- 1Department of Environmental Microbiology, Helmholtz-Centre for Environmental Research—UFZ, Leipzig, Germany
- 2Department Solar Materials, Helmholtz-Centre for Environmental Research—UFZ, Leipzig, Germany
Physiological cellular parameters, such as latency times (lagt), cell production rates (CPR), doubling times (td), relative growth rates (RGR), and yield coefficients (YNe), are only known as endpoint measurements for electroactive microorganisms (EAM). Here we show that these can be gained non-invasively and in real-time for early-stage biofilm formation at electrodes using a microfluidic electrochemical flow-cell (EFC) allowing in vivo optical microscopy. Parameters obtained for early-stage mixed culture biofilm anodes formed at +150 mV vs. Ag/AgCl sat. KCl have lagt of 2.31–4.58 days, CPR of 0.72–1.20 × 105 cells h−1, td of 176.54–1838.65 min, RGR of 0.02–0.27 h−1, and YNe of 5.99–7.94 × 1012 cells mole-−1. However, oxygen permeation into the EFC was the main problem that remained unsolved during the study that provides interesting lessons for future improvements.
1 Introduction
Electroactive microorganisms (EAM) use extracellular electron transfer (EET) to exchange electrons for the metabolism with their environment (Lovley, 2012). Solid-state minerals or electrodes are used as the terminal electron acceptor (TEA), the latter enabling coupling of the EAM metabolism to an external electric circuit. EET can be direct, indirect, or mediated (Lovley, 2012; Ikeda et al., 2021; Lovley and Holmes, 2021). When direct EET is used, a physical contact between the outer membrane cytochromes and the electrode is required resulting in the formation of electroactive biofilms. The model organisms for direct EET are Geobacteraceae that form thick anodic biofilms (>100 µm) with high electrical conductivity (Lovley, 2012; Lovley and Walker, 2019; Wang et al., 2019), whereas the other model organism Shewanella can perform both direct and mediated EET (Baron et al., 2009; Ikeda et al., 2021). EAM find several applications in primary microbial electrochemical technologies (MET) (Schröder, 2011; Logan et al., 2019). The beating heart of all primary MET based on EAM performing direct EET is the electroactive biofilm at the anode. However, there is still a significant lack of knowledge on fundamental physiological properties such as cell production rates (CPR), relative growth rates (RGR), and yield coefficients (YNe), particularly for mixed culture electroactive biofilms. These kinds of data are highly relevant for fundamental research, for example, as modeling parameters, and set the foundation for prospective bioprocess development. Reported fundamental physiological parameters for EAM were determined either by studying cells growing planktonically on soluble TEA such as fumarate or Fe(III) or on carbon-based electrodes (Brown et al., 2005; Esteve-Nunez et al., 2005; Heidrich et al., 2016). As polycrystalline carbon or graphite is optically not transparent, markers that allow not only end-point measurements are needed. Recently, using transparent AuPd anodes, we determined latency times (lagt) and YNe for pure-culture early-stage electroactive biofilms of G. sulfurreducens (Scarabotti et al., 2021; Scarabotti et al., 2022). This required cell staining for performing confocal laser scanning microscopy (CLSM) for single-cell determination. Thus, only end-point measurements were possible and the biofilms needed to be “sacrificed” for each measurement. Here, we report determining lagt and YNe determined using an electrochemical flow-cell (EFC), permitting non-invasive and real-time analysis by optical microscopy for in vivo studies.
2 Materials and Methods
Chemicals of at least analytical grade from Carl Roth GmbH (Karlsruhe, Germany) and Merck KGaA (Darmstadt, Germany) and de-ionized water (Millipore, Darmstadt, Germany) were used. Provided potentials refer to the Ag/AgCl sat. KCl (+197 mV vs. standard hydrogen electrode, SHE).
2.1 Microorganism, Cultivation Media and Pre-culture
Enrichment cultures derived from wastewater inoculum and mainly dominated by Geobacter anodireducens served as the inoculum (Gimkiewicz and Harnisch, 2013; Korth et al., 2020a; Korth et al., 2020b). Two pre-cultures (pre-cultures 1 and 2) of two biofilm electrodes in double-chamber four-neck round-bottom flasks, hereafter referred to as electrochemical batch reactors (EBR), were used as the inoculum for all experiments (Supplementary Section S1.1).
2.2 Electrochemical Flow-Cell
The electrochemical flow-cell (EFC) was custom built based on a standard flow-cell (BioCentrum, Technical University of Denmark) (Weiss Nielsen et al., 2011), which was modified to host a three-electrode system for the electrochemical cultivation in anaerobic conditions. An Ag/AgCl pseudo-reference electrode (pseudo-RE) (Polk et al., 2006), a titanium wire as the counter electrode (CE), and a transparent AuPd working electrode (WE) (Scarabotti et al., 2021, Scarabotti et al., 2022) were implemented (Figure 1A; for the fabrication procedure, refer Supplementary Sections S3.4–S3.7).
