- 1Department of Agricultural and Biological Engineering, University of Illinois at Urbana-Champaign, Urbana, IL, United States
- 2Center for Advanced Bioenergy and Bioproduct Innovations (CABBI), Department of Energy, Urbana, IL, United States
- 3Carl R. Woese Institute for Genomic Biology, University of Illinois at Urbana-Champaign, Urbana, IL, United States
Transgenic bioenergy crops have shown the potential to produce vegetative oil by accumulating energy-rich triacylglyceride molecules that can be converted into biofuels (biodiesel and biojet). These transgenic crops cater to improved biofuel yield by providing lipids along with cellulosic sugars. Efficient bioprocessing technologies are needed to utilize these transgenic plants to their maximum potential. To this end, this study investigates a low- and high-severity chemical-free hydrothermal pretreatment of transgenic oilcane 1566 bagasse with in situ lipids to maximize the recovery of lipids for biodiesel and fermentable sugars for ethanol with minimal inhibitor generation. Hydrothermal pretreatment at 170°C recovered ∼25% of total lipids in the pretreatment liquor, leaving the remainder in bagasse residue for hexane recovery post fermentation . The recovery of lipids in pretreatment liquor remained constant beyond 170°C. Along with lipids, ∼35% w/w and ∼50% w/w fermentable sugars were recovered post saccharification from bagasse pretreated at 170°C and 210°C for 20 min, respectively. Hydrothermal pretreatment at 170°C for 20 min provided the optimum conditions for maximum recovery of lipids and cellulosic sugars that resulted in enhanced biofuel yield per unit biomass. High severity pretreatment increased the generation of inhibitors beyond the tolerance of fermentation microorganisms. In addition, the application of time-domain proton NMR spectroscopy was extended to bioprocessing. NMR technology facilitated the analysis of total lipids, the composition of fatty acids, and the characterization of free and bound lipids in untreated and pretreated oilcane 1566 bagasse subsequent to each step of biomass to biofuel conversion.
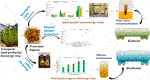
Introduction
Biofuels are a drop-in, environmentally nontoxic, biodegradable, and less contaminating alternative to conventional fuels with comparable energy efficiency, with strong potential for lowering CO2 emissions. Cellulosic biomass has immense potential as a renewable feedstock for global energy needs. Bioenergy from traditional biomass such as cereal grains, forestry, and other energy crops constitutes approximately 7% of total global energy consumption (REN21, 2020). Oils from plants are particularly valued since they can be easily converted to biodiesel and bio-jet, serving markets that are unlikely to be electrified. To this end, bioenergy crops such as sugarcane, energycane, sorghum, and miscanthus are being metabolically engineered to shift the carbon flux from sugar to lipid synthesis and accumulation in their vegetative tissues (Zale et al., 2016; Vanhercke et al., 2019; Parajuli et al., 2020).
The accumulation of energy-rich triacylglyceride (TAG) molecules enhances the energy density of these transgenic bioenergy crops as compared to their wild-type varieties. These metabolically engineered transgenic bioenergy crops can be used for the production of both fermentable sugars and lipids/oil. Moreover, the very high productivity of these crops would result in substantially more oil per unit area than conventionally used oilseed crops (Parajuli et al., 2020). For example, sugarcane produces an annual yield of dry biomass of at least 10–20 times more per hectare than soybean; thus, accumulation of ∼20% lipids in the vegetative tissues would produce 10–20 times more oil for biodiesel than soybean per unit area (Huang et al., 2016a; 2016b). However, the quality of biodiesel is influenced by the composition of the total lipids of transgenic crops. Genetic engineering to modify the metabolic pathways of transgenic bioenergy crops provides a tool to develop desired feedstock to improve biodiesel quality by having a higher content of short, unbranched, and saturated fatty acids (Knothe, 2008, 2009).
Mechanical pressing and solvent extraction are the two most commonly used procedures for commercial oil extraction from oilseeds for biodiesel production (Atabani et al., 2013; Bhuiya et al., 2016). Although it is anticipated that refinement of bioengineering would eventually raise total oil content in transgenic bioenergy crops to 20%, initial steps have only raised total TAG content to 8 and 4.3% dry weight in the leaf and stem, respectively, along with an increase in total fatty acid content to 13% dry weight in the leaf (Parajuli et al., 2020). Nevertheless, this provides material on which challenges to extraction technologies may be evaluated. Since the total lipid content in transgenic bioenergy crops is lower than that in oilseeds and a considerable percentage of lipids are present in a complex bound form (Zale et al., 2016; Parajuli et al., 2020; Maitra et al., 2021), the conventional methods for oil recovery from oilseeds are not sufficient for effective lipid recovery from transgenic cellulosic biomass. Chemical-free low-severity methods for the extraction are necessary to prevent the decomposition of lipids during pretreatment, followed by saccharification of the residual cellulosic biomass to produce fermentable sugars. Jia et al. (2020) reported an improvement in lipid recovery upon hydrothermal pretreatment of oil-bearing corn germ meal at 180°C for 15 min (Jia et al., 2020).
The time-domain NMR technique provides a faster alternative to the existing lipid characterization and profiling techniques (Berman et al., 2013; Robinson and Cistola, 2014; Nascimento et al., 2017; Nikolskaya and Hiltunen, 2018). Rapid analytical methods for the initial screening of samples are critical in optimizing the extraction technology. Previously, we showed the application of TD-NMR as an effective high-throughput phenotyping method to quantify and characterize in situ lipids in transgenic lignocellulosic bagasse (Maitra et al., 2021). TD-NMR facilitates direct analysis of cellulosic biomass for lipid analysis without sample preparation or lipid extraction. Thus, TD-1H NMR provides a convenient and rapid alternative to the tedious wet chemistry technique of fatty acid analysis. The application of TD-NMR for qualitative and quantitative characterization of in situ vegetative lipids in cellulosic biomass after each bioprocessing step such as pretreatment, saccharification, simultaneous saccharification, and fermentation (SSF) has not been reported.
The present work investigates low- and high-severity hydrothermal pretreatment for maximizing lipid and sugar recovery with negligible inhibitor generation from the transgenic lipid-producing sugarcane (oilcane 1566) bagasse and extends the application of TD-NMR spectroscopy for the estimation of the total lipid and fatty acid composition in the cellulosic biomass before and after each of the following steps: pretreatment, enzyme hydrolysis, and SSF.
Materials and Methods
Transgenic Bioenergy Crop
The transgenic oilcane event 1566 was grown in a greenhouse at the University of Illinois at Urbana-Champaign under controlled environmental conditions. This was a 16-h day in which sunlight was supplemented to ensure a minimum photon flux of 600 μmol m−2 s−1 light from high-pressure sodium lamps. The temperature was controlled between 28° and 31°C. Whole shoots of wild-type and transgenic plants were harvested at the stage that they would be harvested in the field for processing, that is, before flowering. The leaves were separated from the stem, and juice was extracted using a juicer (Juicematic SC-3 commercial sugarcane juicer). Each stem was passed through the juicer three times to extract maximum juice. The bagasse was dried to constant weight at 50°C. The dried bagasse was cut into smaller pieces of 1–2 inches with pruning shears, followed by shredding in a hammer mill (W-8-H, Schutte-Buffalo Hammermill, Buffalo, NY), with a sieve size of 2 mm. Bagasse was stored at −20°C until it could be used for further processing.
