- 1USDA–ARS, Agroecosystem Management Research Unit, University of Nebraska-Lincoln East Campus, Lincoln, NE, United States
- 2USDA–ARS, Wheat, Sorghum and Forage Research Unit, University of Nebraska-Lincoln East Campus, Lincoln, NE, United States
- 3USDA–ARS, Soil Management and Sugarbeet Research, Fort Collins, CO, United States
- 4Department of Agricultural Economics, Institute of Agriculture and Natural Resources, University of Nebraska-Lincoln East Campus, Lincoln, NE, United States
- 5Department of Agronomy and Horticulture, University of Nebraska-Lincoln East Campus, Lincoln, NE, United States
- 6Argonne National Laboratory, Lemont, IL, United States
Managing annual row crops on marginally productive croplands can be environmentally unsustainable and result in variable economic returns. Incorporating perennial bioenergy feedstocks into marginally productive cropland can engender ecosystem services and enhance climate resiliency while also diversifying farm incomes. We use one of the oldest bioenergy-specific field experiments in North America to evaluate economically and environmentally sustainable management practices for growing perennial grasses on marginal cropland. This long-term field trial called 9804 was established in 1998 in eastern Nebraska and compared the productivity and sustainability of corn (Zea mays L.)—both corn grain and corn stover—and switchgrass (Panicum virgatum L.) bioenergy systems under different harvest strategies and nitrogen (N) fertilizer rates. This experiment demonstrated that switchgrass, compared to corn, is a reliable and sustainable bioenergy feedstock. This experiment has been a catalyst for other bioenergy projects which have also expanded our understanding of growing and managing bioenergy feedstocks on marginal cropland. We (1) synthesize research from this long-term experiment and (2) provide perspective concerning both the knowledge gained from this experiment and knowledge gaps and how to fill them as well as the role switchgrass will play in the future of bioenergy.
Introduction
The United Nations Sustainable Development Goal 7 (SDG 7) aims to ensure access to affordable, reliable, sustainable, and modern energy for all. The energy goals of the United States align with SDG 7, evident in Executive Order (EO) 14081 issued in September 2022. The official title of EO 14081 is Advancing Biotechnology and Biomanufacturing Innovation for a Sustainable, Safe, and Secure American Bioeconomy. Executive order 14081 aims to accomplish what is stated in its title by enhancing the collection of data associated with the economic value of the U.S. bioeconomy. Bioenergy from biofuels can help achieve international and national goals stated in SDG 7 and EO 14081. Bioenergy from biofuels can also play a role in meeting low carbon fuel standards. The main goal of low carbon fuel standards is to reduce the well-to-wheel carbon content of transportation fuels. The cultivation of biofuel feedstocks may offer opportunities to sequester C—as well as the provision of other important ecosystem services—compared to other fuels such as fossil fuels. The C benefits of biofuels will depend on landscape position, agricultural management, and feedstock selection. Near-term barriers exist for growing perennial bioenergy crops, however, that limit wide-scale adoption of liquid biofuels and bio-based products. Those near-term barriers include demand, market uncertainty, producer adoption and conversion technology at scale. Overcoming these near-term barriers will require concerted efforts from various stakeholders including farmers, policymakers, researchers, industry, and local communities.
Gopalakrishnan et al. (2011) estimated that 1.6 million ha in Nebraska could be classified as marginal cropland using a framework that incorporates several indicators of profitability and soil health. Economically marginal land, however, may not fit the definition of marginal land in a biophysical sense (Csikós and Tóth, 2023). While inputs and land management practices can transform nonproductive land into productive land, the economic feasibility of such a transformation may not be justified. However marginally productive croplands are defined, crop production on marginally productive cropland is typically linked to increased natural resource degradation (Brandes et al., 2018). Perennializing marginally productive croplands already under annual row crop production would diversify agricultural landscapes and farm incomes while protecting natural resources and providing a reliable feedstock for bioenergy production (Asbjornsen et al., 2014). Perennial feedstock production on marginally productive land is likely to result in improved ecosystem services, including reduced soil erosion losses, improved water quality, pest suppression, decreased greenhouse gas emissions, increased SOC storage, and improved wildlife and pollinator habitats (Mitchell et al., 2008; Fargione et al., 2009; Valentine et al., 2012; Werling et al., 2014).
