The conductivity of the dry ion-exchanged sample is very low. We found that by the addition of an ionic liquid or an ionic liquid-based Li+ electrolyte, the DC conductivity (σDC) of the MOF materials increase by eight orders of magnitude. There are indications that, the ionic liquid does not increase the mobility of a significant fraction of Li+ ions from the ion-exchanged MOF structure. Thus, the origin of conductivity in MIL-121 functionalized with Li+ and soaked with ionic liquids is in fact, to a very large extent, due to the ionic liquid located outside of the solid MOF particles. The majority of Li+ ions in the MOF structure are rather slow and do not significantly participate in long range ion transport. Also, we found indications that by soaking the MIL-121 MOF in EMIM-TFSI ionic liquid, the ionic liquid does not enter the pores of the material to a significant extent. This observation requires, however, further work for a definite confirmation.
Data Availability Statement
The raw data supporting the conclusions of this article will be made available by the authors, without undue reservation.
Author Contributions
RZ synthesized the MOFs and prepared the investigated materials, conducted the impedance and NMR measurements and plotted the data. IH conceived and supervised this research. IH and RZ interpreted the data and wrote the manuscript together. IH acquired the funding for this investigation.
Funding
This research received funding from Land Steiermark through Zukunftsfonds Steiermark (project Hybrid-Solarzellenbatterie, grant no. 1341) and from the FFG (K-project Safe Battery, grant no. 856234).
Conflict of Interest
The authors declare that the research was conducted in the absence of any commercial or financial relationships that could be construed as a potential conflict of interest.
Publisher’s Note
All claims expressed in this article are solely those of the authors and do not necessarily represent those of their affiliated organizations, or those of the publisher, the editors and the reviewers. Any product that may be evaluated in this article, or claim that may be made by its manufacturer, is not guaranteed or endorsed by the publisher.
Acknowledgments
We thank Prof. H. Martin R. Wilkening for many useful discussions and for allowing us to use his impedance equipment and NMR spectrometer in Graz. We thank Prof. W. Gössler and S. Meschnark for the elemental analysis (Karl Franzens University Graz). The Deutsche Forschungsgemeinschaft (DFG) (FOR 1227 MoLiFe, project LIDINAM, grant HA6966/1-2) and Austrian Research Promotion Agency (FFG) (project SolaBat, grant 853627) are kindly acknowledged.
Supplementary Material
The Supplementary Material for this article can be found online at: https://www.frontiersin.org/articles/10.3389/fenrg.2021.714698/full#supplementary-material
References
Ameloot, R., Aubrey, M., Wiers, B. M., Gómora-Figueroa, A. P., Patel, S. N., Balsara, N. P., et al. (2013). Ionic Conductivity in the Metal-Organic Framework UiO-66 by Dehydration and Insertion of Lithiumtert-Butoxide. Chem. Eur. J. 19, 5533–5536. doi:10.1002/chem.201300326
Chen, R., Nolan, A. M., Lu, J., Wang, J., Yu, X., Mo, Y., et al. (2020). The Thermal Stability of Lithium Solid Electrolytes with Metallic Lithium. Joule 4, 812–821. doi:10.1016/j.joule.2020.03.012
Chen, S., Mukherjee, S., Lucier, B. E. G., Guo, Y., Wong, Y. T. A., Terskikh, V. V., et al. (2019). Cleaving Carboxyls: Understanding Thermally Triggered Hierarchical Pores in the Metal-Organic Framework MIL-121. J. Am. Chem. Soc. 141, 14257–14271. doi:10.1021/jacs.9b06194
Chung, H., and Kang, B. (2017). Mechanical and Thermal Failure Induced by Contact between a Li1.5Al0.5Ge1.5(PO4)3 Solid Electrolyte and Li Metal in an All Solid-State Li Cell. Chem. Mater. 29, 8611–8619. doi:10.1021/acs.chemmater.7b02301
Irvine, J. T. S., Sinclair, D. C., and West, A. R. (1990). Electroceramics: Characterization by Impedance Spectroscopy. Adv. Mater. 2, 132–138. doi:10.1002/adma.19900020304
Kim, T., Song, W., Son, D.-Y., Ono, L. K., and Qi, Y. (2019). Lithium-ion Batteries: Outlook on Present, Future, and Hybridized Technologies. J. Mater. Chem. A. 7, 2942–2964. doi:10.1039/C8TA10513H