Hydrothermal Pretreatment
The hydrothermal pretreatment at 170 and 210°C for 10 and 20 min was performed in a sand bath (IFB-51 Industrial Fluidized Bath, Techne Inc., Burlington, NJ) attached to an air compressor for even heat distribution. Pretreatment was performed with a 20% w/w biomass solid loading. The transgenic oilcane 1566 bagasse samples were loaded into capped pipe reactors (316 stainless steel reactors: 10.478 cm length × 1.905 cm outer diameter × 0.165 cm wall thickness tubing, SS-T12-S-065–20, Swagelok, Chicago Fluid System Technologies, Chicago, IL; 316 stainless steel caps: SS-1210-C, Swagelok, Chicago Fluid System Technologies, Chicago, IL). A thermocouple [Penetration/Immersion Thermocouple Probe Mini Conn (−418–1652°F), Mc Master-Carr, Robbinsville, NJ] inserted into the reference pipe reactor filled with deionized water was used to monitor the in situ temperature. The thermocouple was connected to a data logger (HH306/306A, Datalogger Thermometer, Omega, Stamford, CT). The pretreatment reactions were quenched immediately after 10 and 20 min of pretreatment by submerging the reactors into cold water. The resulting, pretreatment liquor was analyzed for solubilized sugars and inhibitors using HPLC. The pretreated bagasse residue was kept at 4°C for lipid analysis and saccharification.
Saccharification
Enzymatic saccharification was performed on both untreated and pretreated transgenic oilcane 1566 bagasse using standard protocol NREL/TP-5100-63351 (Resch et al., 2015). Enzyme hydrolysis was carried out with 10% (w/w) biomass solid loading for 72 h at 50°C in an incubator shaker at 180 rpm. The working concentrations of the enzyme hydrolysis reaction mixture contained citrate buffer (0.05 M) and sodium azide (0.005%) to inhibit the growth of microorganisms and 16.9 mg protein/g of dry biomass of cellulase and hemicellulose mixture [59.64 FPU/g dry biomass enzyme loading (NS 22257, Novozymes North America, Inc., Franklinton, NC, United States)].
Simultaneous Saccharification and Fermentation (SSF)
Simultaneous saccharification and fermentation were performed on untreated and two differently pretreated transgenic 1566 bagasse [170°C for 20 min and 210°C for 20 min]. The working concentrations of the SSF reaction mixture contained citrate buffer (0.05 M), 16.9 mg protein/g of dry biomass of cellulase and hemicellulase mixture (NS 22257, Novozymes North America, Inc., Franklinton, NC, United States )], and C6/C5 fermenting recombinant Saccharomyces cerevisiae (kindly provided by DSM). The yeast was grown on a YPX medium (1% yeast extract, 2% peptone, and 2% xylose) for 48 h at 32°C. Yeast cells were centrifuged and inoculated in the SSF medium to obtain a starting cell O.D600 of 0.5. SSF was carried out for 96 h in a shaking incubator (180 rpm) at 32°C with 10% solid loading and sampled after every 24 h. The samples were filtered using 0.2-μm syringe filters and analyzed for sugars and ethanol using HPLC. The bagasse residue obtained post SSF was evaluated for lipid content and composition using TD-NMR.
Sugars and Inhibitor Analysis
The pretreatment liquors and hydrolyzates were centrifuged to separate the solid particles. The supernatants were filtered through 0.2-μm PTFE filters for HPLC analysis. The concentrations of sugars and inhibitors were estimated using an HPLC system (Waters alliance e2695 Separation module, Waters Corporation, MA, United States ) equipped with a refractive index detector and a Bio-Rad Aminex HPX- 87H column (Bio-Rad, Hercules, CA, United States ).
Time-Domain 1H-NMR Spectroscopy
Time-domain NMR spectroscopy was used for quantification of total lipids and major fatty acids, that is, palmitic (C16:0), oleic (C18:1), and linoleic (c18:2) acid and characterization of bound and free lipids in the untreated and pretreated transgenic oilcane 1566 bagasse.
Quantification of Total Lipid Using Benchtop Time-Domain- 1H-NMR Spectroscopy
The total in situ lipids in untreated and pretreated transgenic oilcane 1566 bagasse were quantified and analyzed for bound and free fractions and major fatty acids (palmitic acid, oleic acid, and linoleic acid) using TD-NMR spectroscopy (Maitra et al., 2021). A low-field benchtop time-domain proton NMR spectroscope (Minispec mq20, Bruker, Massachusetts, United States) was used. The NMR system was equipped with an 18-mm thermostat 1H-probe operating at 0.47 T/20 MHz and 40°C. For lipid analysis, the bagasse samples were dried (≤2% moisture content) to alleviate the interference of proton signals from water molecules. The time-domain 1H NMR was calibrated with lipids extracted from transgenic bioenergy crops for analyzing in situ lipids in transgenic lignocellulosic biomass as reported in previous work (Maitra et al., 2021).
Characterization of Bound and Free Oil Fraction Using TD-NMR
T1T2 relaxation/intensity curves were analyzed for characterization of total lipids into bound and free lipid fractions as reported in the previous work (Maitra et al., 2021). The Carr–Purcell–Meiboom–Gill (CPMG) application was used for acquiring the full exponential decay curves for transverse (T2) relaxation times. The longitudinal (T1) relaxation time was obtained by the inversion recovery method. A CPMG pulse sequence was applied for the measurement of spin–spin relaxation. The total number of acquired echoes was 800. 90°–180° pulse separation (tau) was 2. The T1 inversion recovery relaxation time had a first and final pulse separation of 2 and 800 ms, respectively. A sampling window of 0.1 ms was used. The recycle sampling delay for the T1 inversion recovery experiment was 2 s. A total of 10 data points were used to fit the curve for each sample. Both T1 and T2 relaxation spectra were fitted to a bi-exponential equation of second order as reported previously (Maitra et al., 2021). The CONTIN algorithm software obtained from Bruker provided the continuous distribution of T1 and T2 values.
Analyzing Fatty Acid Composition Using TD-NMR
TD-1H NMR was calibrated to quantify palmitic acid (16:0), oleic acid (18:1), and linoleic acid (18:2) in transgenic bagasse samples. The minispec Plus, containing Bruker’s OPUS software, was used for chemometric processing and storage of TD-NMR data. Chemometric processing included discerning differences between samples, identifying outliers, and obtaining regression models to correlate NMR values with the existing reference data in the software. Separate calibration curves were prepared for each fatty acid. Individual pure fatty acids (≥99% purity) were purchased from Sigma-Aldrich. The fatty acids were stored at −20°C. Palmitic acid (16:0), oleic acid (18:1), and linoleic acid (18:2) used in the study did not show susceptibility to oxidation at the experimental temperature of 40°C on repeated usage.
For non-invasive analysis of fatty acid composition in bagasse samples, pure free fatty acid standards were mixed with the “biomass matrix” as a background for calibration. Since bagasse itself is a complex matrix consisting primarily of lignin, cellulose, and hemicellulose, mixed with a variety of pigments, polyquinones and their oxidation products, membrane-proteins, and phospholipids, it is critical to abate the background signal of proton nuclei because of lipid molecules. The lipid biomolecules were removed from the bagasse by hexane, isopropanol, and ethanol extraction in a Soxhlet extractor. The bagasse obtained after extraction served as a “biomass matrix” for calibration purposes. The lipid-extracted bagasse was mixed with different amounts of pure fatty acid to calibrate the TD-NMR. Palmitic acid (16:0) was heated in a water bath at 65°C to ensure that it was in the oil form and above the crystalline-liquid phase transition before mixing it into the “biomass matrix” for calibration. A combination of FID (free induction decay) and CPMG applications was used to analyze the time decay signal of each fatty acid based on the nanofluidity of hydrocarbon chain packing (Robinson and Cistola, 2014). The decay spectra were evaluated by taking 16 scans with no dummy scans and a receiver gain of 40. Each fatty acid (palmitic acid, oleic acid, and linoleic acid) is expressed as the percentage of the total lipid present per gram of dry biomass.