The transition from horsepower to tractor power in the early 20th century in the United States released 32 million hectares of pastureland and hayland—previously used to feed horses—to be used mostly for annual row crop production despite being marginal for row crop production (Vogel, 1996). Perennializing marginal lands currently under row crop production—which were historically used for forage—with perennial bioenergy crops is going back to the future (Vogel, 1996). In that vein, the purpose of this article is to look forward by taking stock of the past—to consider the future of bioenergy derived from perennial grasses by briefly summarizing what has been reported in an on-going study which is believed to be one of the oldest bioenergy-specific experiments in North America. That study, now known as 9804, was established in 1998 by USDA-ARS researchers in Lincoln, NE and Ft. Collins, CO. This study was conducted on marginal cropland. In 9804, marginal land was defined as cropland with crop yields 25% below the regional average (Mitchell et al., 2012; Schmer et al., 2014). While the original objective of comparing soil C and N differences under switchgrass and corn has been researched (Follett et al., 2012) and is still on-going, the study site has allowed for further research related to crop productivity (Varvel et al., 2008; Schmer et al., 2010), soil property changes (Jin et al., 2015; Stewart et al., 2015; Stewart et al., 2016), cell wall composition (Vogel et al., 2011), and greenhouse gas (GHG) emissions (Schmer et al., 2014; Schmer et al., 2015; Jin et al., 2019) among others. Data generated from this study and related studies (Liebig et al., 2008; Perrin et al., 2008; Schmer et al., 2008; Mitchell et al., 2010; Ramirez et al., 2023) has led to greater knowledge on designing economical, reliable, and sustainable bioenergy systems from perennial feedstocks in temperate climates. A brief synthesis of the knowledge gained from 9804, remaining knowledge gaps, and plans to fill those knowledge gaps are below.
Study description
The 9804 study was initiated to determine the reliablity and sustainabilty of biofuel feedstocks (switchgrass, corn grain, and corn stover) and to determine the agricultural management which maximized yields and protected environemental resources. The 9804 experiment is located at the University of Nebraska Eastern Nebraska Research, Extension and Education Center near Mead, Nebraska (N 41.151 W 96.401). Soils at the site consist of Yutan silty clay loams (a fine-silty, mixed, superactive, mesic Mollic Hapludalf), Tomek silt loams (a fine, smectitic, mesic Pachic Argiudoll), and Filbert silt loams (a fine, smectitic, mesic Vertic Argialboll), comprising 47, 35, and 18% of the study area, respectively (Figure 1B). The study was established in a randomized complete block split-split plot design (n = 3), where main plot sizes were 0.3 ha to accommodate commercial equipment. This experiment compared bioenergy crop species (corn and switchgrass) under different N fertilzation rates and different harvest practices. The main plot treatments consist of two cropping systems, no-till corn and switchgrass. Initially, there were two cultivars of switchgrass, Cave-in-Rock and Trailblazer. After 11 years, however, the Trailblazer main plots were converted to the “Liberty” cultivar which was developed specifically for use in biomass production systems. Subplot treatments, consisting of N fertilization (0, 60, and 120 kg N ha−1 year−1), were established during the onset of this study in 1998. A sub-sub plot treatment, initiated in 2001, consisted of harvest practices specfic to cropping sytems. The harvest treatment in no-till, continous corn consists of either no residue removal or 50% residue removal. In switchgrass, the harvested treatment consists of an August or postfrost harvest. Corn stover removal treatments began in 2000. A 0 kg N ha−1 year−1 treatment in corn began in 2010 as a nested treatment within the 60 kg N ha−1 year−1 subplot to quantify background GHG emissions. It was assummed that new switchgrass cultivars would be available about every 10 years so a rotational switchgrass treatment was implemented by killing the Trailblazer plots with herbicides and growing no-till soybeans for 2 years and then seeding the new switchgrass cultivar. Liberty cultivar continues at present in the former Trailblazer plots under the same harvest and N management treatments (e.g., rotational switchgrass), but this treatment is not discussed in detail in this paper.