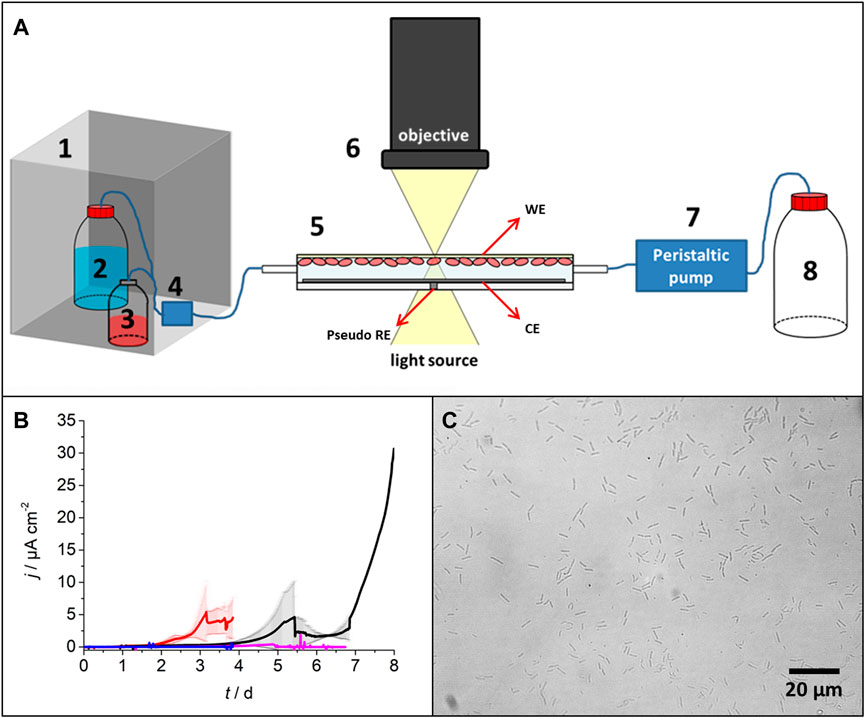
FIGURE 1. (A) Schematic experimental setup including the anaerobic box (1), medium bottle (2), inoculum (3), bubble trap from Weiss Nielsen et al. (2011) (4), electrochemical flow-cell (EFC) with a pseudo-reference electrode (pseudo-RE), working electrode (WE) and counter electrode (CE) (5), microscope (6), peristaltic pump (7), and waste bottle (8). (B) Chronoamperometric measurement of the biofilm growing on the AuPd WE at +150 mV vs. Ag/AgCl sat. KCl in the EFC. Red and black lines indicate the average value of the current density of the experiments performed with pre-cultures 1 and 2 as the inoculum, respectively (each n = 3). Red and black shadowed areas indicate standard deviations. Blue line: negative control without inoculation; magenta line: biofilm cultivation in the presence of additional 50 mmol L−1 2-BES. (C) Optical microscopy picture of the AuPd WE with cells. Experiments were carried out till a multi-layered biofilm started to appear (Section 2.4 and Supplementary Figure S3).
2.3 Electrochemical Experiments
Figure 1A shows the experimental setup consisting of an anaerobic box (1) containing the medium bottle (2), the inoculum pre-culture (3), the bubble trap from Weiss Nielsen et al. (2011) (4), the EFC (5), the optical microscope (6) (BH2 Olympus equipped with a 40X air objective and an XC30 camera, Olympus), and the peristaltic pump (7) (4-channel pump, ISMATEC REGLO ICC®). The setup was positioned on a grounded electrostatic mat. An electrostatic bracelet connected to the electrostatic mat and to the ground was worn when handling the experiment. Tubing was generally E-3603 Tygon (ID 1.6 mm, OD 3.2 mm, wall 0.8 mm, ACF00002-C, Saint-Gobain performance plastics, Charny, France); tubing for the peristaltic pump and the tubing located in the anaerobic box were made of silicon or PTFE. All the components and the assembled EFC were sterilized under UV light for at least 2 h, while the silicon/PTFE tubing and the medium bottle were autoclaved. The anaerobic box and the medium bottle were continuously purged with nitrogen gas (6 mL min−1). Before each experiment, the EFC was flushed with a sterile anaerobic medium for 30 min at 2.5 mL min−1. Microscopic pictures on the transparent AuPd WE were taken before inoculation of each experiment to ensure the absence of contamination. Subsequently, the EFC was emptied using nitrogen gas and inoculated with the prepared pre-cultures according to Supplementary Section S1.1. Air bubbles were removed, and the flow rate was set to 2.5 μL min−1. Chronoamperometry (CA) at 0 mV (being +150 mV vs. Ag/AgCl sat. KCl, Supplementary Figure S1, Supplementary Section S3.5) or open-circuit potential (OCP) measurements were applied to the EFC using a potentiostat (SP-200, Biologic®, Claix, France) equipped with an ultra-low current detection module. After 24 h of continuous inoculation, a sterile fresh medium supplemented with 5 mmol L−1 acetate was continuously pumped through the EFC. For some experiments, an additional 50 mmol L−1 of 2-BES (2-bromoethanesulfonate) was added to prevent the growth of archaea (Kosse et al., 2016; Webster et al., 2016). As control experiments, chronoamperometric cultivation at +150 mV vs. Ag/AgCl sat. KCl and OCP were performed in the EBR.