Organic Solvent Lipid Extraction
Lipids/oils were extracted from the untreated and pretreated bagasse using the organic solvent method as reported by Huang et al. (2017). The organic solvent–extracted samples were analyzed for lipid classes and metabolites using GC/MS and LC/MS/MS. GC/MS and LC/MS/MS were performed at the Metabolomics Lab, Roy J. Carver Biotechnology Center, University of Illinois at Urbana-Champaign, IL, United States. A benchtop Agilent GC/MS (7890A GC with 5975C MS) with commercial Wiley and NIST libraries and a mass range of m/z 2 ∼ m/z 800 designed for small metabolite analysis equipped with EI and CI sources were used for targeted metabolite profiling analysis. For LC/MS/MS analysis, a benchtop Sciex LC/MS-5500 QTrap Mass Spectrometer (hybrid triple Quadrupole-linear accelerator trap mass spectrometer) with Turbo V™ Source including ESI and APCI connected to an Agilent 1200 HPLC was used. The scan modes include full scan and selected ion scan for both Q1 and Q3, Product Ion Scan, Precursor Ion Scan, Neutral Loss Scan, Multiple Reaction Monitoring (MRM), Enhanced MS Scan, Enhanced Product Ion Scan, Enhanced Resolution Scan, and MS3 scan with a mass range of m/z 5 ∼ m/z 1,250.
Results
Lipid Analysis Using TD-NMR Spectroscopy
The recovery of lipids from transgenic bagasse needs bioprocessing that prevents them from decomposition. To maximize lipid recovery, the transgenic oilcane 1566 bagasse was pretreated with a chemical-free hydrothermal pretreatment at low and high severity. The severity of the pretreatment (
where, t, T, and
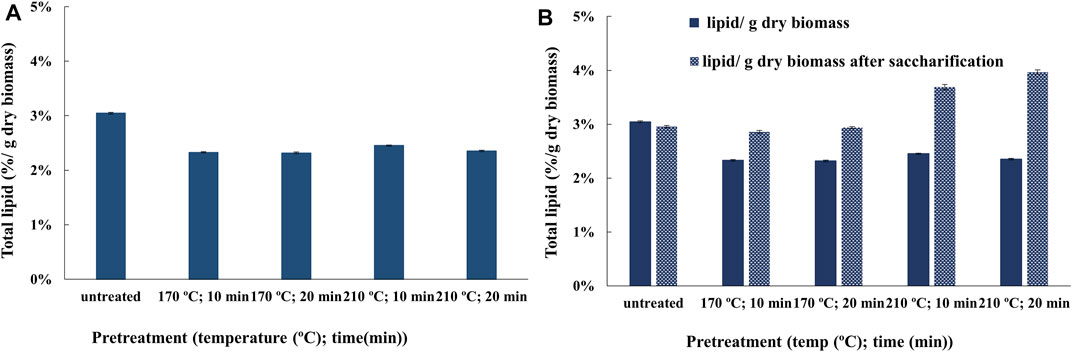
FIGURE 1. Estimation of total in situ lipids in (A) the untreated and pretreated transgenic oilcane 1566 bagasse residues and (B) untreated and pretreated bagasse residues after saccharification using TD-NMR. Data present the mean of triplicates with a standard deviation of 0.05%.
Oil-associated proton relaxation time distributions of untreated and pretreated transgenic oilcane 1566 bagasse were analyzed. The samples exhibited two distinct oil-associated subpopulations of proton nuclei. Shorter and longer relaxation times of proton nuclei correspond to a lesser and higher degree of freedom, which represents the bound and free form of oils/lipids in the cellulosic biomass. In addition, the NMR intensity and magnitude of relaxation times qualitatively correspond to the relative amount of bound and free lipids in the bagasse residue (Maitra et al., 2021). The magnitudes of T1 relaxation times of untreated and hydrothermally pretreated bagasse are presented in Table 1. A decrease in the magnitude of the T1 relaxation times of pretreated bagasse as compared to untreated bagasse confirmed the release of lipids from the bagasse to the pretreatment liquor. The magnitude of T2 relaxation times also showed lower oil-associated proton fluidity of bound oil in pretreated biomass residue as compared to the untreated bagasse, while no oil-associated proton fluidity was observed for free oil (Supplementary Table S1). The result was mirrored in the total lipid measurement using NMR spectroscopy (Figure 1A). The total lipid content of the bagasse residue was reduced from ∼3 to 2.3% per g of dry bagasse. The lipid analysis of transgenic oil-containing bagasse using NMR is in agreement with that in our previous study with model biomass (bagasse with externally added crude corn oil) that successfully established the application of TD-NMR spectroscopy for precise measurement and characterization of the residual lipids in the biomass fibers after hydrothermal pretreatment (Maitra et al., 2021).
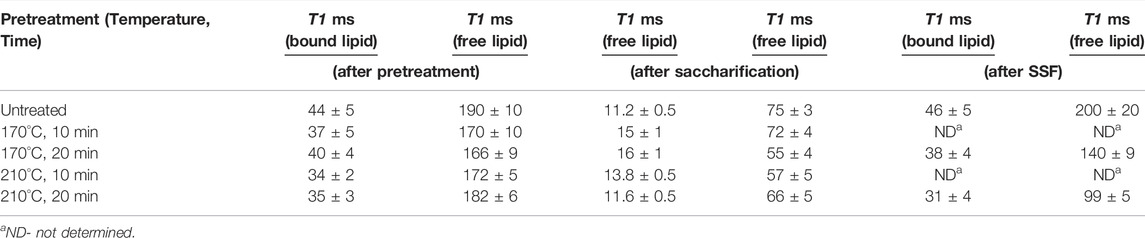
TABLE 1. Analyzing T1 relaxation/intensity curves to investigate the fraction of bound and free lipids in the untreated and pretreated bagasse residues after various hydrothermal pretreatment conditions, saccharification, and SSF.
The bagasse residues obtained post saccharification were also analyzed for total lipid content and a change in the magnitude of T1 relaxation times using TD-1H NMR to investigate the further release of lipids. After saccharification, the total lipid content of bagasse residues increased by 26 and 54% for bagasse pretreated at 170°C (∼2.3 to ∼2.9% w/w) and 210°C (∼2.4 to ∼3.9% w/w), respectively (Figure 1B). The magnitude of T1 relaxation times of bagasse residues after saccharification for free oil was found to be lower than their corresponding untreated and pretreated bagasse residues (Table 1). The magnitude of T2 relaxation times for bound oil remained low, and no oil-associated proton fluidity was observed for free oil in biomass residues after saccharification (Supplementary Table S1). The magnitude of relaxation times corresponds to the amount of oil-associated proton nuclei, which is directly correlated to the concentration of oil/lipid in the residue (Maitra et al., 2021). Therefore, this observation indicates a further recovery of 45–60% of lipids in the hydrolyzate after saccharification. However, the magnitude of T1 relaxation time for bound oil showed no significant change in the signals. This suggests that the process of enzyme hydrolysis releases sugars by disrupting the cellulosic and hemicellulosic framework of the bagasse structure, which aids in the further recovery of the free form of lipids. However, the membrane-bound lipids are not recovered.
Analysis of NMR Relaxation/Intensity Curves
The study correlates T1 relaxation/intensity curves with the recovery of lipid from bagasse residues (Figure 2). A reduction in the NMR intensity of the T1 relaxation/intensity curves of the bagasse pretreated at 170°C for 10 and 20 min as compared to the untreated bagasse suggests reduced oil-associated proton fluidity of the bagasse and, hence, is directly correlated with the release of lipids from cellulosic biomass upon pretreatment (Maitra et al., 2021). The observation is in agreement with the quantitative analysis of the lipid content of the biomass residues (Figure 1A). T2 relaxation/intensity curves mirrored the results of the T1 relaxation/intensity curves but displayed lower NMR intensity for each biomass residue (Supplementary Figure S1). On the contrary, bagasse pretreated under severe conditions, that is, 210°C for 10 and 20 min exhibited higher NMR intensity. Therefore, the samples were analyzed using GC/MS to investigate the reason for the higher NMR intensity. The GC/MS analysis of the samples showed higher amounts of phenolics and various other metabolites than the untreated bagasse (Figure 3A). The proton nucleus metabolites could possibly contribute to the total proton signals, resulting in higher NMR intensity.