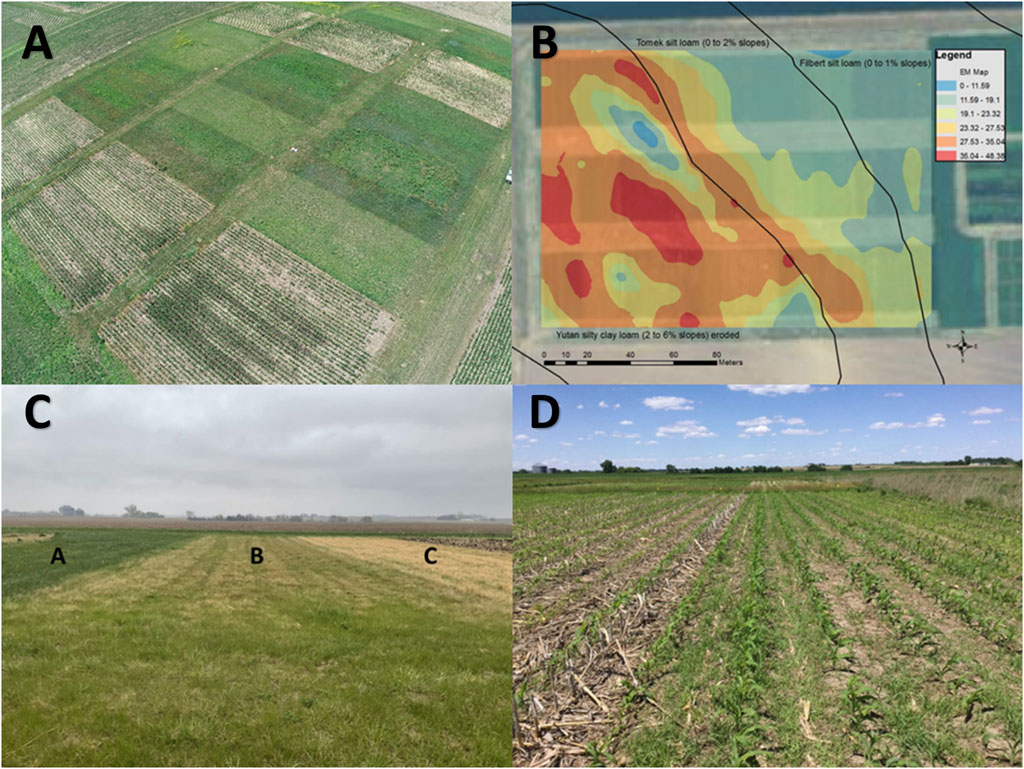
FIGURE 1. (A) Aerial image of a long-term corn-switchgrass study in the western Corn Belt Region USA Agricultural Research Service scientists in Lincoln, NE originally coded this experiment as “9804–2116” but is now known simply as “9804” (B) Overlay of soil apparent electrical conduction measured by electromagnetic induction on a soil survey map of the site. Considerable site variation exists on the western portion of the site, a result of buried sand deposits from a former river channel. (C) Harvest timing and N management influences C3 grass encroachment in switchgrass stands. Plot A was established in 1998 using cultivar “Cave-in-Rock” that has been fertilized at 120 kg N ha−1 and annually harvested in August. Plot A has largely been invaded by smooth bromegrass (Bromus inermis Leyss.) and Kentucky bluegrass (Poa pratensis L.). Plot B was originally in Trailblazer then converted to Liberty and fertilized at 0 kg N ha−1 and harvested in August whereas Plot C was originally in Trailblazer then converted to Liberty and fertilized at 0 kg N ha−1 and harvested after a killing frost. Plot C has minimal C3 grass encroachment from the later harvest period. (D) Historical corn stover removal (center right) can lead to increased annual weeds during late spring compared to stover being retained in the system (left).