2.4 Microscopy Pictures Analysis
Per each time point, ten pictures were acquired (five pictures on the left and right of the WE, respectively; Supplementary Figure S2). The pictures were analyzed (ImageJ 1.45 s, Java 1.8.0_202, 32-bit) for cell counting. Cell counting was possible till a multilayer biofilm started to appear (Supplementary Section S1.2; Supplementary Figures S3, S4).
2.5 Sampling and Microbial Community Analysis
The inoculum was sampled before each experiment (Supplementary Section S1.1), while the biofilms of the EFC and EBR were sampled at the end of each experiment under sterile conditions. Genomic DNA was extracted, and PCR and amplicon sequencing of the bacterial 16S rRNA gene and archaeal mcrA gene were performed (Supplementary Sections S1.3, S1.4).
2.6 Calculations of Latency Times, Relative Growth Rates, Cell Production Rates, Doubling Times, and Yield Coefficients
In line with Scarabotti et al. (2021) and Scarabotti et al. (2022), lagt was defined as the time from inoculation until j ≥ 1 μA cm−2. The cell number (Ncell) at a time point
with the slope being the angular coefficient of the linear regression from data points plotted with time (t, x-axis) and number of cells (Ncell, y-axis).
with Ncell,t2 and Ncell,t1 being the number of cells counted at
with the slope being the angular coefficient of the linear regression on data points plotted as the measured charge (Q, x-axis) versus the cell number (Ncell, y-axis) and F as the Faraday constant (96,485 C mole-−1).
3 Results and Discussion
3.1 Chronoamperometric Cultivation and Determination of Relative Growth Rates, Doubling Times, and Yield Coefficients
Figure 1B shows the chronoamperometric cultivation of electroactive biofilms in the EFC on AuPd WE at +150 mV vs. Ag/AgCl sat. KCl. The current profiles based on pre-cultures 1 and 2 were similar, but their latency differed significantly. For pre-culture 1, lagt was 2.31 ± 0.20 days (n = 3), whereas for pre-culture 2, lagt was 4.58 ± 1.14 days (n = 3). Thus, lagt are in accordance with our previous studies (Scarabotti et al., 2021; Scarabotti et al., 2022) for G. sulfurreducens cultured in single- or double-chamber reactors on the AuPd WE (Table 1). Only at −200 mV vs. Ag/AgCl sat. KCl in double-chamber reactors G. sulfurreducens did show a lower lagt of 0.24 ± 0.26 days (n = 7). The shortest lagt of 0.05 ± 0.03 days (n = 3) was observed for biofilms on graphite anodes at +200 mV vs. Ag/AgCl sat. KCl in double-chamber reactors, which can be assigned to the different electrode materials (Table 1). Differences in latency were also observed by other researchers and might reflect the intrinsic heterogeneity in the microbial communities and the stochasticity of the initial settling phase during biofilm formation (Molenaar et al., 2018). The 16S rRNA gene sequencing data (Supplementary Section S1.3) show that the bacterial community composition of the biofilms in the EFC strongly differs from the inoculum.