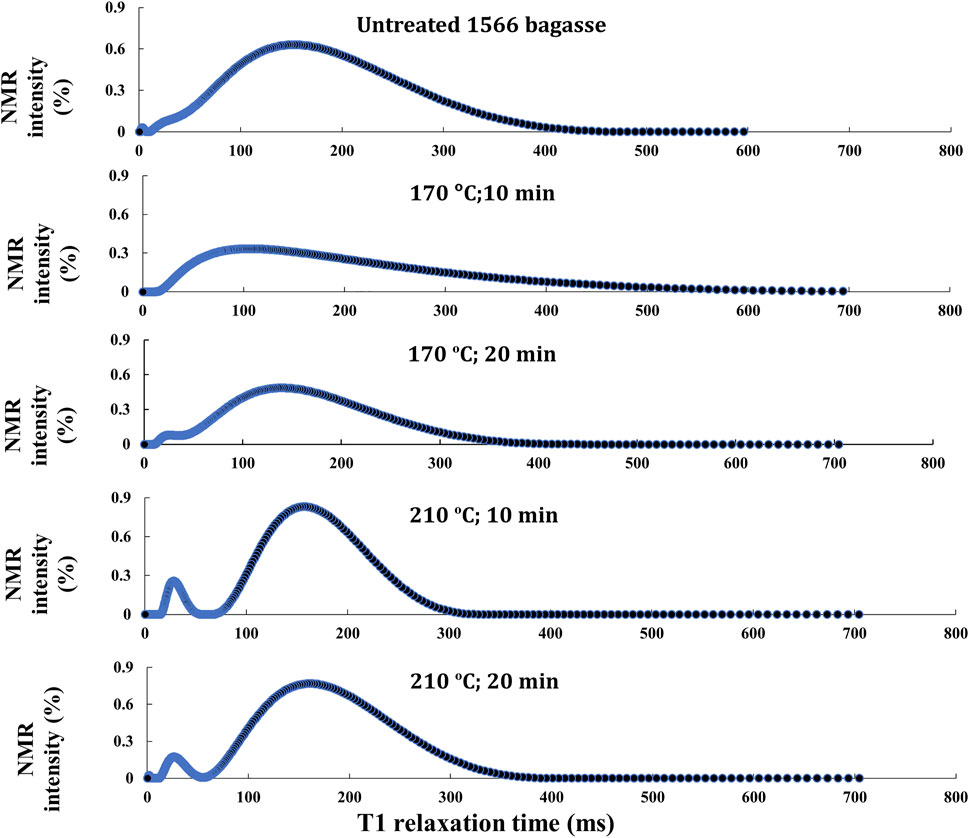
FIGURE 2. T1 relaxation/intensity curve analysis of in situ lipids in the untreated and pretreated bagasse residue of the transgenic oilcane 1566 bagasse.
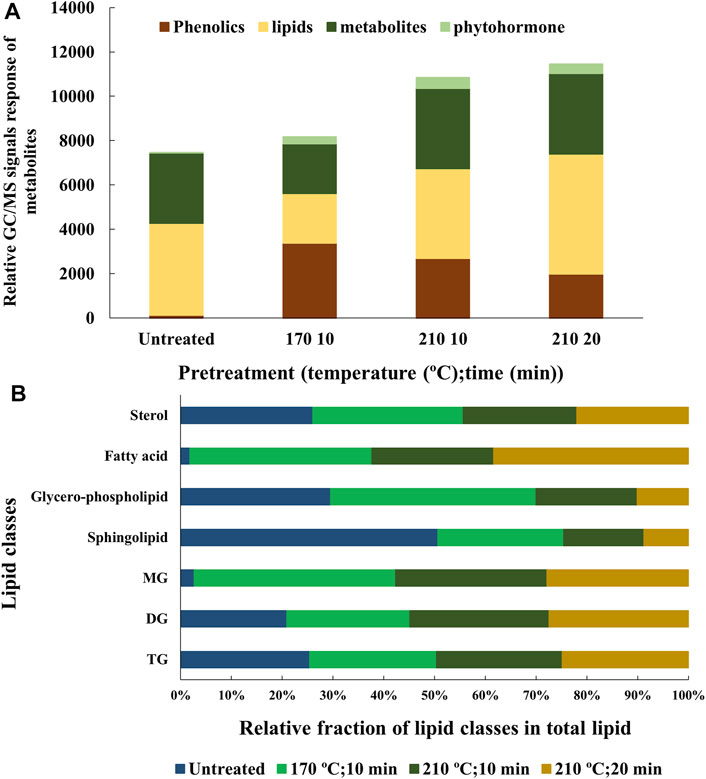
FIGURE 3. (A) GC/MS analysis of total metabolites and (B) LC/MS/MS analysis of various lipid classes of untreated and hydrothermally pretreated transgenic oilcane 1566 bagasse using the organic solvent method. TG, DG, and MG denote triglycerides, diglycerides, and monoglycerides, respectively.
Analyzing Changes in Lipid Composition on Pretreatment
A qualitative GC/MS analysis was performed on the hexane-/IPA-extracted samples from untreated and pretreated transgenic oilcane 1566 bagasse (170°C for 10 min and 210°C for 10 and 20 min). The untreated bagasse sample contained >50% lipids; ∼ 40% plant metabolites such as maleic acid, fumaric acid, salicylic acid, itaconic acid, hydroxyquinone, and their derivatives; and 0.51% of phytohormones like derivatives of phenylacetic acid, indole-acetic acid, and indole-carboxylic acid. Hydrothermal pretreatment significantly increased the generation of phenolic compounds (Figure 3A).
LC/MS/MS analysis was performed to investigate the changes in the composition of lipid classes due to low and high severity hydrothermal pretreatment as compared to the untreated bagasse (Figure 3B). The untreated bagasse sample showed a higher relative percentage of membrane lipids, that is`, phospholipids and sphingolipids and only 0.02% w/w of free fatty acids. Both low and high severity pretreatment procedures aided in decreasing the membrane lipid content. The levels of mono glycerol (MG) and free fatty acids increased on pretreatment, which can be attributed to the thermal decomposition of membrane lipids during hydrothermal pretreatment (Maher and Bressler, 2007). The relative percentage of triglycerides (TGs), diglycerides (DGs), and sterol remained similar after pretreatment.
Analysis of Fatty Acid Composition of Transgenic Bagasse Using TD-NMR
The fatty acid profiles of untreated and pretreated bagasse residues are shown in Figure 4A. A decrease in the percentage of palmitic acid (C16:0) and oleic acid (C18:1) in the pretreated bagasse residue as compared to untreated bagasse indicates that both low- and high-severity pretreatment release fatty acids in the pretreatment liquor upon pretreatment. Pretreatment at 170°C for 10 and 20 min recovered ∼42% palmitic acid (C16:0) (a decrease from 14 to ∼8% C16:0 per g dry biomass), ∼55% oleic acid (C18:1) (a decrease from 38 to ∼17% C18:1 per g dry biomass), and ∼30% linoleic acid (C18:2) (∼9 to ∼6% C18:2 per g dry biomass) in the pretreatment liquor. On the other hand, high-severity pretreatment at 210°C for 10 and 20 min released ∼42% palmitic acid (C16:0) (a decrease from 14 to ∼8% C16:0 per g dry biomass), 39% of oleic acid (C18:1) (a decrease from 38 to ∼23% C18:1 per g dry biomass), and 20% of linoleic acid (C18:2) (a decrease from ∼9 to ∼7% C18:2 per g dry biomass) in the pretreatment liquor. The other fatty acids and lipid classes were concentrated in the bagasse residue on pretreatment. This observation is in agreement with that of the previously reported study (Jia et al., 2020). Pretreatment temperatures beyond 170°C exhibited no significant improvement in the recovery of fatty acids in pretreatment liquor.