Switchgrass is a reliable and sustainable bioenergy feedstock
The purpose of this article is to look toward developing sustainable bioenergy systems by taking stock of the past (i.e., results from 9804). While there has been a wide range of research topics investigated in 9804 (Supplementary Table S1), the focus of this paper will be to summarize the (1) aboveground and belowground productivity and (2) climate change mitigation potential (i.e., SOC sequestration and GHG emissions) of corn and switchgrass. Additionally, corn stover was a bioenergy feedstock of interest in the early 2000s. A key research objective concerning corn stover was to determine how much—if any—could be sustainably harvested as a bioenergy feedstock while maintaining SOC levels and avoiding soil erosion. This study (9804) was part of an ARS-REAP subset of studies used to evaluate the sustainability of corn stover harvest (Karlen, 2010) and was one of the first to document near-term differences in SOC accrual rates in corn systems (Follett et al., 2012) due to harvesting stover (Varvel et al., 2008). Thus, special attention will be paid to the effect of stover removal on crop productivity and climate change mitigation. As such, a synthesis of crop productivity, C sequestration, GHG emissions, and plant rooting dynamics in 9804 are briefly summarized below. We focus our review on the subset of commonly managed treatments; corn—with and without 50% corn stover harvest—under 120 kg N ha−1 and switchgrass harvested post frost under 60 and 120 kg N ha−1.
Management influences the long-term productivity and sustainability of bioenergy feedstocks
The 9804 study has demonstrated that (1) optimally manged switchgrass can maintain productive biomass yields similar to corn grain and or corn grain + harvested stover, and (2) management determines the productivity and sustainability of switchgrass and corn bioenergy systems on marginally productive lands. Harvesting switchgrass post-killing frost compared with an August harvest resulted in greater biomass yields (Follett et al., 2012) and increased aboveground biomass C while decreasing aboveground biomass N (Stewart et al., 2016). Harvesting post-killing frost results in nutrient translocation from aboveground to belowground biomass, as well as an increase in the C:N ratio of aboveground biomass (Wayman et al., 2014; Stewart et al., 2016). As such, the N dynamics discussed below are for switchgrass harvested post killing frost. Increasing N fertilization increased aboveground biomass (3.13, 8.27, and 11.20 Mg ha−1 under 0, 60, and 120 kg N ha−1) and influenced C allocation (Stewart et al., 2016). Although N fertilization also increased aboveground biomass C, 60 kg N ha−1 optimized belowground biomass C. Furthermore, increasing N fertilization decreased switchgrass root C:N ratio. In summary, 120 kg N ha−1 maximized aboveground biomass, but 60 kg N ha−1 maximized belowground biomass and incorporated more belowground root biomass C into the soil C pool.
Optimally managed switchgrass—switchgrass under N fertilization harvested post killing frost—yields equated to ethanol potentials similar to those from a corn with stover removal (also used for bioenergy production) and exceeded ethanol potentials for corn grain-only systems (Schmer et al., 2014; Jin et al., 2019). Fertilized switchgrass was as net energy efficient as low-input switchgrass systems but produced significantly greater quantities of energy per unit of land (Schmer et al., 2014). Conversely, less than optimally managed switchgrass—under no N fertilization and harvested in August—resulted in plant encroachment. Harvest timing and N management influences C3 grass encroachment in switchgrass stands (Figure 1C). Results indicate that switchgrass is more likely to be invaded by C3 grasses and native C4 grasses especially in low-input systems (Mitchell and Vogel, 2016). Similarly, less than optimally managed corn systems, or those with long term corn stover removal, can result in altered weed dynamics compared to those with stover retention (Figure 1D).