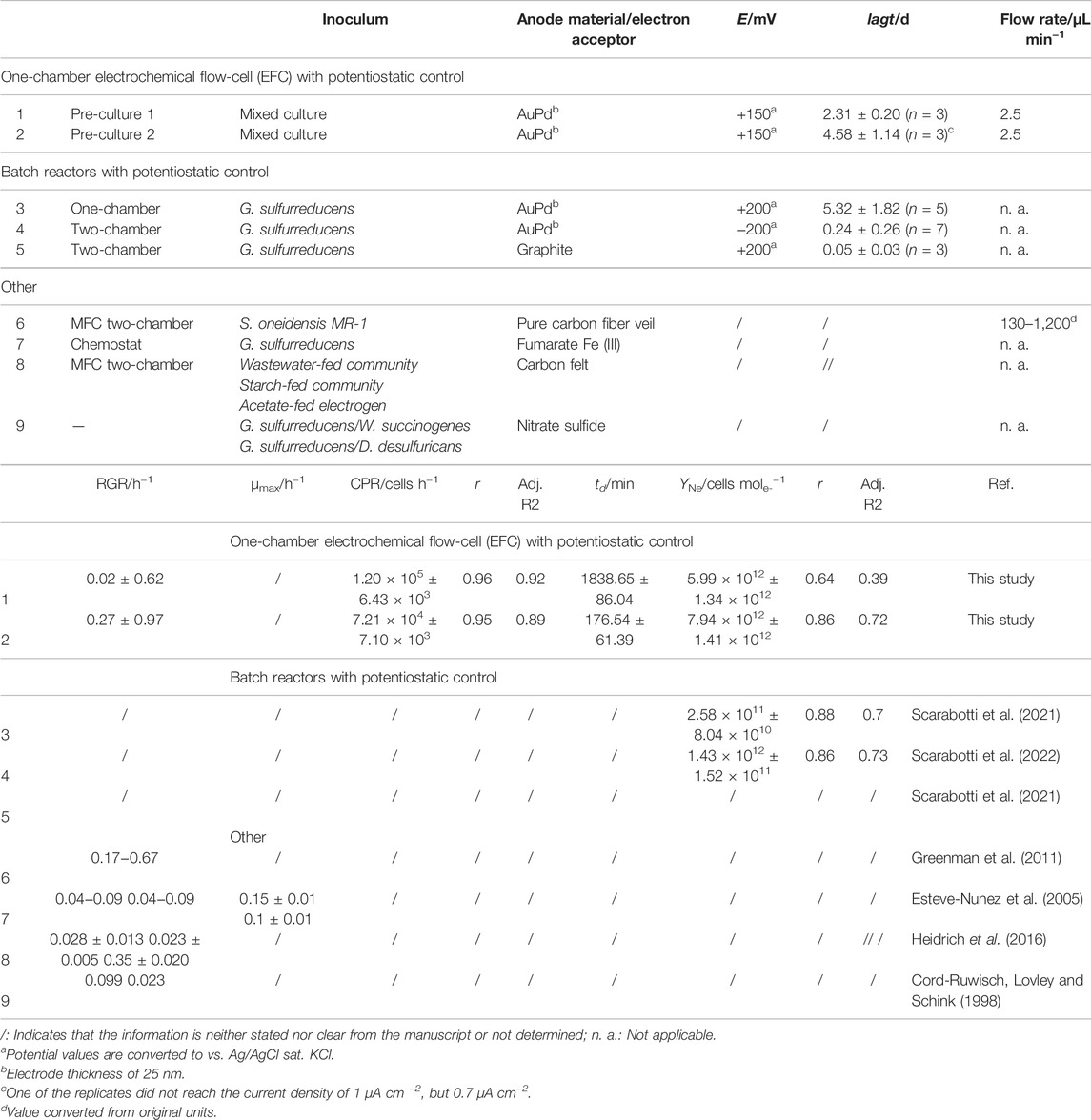
TABLE 1. Latency time (lagt), relative growth rates (RGR), cell production rate (CPR), doubling times (td), and yield coefficients (YNe) of early-stage mixed culture electroactive biofilms.
Non-invasive optical microscopy for determining Ncell of the AuPd WE (Figure 2A) showed an increase in the Ncell with the cultivation time for all CA experiments. To confirm microbial electrochemical activity, an abiotic control was performed showing no current production after 92 h (Figure 1B, blue line). For OCP controls, where the electric circuit was opened and thus the WE did not serve as TEA, a 24.4% increase was observed (Supplementary Figure S5). This was also the case in the presence of 50 mmol L−1 2-BES, being an inhibitor of archaea, where also no current was observed (Figure 1B). One may speculate that this non-electrochemical microbial growth decreases during electrochemical cultivation.
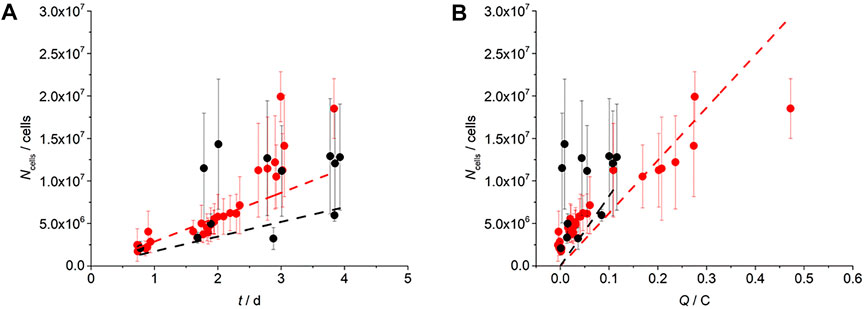
FIGURE 2. Cell number (Ncell) determined in vivo on a transparent AuPd WE during cultivation at +150 mV vs. Ag/AgCl sat. KCl as the function of time (A) and charge (B). Red and black data points indicate EFC experiments based on pre-cultures 1 and 2 (each n = 3), respectively. Dashed lines indicate linear regression used for the calculation of single-cell yield coefficients (YNe). Error bars indicate the standard error from cell counting. Each data point represents the average of 10 analyzed microscopy pictures (Supplementary Figure S3).