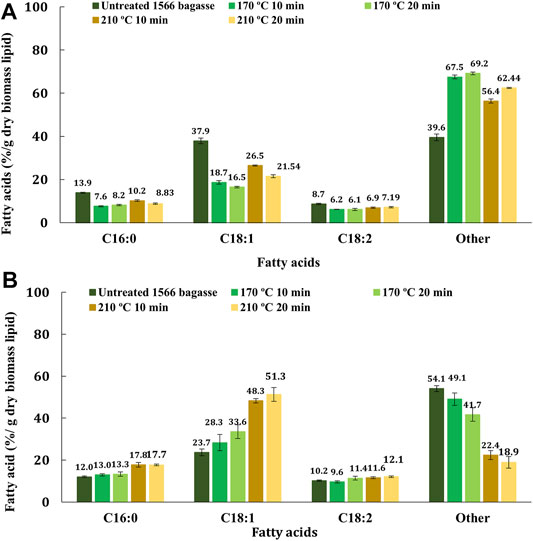
FIGURE 4. Non-invasive analysis of the recovery of three important fatty acids such as palmitic (C16:0), oleic (C18:1), and linoleic (C18:2) acids in the bagasse residues before and after (A) hydrothermal pretreatment and (B) saccharification using TD-NMR.
On the other hand, enzyme hydrolysis aided the recovery of fatty acids other than C16:0, C18:1, and C18:2 by ∼16% (a decrease from 54 to ∼45% per g dry biomass) and ∼63% (a decrease from 54 to ∼20% C per g dry biomass) from 170°C and 210°C pretreated bagasse, respectively (Figure 4B). No further recovery of palmitic (C16:0) or linoleic acids (C18:2) was observed after saccharification. However, bagasse pretreated at 170°C and 210°C showed 1.3 and 2.1 times higher accumulation of oleic acid (C18:1) in the bagasse residue post saccharification, respectively.
Recovery of Fermentable Sugars From Transgenic Oilcane 1566 Bagasse
Besides lipids for biodiesel, fermentable sugars for bioethanol and various value-added bioproducts were recovered from the transgenic oilcane 1566 bagasse. The study categorizes the total sugar yield into solubilized and non-solubilized sugars. The sugars recovered in pretreatment liquor represented the solubilized sugar, while the yield of glucose and xylose obtained post saccharification is denoted as non-solubilized sugar as previously discussed (Maitra and Singh, 2021). Untreated oilcane 1566 bagasse yielded 0.200 ± 0.042 g sugar per g dry biomass on enzyme hydrolysis. Hydrothermal pretreatment at 170°C for 10 and 20 min yielded 0.227 ± 0.004 and 0.268 ± 0.004 g sugar per g dry biomass on saccharification, respectively. Pretreatment at 210°C for 10 and 20 min increased the sugar yield by 1.9 times (0.388 ± 0.003 g/g dry biomass) and 2.3 times (0.457 ± 0.010 g/g dry biomass) post saccharification as compared to the untreated biomass, respectively (Figure 5A). The total fermentable sugar (solubilized + non-solubilized sugars) increased from 20% w/w (untreated biomass) to 50% w/w (pretreated at 210°C), demonstrating >80% recovery of total sugar on pretreatment. However, with an increase in sugar recovery, the yield of inhibitors, specifically acetic acid, also increased significantly beyond the 170°C pretreatment temperature (Figure 5B). The observation is in agreement with that in our previous study that inhibitor generation increases exponentially beyond 170°C in hydrothermal pretreatment (Maitra and Singh, 2021).
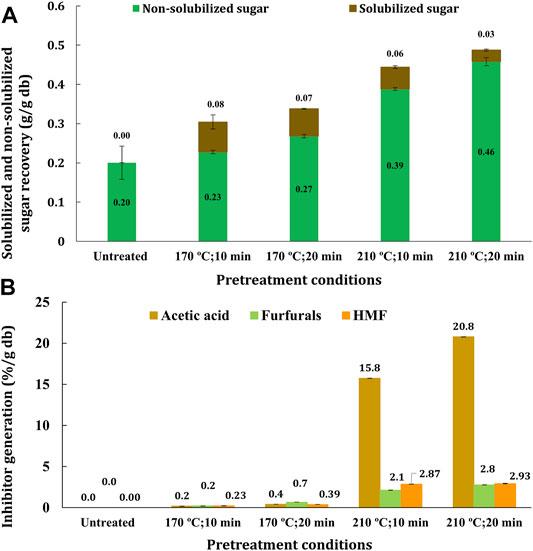
FIGURE 5. (A) Total sugar recovered and (B) inhibitors generated during hydrothermal pretreatment of transgenic oilcane 1566 bagasse.
Lipid and Ethanol Yield After Simultaneous Saccharification and Fermentation (SSF)
Since ethanol has lipid-solubilizing property, simultaneous saccharification and fermentation (SSF) were performed with untreated and pretreated (170°C and 210°C for 20 min) bagasse to examine the recovery of lipids in the post-fermentation broth as observed in the corn dry–grind process (Moreau et al., 2010; Luangthongkam et al., 2015). The release of lipids from the cellulosic biomass after SSF is evident from the decrease in the magnitude of T1 relaxation time of pretreated bagasse samples (Table 1). A left shift in relaxation/intensity curves of the pretreated bagasse residue after SSF in Figure 6A indicates a reduction in the degree of freedom of lipid-associated proton molecules of the pretreated bagasse samples (Maitra et al., 2021). An increase in NMR intensity could be due to the contribution of proton molecules from citrate buffer, yeast, or enzymes used in SSF. The lipid released during fermentation can be present in different forms, such as oil-in-water emulsion, oil inside unbroken oil bodies, and oil droplets attached to cellulosic biomass (Luangthongkam et al., 2015). However, unlike the dry–grind process, due to the low lipid content in transgenic oilcane 1566 bagasse, most of the lipids remained either attached to or accumulated in the cellulosic biomass. Thus, an increase in the total lipid content of the pretreated bagasse residue after SSF was observed (Figure 6B). The fatty acid composition of the bagasse residue after SSF was similar to that of post-saccharification (Figure 6C).