Kuhn, A., Narayanan, S., Spencer, L., Goward, G., Thangadurai, V., and Wilkening, M. (2011). Li Self-Diffusion in Garnet-type Li7La3Zr2O12as Probed Directly by Diffusion-inducedLi7spin-Lattice Relaxation NMR Spectroscopy. Phys. Rev. B. 83, 094302. doi:10.1103/PhysRevB.83.094302
Miner, E. M., Park, S. S., and Dincă, M. (2019). High Li+ and Mg2+ Conductivity in a Cu-Azolate Metal-Organic Framework. J. Am. Chem. Soc. 141, 4422–4427. doi:10.1021/jacs.8b13418
Park, S. S., Tulchinsky, Y., and Dincă, M. (2017). Single-Ion Li+, Na+, and Mg2+ Solid Electrolytes Supported by a Mesoporous Anionic Cu-Azolate Metal-Organic Framework. J. Am. Chem. Soc. 139, 13260–13263. doi:10.1021/jacs.7b06197
Porz, L., Swamy, T., Sheldon, B. W., Rettenwander, D., Frömling, T., Thaman, H. L., et al. (2017). Mechanism of Lithium Metal Penetration through Inorganic Solid Electrolytes. Adv. Energ. Mater. 7, 1701003. doi:10.1002/aenm.201701003
Ratner, M. A., and Shriver, D. F. (1988). Ion Transport in Solvent-free Polymers. Chem. Rev. 88, 109–124. doi:10.1021/cr00083a006
Sadakiyo, M., and Kitagawa, H. (2021). Ion-conductive Metal-Organic Frameworks. Dalton Trans. 50, 5385–5397. doi:10.1039/D0DT04384B
Tammann, G., and Hesse, W. (1926). Die Abhängigkeit der Viscosität von der Temperatur bie unterkühlten Flüssigkeiten. Z. Anorg. Allg. Chem. 156, 245–257. doi:10.1002/zaac.19261560121
Wiers, B. M., Foo, M.-L., Balsara, N. P., and Long, J. R. (2011). A Solid Lithium Electrolyte via Addition of Lithium Isopropoxide to a Metal-Organic Framework with Open Metal Sites. J. Am. Chem. Soc. 133, 14522–14525. doi:10.1021/ja205827z
Wilkening, M., Epp, V., Feldhoff, A., and Heitjans, P. (2008). Tuning the Li Diffusivity of Poor Ionic Conductors by Mechanical Treatment: High Li Conductivity of Strongly Defective LiTaO3 Nanoparticles. J. Phys. Chem. C. 112, 9291–9300. doi:10.1021/jp801537s
Wu, T. Y., Hao, L., Chen, P. R., and Liao, J. W. (2013). Ionic Conductivity and Transporting Properties in LiTFSI-Doped Bis(trifluoromethanesulfonyl)imide-Based Ionic Liquid Electrolyte. Int. J. Electrochem. Sci. 8, 2606–2624.
Zettl, R., Lunghammer, S., Gadermaier, B., Boulaoued, A., Johansson, P., Wilkening, H. M. R., et al. (2021). High Li + and Na + Conductivity in New Hybrid Solid Electrolytes Based on the Porous MIL‐121 Metal Organic Framework. Adv. Energ. Mater. 11, 2003542. doi:10.1002/aenm.202003542
Zhang, Z., Shao, Y., Lotsch, B., Hu, Y.-S., Li, H., Janek, J., et al. (2018). New Horizons for Inorganic Solid State Ion Conductors. Energy Environ. Sci. 11, 1945–1976. doi:10.1039/C8EE01053F
Zhao, W., Yi, J., He, P., and Zhou, H. (2019). Solid-State Electrolytes for Lithium-Ion Batteries: Fundamentals, Challenges and Perspectives. Electrochem. Energ. Rev. 2, 574–605. doi:10.1007/s41918-019-00048-0
Zheng, F., Kotobuki, M., Song, S., Lai, M. O., and Lu, L. (2018). Review on Solid Electrolytes for All-Solid-State Lithium-Ion Batteries. J. Power Sourc. 389, 198–213. doi:10.1016/j.jpowsour.2018.04.022
Zheng, J., Kim, M. S., Tu, Z., Choudhury, S., Tang, T., and Archer, L. A. (2020a). Regulating Electrodeposition Morphology of Lithium: towards Commercially Relevant Secondary Li Metal Batteries. Chem. Soc. Rev. 49, 2701–2750. doi:10.1039/c9cs00883g
Keywords: ion conduction, metal organic framework (MOF), solid electrolytes, solid-state batteries, conductivity spectroscopy, ionic Liquids
Citation: Zettl R and Hanzu I (2021) The Origins of Ion Conductivity in MOF-Ionic Liquids Hybrid Solid Electrolytes. Front. Energy Res. 9:714698. doi: 10.3389/fenrg.2021.714698
Received: 25 May 2021; Accepted: 22 September 2021;
Published: 08 October 2021.
Edited by:
Shripad T Revankar, Purdue University, United StatesReviewed by:
Sheng S. Zhang, United States Army Research Laboratory, United StatesUwe Schröder, Technische Universitat Braunschweig, Germany
Copyright © 2021 Zettl and Hanzu. This is an open-access article distributed under the terms of the Creative Commons Attribution License (CC BY). The use, distribution or reproduction in other forums is permitted, provided the original author(s) and the copyright owner(s) are credited and that the original publication in this journal is cited, in accordance with accepted academic practice. No use, distribution or reproduction is permitted which does not comply with these terms.
*Correspondence: Ilie Hanzu, aGFuenVAdHVncmF6LmF0