The high standard errors in the Ncell can be assigned to an inhomogeneous cell distribution over the AuPd WE during early-stage biofilm formation. As Supplementary Figure S6 shows, a higher cell density was observed closer to the inflow of the EFC. We hypothesize that this is mainly due to a voltage drop (i×R drop) over the length of the anode (Supplementary Figure S7). Figures 2A,B show the determination of the physiological parameters of the early-stage electroactive biofilms, with a CPR of 1.20 × 105 cells h−1 and 7.21 × 104 cells h−1, td of 1838.65 ± 86.04 min and 176.54 ± 61.39 min, and the RGR being 0.02 ± 0.62 h−1 and 0.27 ± 0.97 h−1, for the EFC based on pre-cultures 1 and 2, respectively (Table 1). Esteve-Nunez et al. (2005) showed that pure cultures of G. sulfurreducens grown on soluble TEA fumarate and Fe(III) exhibited an RGR of 0.04–0.09 h−1, with maximum growth rates of 0.15 ± 0.01 h−1 and 0.1 ± 0.01 h−1, respectively. Similarly, Heidrich et al. (2016) reported for wastewater, acetate, or starch-fed communities RGR of 0.028 ± 0.013 h−1, 0.023 ± 0.005 h−1, and 0.35 ± 0.020 h−1, respectively.
As expected, Figure 2B shows an increase in the cell number with charge (Molenaar et al., 2018; Scarabotti et al., 2021; Scarabotti et al., 2022). The
3.2 Microbial Community Composition
Sequencing of partial bacterial 16S rRNA genes and mcrA as the marker gene for methanogenic archaea was applied to analyse the microbial community composition of the pre-cultures and grown biofilms at the end of each experiment. As Figure 3 shows, both pre-cultures were mainly dominated by the genus Geobacter with relative abundances of 91.4 and 85.9%. Interestingly, in all EFC experiments, a community shift was observed. The relative abundances of Geobacter decreased toward the end of each experiment to 13.2 ± 3.5% for EFC experiments based on pre-culture 1 and 8.1 ± 10.3% for EFC experiments based on pre-culture 2. The high standard deviations, especially in the latter case, show that a stable establishment of Geobacter was not possible. The presence of other microorganisms, in particular Pseudomonadaceae, Xanthomonadaceae, and Rhodocyclaceae, strongly indicates that the EFC was not maintained under full anaerobic conditions. However, it is worth noticing that in OCP experiments performed in the EFC, Geobacter was almost absent in the biofilm samples and a high relative abundance of Pseudomonadaceae (72.7 ± 10.1%) and Xanthomonadaceae (22.4 ± 8.3%) was observed. These results confirm that the presence of an anode as the sole TEA is needed for the growth of electroactive biofilms and particularly Geobacteraceae.
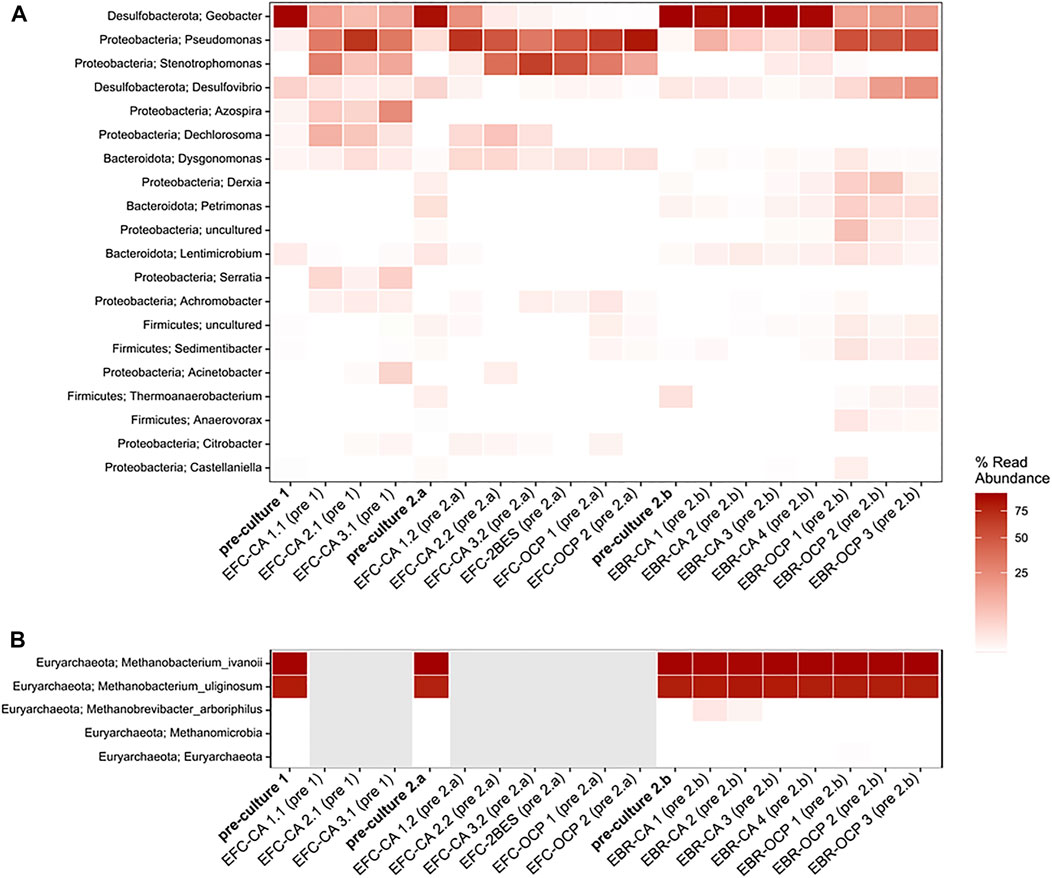
FIGURE 3. Heatmaps showing the relative community composition of bacteria (A) and methanogenic archaea (B) derived from the ILLUMINA sequencing analysis of bacterial 16S rRNA genes and archaeal mcrA gene (B). Gray boxes in (B) indicate samples where no methanogenic archaea were detected. Samples legend: pre-culture 1 and pre-culture 2 indicate the used pre-culture (or inocula); EFC: electrochemical flow-cell; CA: chronoamperometry; OCP: open circuit potential; EBR: electrochemical batch reactor (Section 2.1); pre-culture 2a and 2b indicate the respective inoculum that was used for CA and OCP experiments, respectively, and refer to pre-culture 2. Numbers indicate the replicate number.