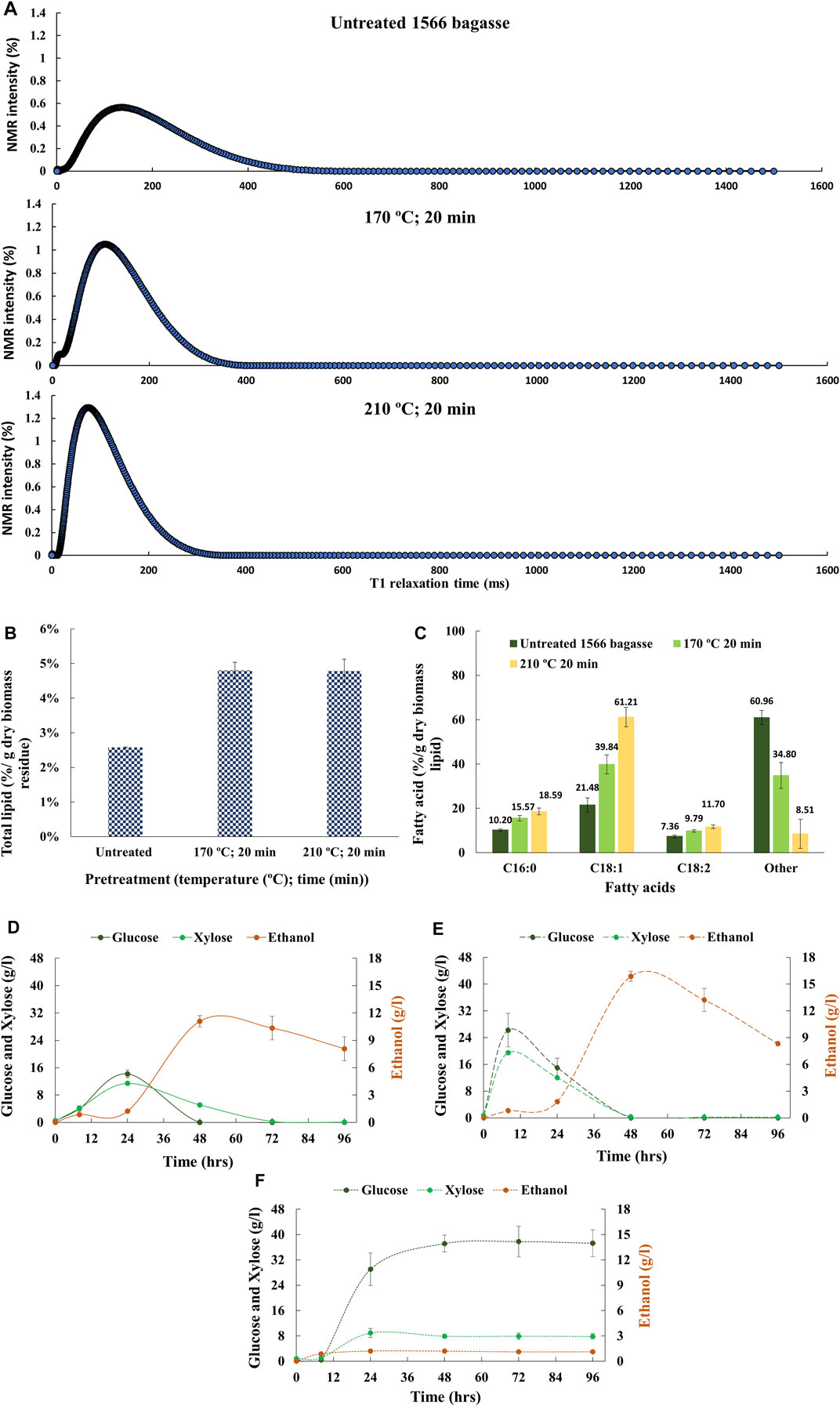
FIGURE 6. (A) T1 relaxation/intensity curves analysis of in situ lipids, (B) amount of total in situ lipids, and (C) fatty acid profile of lipids in the untreated and pretreated bagasse residues after SSF. Sugar and ethanol profile of (D) untreated and hydrothermally pretreated oilcane 1566 bagasse, that is, (E) 170°C for 20 min, and (F) 210°C for 20 min during SSF.
The sugar and ethanol profiles of untreated and pretreated bagasse during SSF are presented in Figures 6D–F. Oilcane 1566 bagasse pretreated at 170°C for 20 min resulted in the best outcome for enhanced biofuel yield. It yielded a maximum ethanol concentration of 15.3 g/l which is 23.7 and 92.1% higher than ethanol obtained from untreated bagasse (11.6 g/l) and bagasse pretreated at 210°C for 20 min (1.2 g/l), respectively. Glucose and xylose were consumed simultaneously and completely within 48 h of fermentation (Figure 6E). A decrease in ethanol concentration after 55 h was observed that could be due to the consumption of ethanol by yeast as a carbon source after complete consumption of sugars (Figures 6D, E) (Raamsdonk et al., 2001). It has been observed in several fermentation studies with both Crabtree positive and negative yeasts that once all the sugars in the fermentation medium are consumed, yeasts start to consume sugar metabolism products as a carbon source and proliferate slowly (Polakis and Bartley, 1965; Skoog et al., 1992). Unfortunately, due to high concentration of inhibitors (Figure 5B), yeast could not grow well on bagasse pretreated at 210°C for 20 min. No significant change in sugar consumption or ethanol production was observed over 96 h of fermentation (Figure 6F). High-severity pretreatment generates inhibitory compounds that restrict the growth of yeast and reduce ethanol yield (Figures 5B, 6F). Removal of inhibitors could improve the fermentation efficiency of high-severity–pretreated bagasse.
Discussion
Recovery and Stability of Lipids During Bioprocess Steps
Plant tissues have a variety of lipid classes present in both bound and free forms. Triacylglyceride (TAG) molecules in the transgenic oilcane 1566 accumulate in the form of droplets inside the vegetative tissues (Parajuli et al., 2020). Recovery of a fraction of these free lipids in the pretreatment liquor decreases the cost of subsequent solvent extraction. The present NMR study showed that hydrothermal pretreatment and saccharification release ∼25% of total lipids in pretreatment liquor and 45–60% of the remaining lipids in hydrolyzate, respectively. The remaining bound lipids in the cellulosic biomass can be extracted at the end of the bioprocess using organic solvent extraction (Huang et al., 2017).
Analysis of the stability of in situ lipids in the transgenic cellulosic biomass upon pretreatment is critical to optimizing the pretreatment parameters. To this end, time-domain proton NMR spectroscopy can detect minute alterations in the degree of freedom of oil-associated proton nuclei in the bagasse residues after various pretreatment procedures. Changes can be observed in the NMR intensity and the magnitude of relaxation time distribution (Robinson and Cistola, 2014; Nikolskaya and Hiltunen, 2020; Maitra et al., 2021). The T1 relaxation/intensity curves also provide information on the decomposition of lipids in the pretreated bagasse residue upon pretreatment. In the previous study, the authors demonstrated the effects of hydrothermal, dilute-acid, and alkali pretreatment on model biomass systems (bagasse with externally added crude corn oil). In the alkaline pretreated biomass, alkali caused saponification of the oil in the biomass which resulted in inconsistent relaxation/intensity curves, indicating the decomposition of oil due to the saponification reaction (Maitra et al., 2021). Unlike the NMR relaxation/intensity curves of alkali-pretreated biomass, both low- and high-severity hydrothermal pretreatment retain the stability of in situ lipids (Figure 2).
The Composition of Lipids Recovered Decides the Quality of Biodiesel
In addition to the stability of lipids, the lipid classes and the fatty acid profile of the lipids in the transgenic bioenergy crops are crucial as they decide the properties of biodiesel such as oxidative stability, freezing point, heating value, lubricity, kinematic viscosity, and cloud point (Knothe and Gerpen, 2005; Knothe, 2008). The approach of hyperaccumulation of lipids in transgenic bioenergy crops also aims to improve the percentage of desirable fatty acids. Therefore, during the development and optimization of the pretreatment protocol for transgenic bioenergy crops, it is necessary to analyze the fatty acid composition of lipids after each step of bioprocessing. TD-NMR provides a rapid and convenient screening method during the initial developmental stages. The TD-NMR technique utilizes the specific T1T2 relaxometry spectra of saturated (SFA), unsaturated (UFA), and polyunsaturated (PUFA) fatty acids. Each fatty acid exhibits distinct spectra due to the nanofluidity of the hydrocarbon side chains and molecular properties (Robinson and Cistola, 2014; Nikolskaya and Hiltunen, 2018, 2019). The fatty acid composition of seed oils is routinely analyzed using NMR (Ebrahimi et al., 2017; Engelsen and van den Berg, 2017; Gottstein et al., 2019). The present study extends the application of the TD-NMR technique for determining the fatty acid profile of lipids in cellulosic biomass residues subsequent to each bioprocessing step.
TD-NMR analysis of the oil-associated proton fluidity (Figure 2) and fatty acid profile (Figure 4A) of untreated and pretreated bagasse residues confirmed the release of lipid molecules in the pretreatment liquor upon pretreatment. However, bagasse residues obtained post saccharification showed a relatively higher percentage of C16:0, C18:1, and C18:2 fatty acids (Figure 4B). This can be attributed to the deconstruction of the biomass residue to release sugars and the accumulation of membrane-bound lipids in the remaining bagasse residue. An increased fraction of C18:1 (oleic) was observed in the pretreated biomass post saccharification. C18 unsaturated fatty acids are one of the predominant fatty acids in plants. Since both pretreatment and saccharification solubilize the structural carbohydrates, an increase in the percentage of oleic acid (C18:1) in the pretreated bagasse residue after saccharification can be attributed to its higher occurrence as part of membrane-bound lipids (Reszczyńska and Hanaka, 2020). A higher percentage of C18 unsaturated fatty acids, specifically oleic acid in the lipids recovered from transgenic crops, aids the improvement in biodiesel quality by balancing the oxidative stability and cold flow of biodiesel without affecting the cetane number (Knothe, 2009). Interestingly, genetically modification expressed higher average values of oleic fatty acid in the transgenic oilcane bagasse which is best suited for biodiesel.
Enhanced Biofuel Yield From Transgenic Oilcane 1566 Bagasse
Both low- and high-severity chemical-free hydrothermal pretreatment of the transgenic oilcane 1566 bagasse efficiently recover lipids in pretreatment liquor and hydrolyzate, while remaining lipids get concentrated in the bagasse residue that could be recovered post fermentation for biodiesel production. High-severity hydrothermal pretreatment (210°C for 10 and 20 min) improved recovery of various lipid classes as compared to low severity pretreatment (170°C for 10 min) (Figure 3A). However, unfortunately, high-severity pretreatment produced >15%, >2.5%, >2% per g dry biomass of acetic acid, HMF, and furfurals, respectively (Figure 5B). The high concentration of inhibitory compounds restricted the growth and fermentation process of yeast during SSF, which limited the optimal production of bioethanol (Figure 6F), even though the recovery of lipids (Figure 3A) and fermentable sugars on saccharification was higher (Figure 5A). In hydrothermal pretreatment, the amount of inhibitory compounds generated is a function of biomass type, moisture content during pretreatment, and pretreatment time and temperature (Ximenes et al., 2011; Maitra and Singh, 2021). To this end, the low-severity pretreatment of bagasse recovered comparable lipids, ∼35% w/w fermentable sugars, and significantly fewer inhibitory compounds that resulted in enhanced biofuel yield (lipids for biodiesel and bioethanol). Hence, hydrothermal pretreatment of transgenic oilcane 1566 bagasse at 170°C for 20 min provides optimum pretreatment conditions to balance the maximum recovery of lipid and fermentable sugar and minimal generation of inhibitors.
Conclusion
The study evaluates and presents chemical-free hydrothermal pretreatment at 170°C for 20 min as an optimized condition that balances the maximum recovery of lipid and fermentable sugars and minimal generation of inhibitors from oilcane 1566 bagasse. The NMR relaxometry spectra revealed that hydrothermal pretreatment prevents the decomposition of in situ lipids of transgenic bioenergy crops during the recovery process. Moreover, the study successfully demonstrates the application of time-domain 1H-NMR spectroscopy in the field of bioprocessing for quantification of total lipids in cellulosic biomass, characterization of in-situ lipids into bound and free fractions, and determining the fatty acid composition of cellulosic biomass. The use of NMR spectroscopy has significantly sped up the analysis.
Data Availability Statement
The original contributions presented in the study are included in the article/Supplementary Material, further inquiries can be directed to the corresponding author.
Author Contributions
SM: conceptualization, experiments, data analysis, and manuscript drafting. SL: Development of transgenic plant, supervision, reviewing, and editing the manuscript. VS: conceptualization, supervision, reviewing and editing the manuscript, and funding acquisition.
Funding
This work was funded by the DOE Center for Advanced Bioenergy and Bioproducts Innovation (United States Department of Energy, Office of Science, Office of Biological and Environmental Research under Award Number DE-SC0018420.
Author Disclaimer
Any opinions, findings and conclusions or recommendations expressed in this work are those of the author(s) and do not necessarily reflect the views of the United States Department of Energy.
Conflict of Interest
The authors declare that the research was conducted in the absence of any commercial or financial relationships that could be construed as a potential conflict of interest.
Publisher’s Note
All claims expressed in this article are solely those of the authors and do not necessarily represent those of their affiliated organizations, or those of the publisher, the editors, and the reviewers. Any product that may be evaluated in this article, or claim that may be made by its manufacturer, is not guaranteed or endorsed by the publisher.
Supplementary Material
The Supplementary Material for this article can be found online at: https://www.frontiersin.org/articles/10.3389/fenrg.2022.840418/full#supplementary-material
References
Atabani, A. E., Silitonga, A. S., Ong, H. C., Mahlia, T. M. I., Masjuki, H. H., Badruddin, I. A., et al. (2013). Non-edible Vegetable Oils: A Critical Evaluation of Oil Extraction, Fatty Acid Compositions, Biodiesel Production, Characteristics, Engine Performance and Emissions Production. Renew. Sustain. Energ. Rev. 18, 211–245. doi:10.1016/j.rser.2012.10.013
Berman, P., Leshem, A., Etziony, O., Levi, O., Parmet, Y., Saunders, M., et al. (2013). Novel 1H Low Field Nuclear Magnetic Resonance Applications for the Field of Biodiesel. Biotechnol. Biofuels 6, 55. doi:10.1186/1754-6834-6-55
Bhuiya, M. M. K., Rasul, M. G., Khan, M. M. K., Ashwath, N., and Azad, A. K. (2016). Prospects of 2nd Generation Biodiesel as a Sustainable Fuel-Part: 1 Selection of Feedstocks, Oil Extraction Techniques and Conversion Technologies. Renew. Sustain. Energ. Rev. 55, 1109–1128. doi:10.1016/j.rser.2015.04.163
Ebrahimi, P., Viereck, N., Bro, R., and Engelsen, S. B. (2017). Chemometric Analysis of NMR Spectra. Mod. Magn. Reson., 1–20. doi:10.1007/978-3-319-28275-6_20-1
Engelsen, S. B., and van den Berg, F. W. J. (2017). “Quantitative Analysis of Time Domain NMR Relaxation Data,” in Modern Magnetic Resonance. Editor G. A. Webb (Cham: Springer International Publishing), 1–19. doi:10.1007/978-3-319-28275-6_21-1
Gottstein, V., Müller, M., Günther, J., Kuballa, T., and Vetter, W. (2019). Direct 1H NMR Quantitation of Valuable Furan Fatty Acids in Fish Oils and Fish Oil Fractions. J. Agric. Food Chem. 67, 11788–11795. doi:10.1021/acs.jafc.9b04711
Huang, H., Long, S. P., Clemente, T. E., and Singh, V. (2016a). Technoeconomic Analysis of Biodiesel and Ethanol Production from Lipid-Producing Sugarcane and Sweet Sorghum. Ind. Biotechnol. 12, 357–365. doi:10.1089/ind.2016.0013
Huang, H., Long, S., and Singh, V. (2016b). Techno‐economic Analysis of Biodiesel and Ethanol Co‐production from Lipid‐producing Sugarcane. Biofuels, Bioprod. Bioref. 10, 299–315. doi:10.1002/bbb.1640
Huang, H., Moreau, R. A., Powell, M. J., Wang, Z., Kannan, B., Altpeter, F., et al. (2017). Evaluation of the Quantity and Composition of Sugars and Lipid in the Juice and Bagasse of Lipid Producing Sugarcane. Biocatal. Agric. Biotechnol. 10, 148–155. doi:10.1016/j.bcab.2017.03.003
Jia, Y., Kumar, D., Winkler-Moser, J. K., Dien, B., and Singh, V. (2020). Recoveries of Oil and Hydrolyzed Sugars from Corn Germ Meal by Hydrothermal Pretreatment: A Model Feedstock for Lipid-Producing Energy Crops. Energies 13, 6022. doi:10.3390/en13226022
Knothe, G., and Gerpen, J. V. (2005). The Biodiesel Handbook. Editors G. Knothe, J Van Gerpen, and J. Krahl (AOCS Publishing). Available at: https://www.taylorfrancis.com/books/9781439822357.