Interestingly, Geobacter was only present in low proportions (0.2%) in the presence of 2-BES. As expected, in this case, no archaea were detected in the biofilm. Furthermore, neither current nor a significant Ncell increase was observed under these conditions (Figure 1B, Supplementary Figure S5). It was recently shown that the presence of archaea, and in particular, methanogens, in an electroactive biofilm inhibits Geobacter activity (Dzofou et al., 2021). These results are in contradiction with our observations, albeit being based on, for example, a different anode material. More importantly, we have to assume that our system was not anaerobic, as indicated by the presence of aerophilic microorganisms such as Pseudomonadaceae, which may explain these differences. Interestingly, for all the CA and OCP EFC experiments, no methanogenic archaea were detected in the biofilms, although they were present in the inoculum. This indicates that an establishment of methanogenic archaea in the EFC was not possible; especially as in OCP controls in the EBR, methanogenic archaea (in particular, representatives of the hydrogenotrophic Methanobacteriaceae) were detected. This observation emphasizes that in the EFC experiments, oxygen could permeate into the system. It should be noted that without microbial community analysis, this shortcoming certainly would have been overlooked and may have led to wrong conclusions. That it is a specific shortcoming of the EFC is also underlined by the CA control experiments in the EBR (Figure 3), where anaerobic conditions were maintained and the gained biofilms were dominated by Geobacter (up to 90.2 ± 3.1%). The electrochemical data have already indicated this, as in the biotic OCP EBR control experiments (Supplementary Figure S8, continuous red line), the potential of the AuPd WE stabilized at ∼−400 to 500 mV after ∼24–26 h of cultivation, whereas in the EFC experiments, the levels were ∼ +150 mV (Supplementary Figure S8, dotted und dashed red lines).
4 Conclusion
Coupling optical microscopy with chronoamperometric measurements in an EFC allows the correlation of the cell number with time and charge for early-stage electroactive biofilm anodes. This allows obtaining physiological information including replication rates, relative growth rates, and yield coefficients that are needed for process modeling, development, and especially scaling. The introduced EFC shows that the growth of EAM can be monitored in vivo in real-time and nondestructively, being a clear advantage when compared with other analyses (e.g., CLSM requiring staining and SEM) requiring sample preparation. The presented EFC is certainly a good starting point for this kind of analysis. Yet, the gathered data are only preliminary and have to be interpreted with outstanding care, and obtaining conclusive data requires significant improvements, especially to work in strictly anaerobic conditions, for example, by housing the entire system in an anaerobic tent. In this endeavor, numerous lessons can be learned from this study.
Data Availability Statement
Raw sequence data for this study was deposited at the European Nucleotide Archive (ENA) under the study accession number PRJEB52487 (http://www.ebi.ac.uk/ena/data/view/PRJEB52487).
Author Contributions
FS, FH, and KB contributed to the conception and design of the study. FS performed the experiments and wrote the first draft of the manuscript. AK and RK performed the ILLUMINA sequencing and sequencing analysis. All authors contributed to manuscript revision, and read and approved the submitted version.
Funding
This work was supported by the Helmholtz Association within the Research Programme Renewable Energies.
Conflict of Interest
The authors declare that the research was conducted in the absence of any commercial or financial relationships that could be construed as a potential conflict of interest.
Publisher’s Note
All claims expressed in this article are solely those of the authors and do not necessarily represent those of their affiliated organizations, or those of the publisher, the editors, and the reviewers. Any product that may be evaluated in this article, or claim that may be made by its manufacturer, is not guaranteed or endorsed by the publisher.
Acknowledgments
We thank Christin Koch for the highly valuable pre-work and discussion. The authors are grateful for the use of the sputter-coater facility at ProVIS-Centre for Chemical Microscopy by the Helmholtz-Centre for Environmental Research, Leipzig, which is supported by the European Regional Development Fund (ERDF, operational programme for Saxony) and the Helmholtz Association.
Supplementary Material
The Supplementary Material for this article can be found online at: https://www.frontiersin.org/articles/10.3389/fenrg.2022.920266/full#supplementary-material
References
Baron, D., LaBelle, E., Coursolle, D., Gralnick, J. A., and Bond, D. R. (2009). Electrochemical Measurement of Electron Transfer Kinetics by Shewanella Oneidensis MR-1. J. Biol. Chem. 284 (42), 28865–28873. doi:10.1074/jbc.M109.043455
Brown, D. G., Komlos, J., and Jaffé, P. R. (2005). Simultaneous Utilization of Acetate and Hydrogen by Geobacter Sulfurreducens and Implications for Use of Hydrogen as an Indicator of Redox Conditions. Environ. Sci. Technol. 39 (9), 3069–3076. doi:10.1021/es048613p
Cord-Ruwisch, R., Lovley, D. R., and Schink, B. (1998). Growth of Geobacter Sulfurreducens with Acetate in Syntrophic Cooperation with Hydrogen-Oxidizing Anaerobic Partners. Appl. Environ. Microbiol. 64 (6), 2232–2236. doi:10.1128/AEM.64.6.2232-2236.1998
Dzofou Ngoumelah, D., Harnisch, F., and Kretzschmar, J. (2021). Benefits of Age-Improved Resistance of Mature Electroactive Biofilm Anodes in Anaerobic Digestion. Environ. Sci. Technol. 55 (12), 8258–8266. doi:10.1021/acs.est.0c07320
Esteve-Nunez, A., Rothermich, M., Sharma, M., and Lovley, D. (2005). Growth of Geobacter Sulfurreducens under Nutrient-Limiting Conditions in Continuous Culture. Environ. Microbiol. 7 (5), 641–648. doi:10.1111/j.1462-2920.2005.00731.x
Gimkiewicz, C., and Harnisch, F. (2013). Waste Water Derived Electroactive Microbial Biofilms: Growth, Maintenance, and Basic Characterization. JoVE (82), e50800. doi:10.3791/50800
Greenman, J., Pablo, L., Pereira-Medrano, A. G., Wright, P. C., and Ieropoulos, I. (2011). “The Importance of Specific Growth Rate of Biofilms for Electricity Production in Microbial Fuel Cell Systems,” in Proceedings of the IWA Biofilm conference, Shanghai, China, October 2011.