Knothe, G. (2008). "Designer" Biodiesel: Optimizing Fatty Ester Composition to Improve Fuel Properties. Energy Fuels 22, 1358–1364. doi:10.1021/ef700639e
Knothe, G. (2009). Improving Biodiesel Fuel Properties by Modifying Fatty Ester Composition. Energy Environ. Sci. 2, 759–766. doi:10.1039/b903941d
Luangthongkam, P., Fang, L., Noomhorm, A., and Lamsal, B. (2015). Addition of Cellulolytic Enzymes and Phytase for Improving Ethanol Fermentation Performance and Oil Recovery in Corn Dry Grind Process. Ind. Crops Prod. 77, 803–808. doi:10.1016/j.indcrop.2015.09.060
Maher, K. D., and Bressler, D. C. (2007). Pyrolysis of Triglyceride Materials for the Production of Renewable Fuels and Chemicals. Bioresour. Technol. 98, 2351–2368. doi:10.1016/j.biortech.2006.10.025
Maitra, S., and Singh, V. (2021). Balancing Sugar Recovery and Inhibitor Generation during Energycane Processing: Coupling Cryogenic Grinding with Hydrothermal Pretreatment at Low Temperatures. Bioresour. Technol. 321, 124424. doi:10.1016/j.biortech.2020.124424
Maitra, S., Dien, B., Long, S. P., and Singh, V. (2021). Development and Validation of Time‐domain 1 H‐NMR Relaxometry Correlation for High‐throughput Phenotyping Method for Lipid Contents of Lignocellulosic Feedstocks. GCB Bioenergy 13, 1179–1190. doi:10.1111/gcbb.12841
Moreau, R. A., Hicks, K. B., Johnston, D. B., and Laun, N. P. (2010). The Composition of Crude Corn Oil Recovered after Fermentation via Centrifugation from a Commercial Dry Grind Ethanol Process. J. Am. Oil Chem. Soc. 87, 895–902. doi:10.1007/s11746-010-1568-z
Nascimento, P. A. M., Barsanelli, P. L., Rebellato, A. P., Pallone, J. A. L., Colnago, L. A., and Pereira, F. M. V. (2017). Time-domain Nuclear Magnetic Resonance (TD-NMR) and Chemometrics for Determination of Fat Content in Commercial Products of Milk Powder. J. AOAC Int. 100, 330–334. doi:10.5740/jaoacint.16-0408
Nikolskaya, E., and Hiltunen, Y. (2018). Determination of Carbon Chain Lengths of Fatty Acid Mixtures by Time Domain NMR. Appl. Magn. Reson. 49, 185–193. doi:10.1007/s00723-017-0953-2
Nikolskaya, E., and Hiltunen, Y. (2019). Molecular Properties of Fatty Acid Mixtures Estimated by Online Time-Domain NMR. Appl. Magn. Reson. 50, 159–170. doi:10.1007/s00723-018-1046-6
Nikolskaya, E., and Hiltunen, Y. (2020). Time-Domain NMR in Characterization of Liquid Fuels: A Mini-Review. Energy Fuels 34, 7929–7934. doi:10.1021/acs.energyfuels.0c01464
Overend, R. P., Chornet, E., and Gascoigne, J. A. (1987). Fractionation of Lignocellulosics by Steam-Aqueous Pretreatments. Phil. Trans. R. Soc. Lond. A. 321, 523–536. doi:10.1098/rsta.1987.0029
Parajuli, S., Kannan, B., Karan, R., Sanahuja, G., Liu, H., Garcia‐Ruiz, E., et al. (2020). Towards Oilcane: Engineering Hyperaccumulation of Triacylglycerol into Sugarcane Stems. GCB Bioenergy 12, 476–490. doi:10.1111/gcbb.12684
Polakis, E., and Bartley, W. (1965). Changes in the Enzyme Activities of Saccharomyces cerevisiae during Aerobic Growth on Different Carbon Sources. Biochem. J. 97, 284–297. doi:10.1042/bj0970284
Raamsdonk, L. o. M., Diderich, J. A., Kuiper, A., van Gaalen, M., Kruckberg, A. L., Berden, J. A., et al. (2001). Co-Consumption of Sugars or Ethanol and Glucose in aSaccharomyces Cerevisiae Strain Deleted in theHXK2 Gene. Yeast 18, 1023–1033. doi:10.1002/yea.746
REN21 (2020). Renewables 2020 Global Status Report. Available at: https://www.ren21.net/wp-content/uploads/2019/05/GSR_2020_Press_Release_ES.pdf (Accessed September 15, 2021).
Resch, M. G., Baker, J. O., and Decker, S. R. (2015). Low Solids Enzymatic Saccharification of Lignocellulosic Biomass (Laboratory Analytical Procedure). Available at: https://linkinghub.elsevier.com/retrieve/pii/S0925838818308703 (Accessed December 15, 2020).
Reszczyńska, E., and Hanaka, A. (2020). Lipids Composition in Plant Membranes. Cell Biochem. Biophys. 78, 401–414. doi:10.1007/s12013-020-00947-w
Robinson, M. D., and Cistola, D. P. (2014). Nanofluidity of Fatty Acid Hydrocarbon Chains as Monitored by Benchtop Time-Domain Nuclear Magnetic Resonance. Biochemistry 53, 7515–7522. doi:10.1021/bi5011859
Skoog, K., Hahn-Hägerdal, B., Degn, H., Jacobsen, J. P., and Jacobsen, H. S. (1992). Ethanol Reassimilation and Ethanol Tolerance in Pichia Stipitis CBS 6054 as Studied by 13 C Nuclear Magnetic Resonance Spectroscopy. Appl. Environ. Microbiol. 58, 2552–2558. doi:10.1128/AEM.58.8.2552-2558.1992
Vanhercke, T., Belide, S., Taylor, M. C., El Tahchy, A., Okada, S., Rolland, V., et al. (2019). Up-regulation of Lipid Biosynthesis Increases the Oil Content in Leaves ofSorghum Bicolor. Plant Biotechnol. J. 17, 220–232. doi:10.1111/pbi.12959
Ximenes, E., Kim, Y., Mosier, N., Dien, B., and Ladisch, M. (2011). Deactivation of Cellulases by Phenols. Enzyme Microb. Technol. 48, 54–60. doi:10.1016/j.enzmictec.2010.09.006
Keywords: biofuels (biodiesel and bioethanol), hydrothermal pretreatment, time-domain 1H NMR spectroscopy, lipid analysis, bioenergy crops
Citation: Maitra S, Long SP and Singh V (2022) Optimizing Chemical-Free Pretreatment for Maximizing Oil/Lipid Recovery From Transgenic Bioenergy Crops and Its Rapid Analysis Using Time Domain-NMR. Front. Energy Res. 10:840418. doi: 10.3389/fenrg.2022.840418
Received: 21 December 2021; Accepted: 21 March 2022;
Published: 27 April 2022.
Edited by:
Allison E. Ray, Idaho National Laboratory (DOE), United StatesReviewed by:
Somnath D. Shinde, Conagen Inc., United StatesCorey Pilgrim, Idaho National Laboratory (DOE), United States
Copyright © 2022 Maitra, Long and Singh. This is an open-access article distributed under the terms of the Creative Commons Attribution License (CC BY). The use, distribution or reproduction in other forums is permitted, provided the original author(s) and the copyright owner(s) are credited and that the original publication in this journal is cited, in accordance with accepted academic practice. No use, distribution or reproduction is permitted which does not comply with these terms.
*Correspondence: Vijay Singh, dnNpbmdoQGlsbGlub2lzLmVkdQ==