Heidrich, E. S., Curtis, T. P., Woodcock, S., and Dolfing, J. (2016). Quantification of Effective Exoelectrogens by Most Probable Number (MPN) in a Microbial Fuel Cell. Bioresour. Technol. 218, 27–30. doi:10.1016/j.biortech.2016.06.066
Ikeda, S., Takamatsu, Y., Tsuchiya, M., Suga, K., Tanaka, Y., Kouzuma, A., et al. (2021). Shewanella Oneidensis MR-1 as a Bacterial Platform for Electro-Biotechnology. Essays Biochem. 65 (2), 355–364. doi:10.1042/EBC20200178
Koch, C., and Harnisch, F. (2016). What Is the Essence of Microbial Electroactivity? Front. Microbiol. 7 (NOV), 1890. doi:10.3389/fmicb.2016.01890
Korth, B., Kretzschmar, J., Bartz, M., Kuchenbuch, A., and Harnisch, F. (2020a). Determining Incremental Coulombic Efficiency and Physiological Parameters of Early Stage Geobacter Spp. Enrichment Biofilms. PLOS ONE 15 (6), e0234077. doi:10.1371/journal.pone.0234077
Korth, B., Kuchenbuch, A., and Harnisch, F. (2020b). Availability of Hydrogen Shapes the Microbial Abundance in Biofilm Anodes Based on Geobacter Enrichment. ChemElectroChem 7 (18), 3720–3724. doi:10.1002/celc.202000731
Kosse, P., Lübken, M., and Wichern, M. (2016). Selective Inhibition of Methanogenic Archaea in Leach Bed Systems by Sodium 2-bromoethanesulfonate. Environ. Technol. Innovation 5, 199–207. doi:10.1016/j.eti.2016.03.003
Logan, B. E., Rossi, R., Ragab, A. a., and Saikaly, P. E. (2019). Electroactive Microorganisms in Bioelectrochemical Systems. Nat. Rev. Microbiol. 17 (5), 307–319. doi:10.1038/s41579-019-0173-x
Lovley, D. R. (2012). Electromicrobiology. Annu. Rev. Microbiol. 66 (1), 391–409. doi:10.1146/annurev-micro-092611-150104
Lovley, D. R., and Holmes, D. E. (2021). Electromicrobiology: the Ecophysiology of Phylogenetically Diverse Electroactive Microorganisms. Nat. Rev. Microbiol. 20, 5–19. doi:10.1038/s41579-021-00597-6
Lovley, D. R., and Walker, D. J. F. (2019). Geobacter Protein Nanowires. Front. Microbiol. 10. doi:10.3389/fmicb.2019.02078
Molenaar, S. D., Sleutels, T., Pereira, J., Iorio, M., Borsje, C., Zamudio, J. A., et al. (2018). In Situ Biofilm Quantification in Bioelectrochemical Systems by Using Optical Coherence Tomography. ChemSusChem 11 (13), 2171–2178. doi:10.1002/cssc.201800589
Polk, B. J., Stelzenmuller, A., Mijares, G., MacCrehan, W., and Gaitan, M. (2006). Ag/AgCl Microelectrodes with Improved Stability for Microfluidics. Sensors Actuators B Chem. 114 (1), 239–247. doi:10.1016/j.snb.2005.03.121
Scarabotti, F., Bühler, K., Schmidt, M., and Harnisch, F. (2022). Thickness and Roughness of Transparent Gold-Palladium Anodes Have No Impact on Growth Kinetics and Yield Coefficients of Early-Stage Geobacter Sulfurreducens Biofilms. Bioelectrochemistry 144, 108043. doi:10.1016/j.bioelechem.2021.108043
Scarabotti, F., Rago, L., Bühler, K., and Harnisch, F. (2021). The Electrode Potential Determines the Yield Coefficients of Early-Stage Geobacter Sulfurreducens Biofilm Anodes. Bioelectrochemistry 140, 107752. doi:10.1016/j.bioelechem.2021.107752
Schröder, U. (2011). Discover the Possibilities: Microbial Bioelectrochemical Systems and the Revival of a 100-Year-Old Discovery. J. Solid State Electrochem 15, 1481–1486. doi:10.1007/s10008-011-1395-7
Wang, F., Gu, Y., O’Brien, J. P., Yi, S. M., Yalcin, S. E., Srikanth, V., et al. (2019). Structure of Microbial Nanowires Reveals Stacked Hemes that Transport Electrons over Micrometers. Cell. 177 (2), 361–369. e10. doi:10.1016/j.cell.2019.03.029
Webster, T. M., Smith, A. L., Reddy, R. R., Pinto, A. J., Hayes, K. F., and Raskin, L. (2016). Anaerobic Microbial Community Response to Methanogenic Inhibitors 2‐bromoethanesulfonate and Propynoic Acid. MicrobiologyOpen 5 (4), 537–550. doi:10.1002/mbo3.349
Keywords: electroactive microorganisms, relative growth rates, duplication rate, Geobacter, flow-cell
Citation: Scarabotti F, Kuchenbuch A, Kallies R, Bühler K and Harnisch F (2022) Toward Real-Time Determination of Yield Coefficients of Early-Stage Electroactive Biofilms by Optical Microscopy. Front. Energy Res. 10:920266. doi: 10.3389/fenrg.2022.920266
Received: 14 April 2022; Accepted: 24 May 2022;
Published: 05 July 2022.
Edited by:
Pierangela Cristiani, Ricerca Sul Sistema Energetico, ItalyReviewed by:
Xianhua Liu, Tianjin University, ChinaOlja Simoska, The University of Utah, United States
Copyright © 2022 Scarabotti, Kuchenbuch, Kallies, Bühler and Harnisch. This is an open-access article distributed under the terms of the Creative Commons Attribution License (CC BY). The use, distribution or reproduction in other forums is permitted, provided the original author(s) and the copyright owner(s) are credited and that the original publication in this journal is cited, in accordance with accepted academic practice. No use, distribution or reproduction is permitted which does not comply with these terms.
*Correspondence: Falk Harnisch, ZmFsay5oYXJuaXNjaEB1ZnouZGU=