Mitigation benefits: C sequestration and GHG emissions
The GHG balance of 9804 was driven primarily by changes in SOC and soil N2O emissions associated with N fertilzaiton. Soil methane (CH4) emissions were not affected by year, crop, or N rate in 9804 and were negligible compared to N2O emissions (Jin et al., 2019), so the GHG emissions discussion below will focus on N2O fluxes. Similarly, it is important to note that while the following discussion of SOC and N2O dynamics are based on soils from 0 to 30 cm (Jin et al., 2019), Follett et al. (2012) sampled surface and subsurface soils (0–120 cm) in 9804 and found that, after 9 years (1998–2007), over half of the SOC stored under both switchgrass and corn occurred below the 30 cm depth. While there were no significant differences in the rate of SOC accrual under bioenergy switchgrass and corn after 9 years (1998–2007) or 16 years (1998–2014) in the surface soils (0–30 cm) of 9804, the rate of accrual under switchgrass, albeit non-significant, was greater after 16 years. After 16 years, corn under 120 kg N ha−1 maintained SOC when corn residue was harvested (0.5 ± 0.3 Mg C ha−1 year−1) and gained SOC when no corn residue was harvested (0.7 ± 0.4 Mg C ha−1 year−1) (Jin et al., 2019). Although non-significant, accrual rates under switchgrass harvested post killing frost were greater (1.1 ± 0.1 and 1.1 ± 0.3 Mg C ha−1 year−1 under 60 kg N ha-1 and 120 kg N ha−1, respectively) than accrual rates under corn (Jin et al., 2019). Because corn and switchgrass systems either maintained or gained SOC after 16 years, GHG balances were driven by the magnitude of direct N2O emissions. N2O emissions from switchgrass under 60 kg N ha−1 year−1 did not differ from 0. In contrast, both switchgrass and corn—with and without residue removal—under 120 kg N ha−1 year−1 released N2O (Jin et al., 2019). In summary, (Jin et al., 2019), aggregated the changes in SOC (1998–2014) and N2O emissions (2011–2017) along with emisisons from fuel use and agrochemical manufaction in order to determine the production phase net GHG emissions of bionergy switchgrass and corn, concluding that net agricultural emissions from no-till corn systems were GHG neutral (i.e., not significantly different from zero; 0.3–1.6 Mg CO2 eq ha−1 year1) while switchgrass was as GHG sink (−3.1 to 1.1 Mg CO2 eq ha−1 year−1) (Jin et al., 2019). Changes in SOC accounted for a greater portion of gross emissions when comparing switchgrass to corn (66%–70% and 35%, respectively), while direct N2O emissions accounted for a greater portion of gross emissions under corn compared to switchgrass (40% and 22%–23%, respectively) (Jin et al., 2019).
The effect of N fertilization on switchgrass rooting dynamics
Switchgrass rooting dynamics were influenced by N fertilization. The 60 kg N ha−1 compared to the 120 kg N ha−1 in switchgrass optimized both belowground biomass (26.92 and 20.17 Mg ha−1, respectively) and belowground biomass C (8.66 and 5.43 Mg C ha−1, respectively) when taking a mean across harvest methods in the 0–150 cm soil sampling depth after 9 years (Stewart et al., 2016). These differing belowground dynamics, however, did not lead greater rates of SOC accrual under 60 kg N ha−1 after 16 years. Greater, albeit non-significant, rates of SOC accrual were reported under 60 compared to 120 kg N ha−1 in the 0–30, 0–120, and 0–150 cm cumulative soil layers after 9 years (Follett et al., 2012), but similar accrual rates were reported after 16 years (1.1 ± 0.1 and 1.1 ± 0.3 Mg C ha−1 under 60 kg N ha−1, and 120 kg N ha−1, respectively) (Jin et al., 2019). This lack of preferential accrual when comparing the 2 N fertilization rates even though switchgrass belowground biomass and belowground biomass C is maximized under 60 kg N ha−1 could be due to differences in root biomass C:N ratios (Stewart et al., 2016).
Corn stover harvest effects on soil properties
Research conducted at 9804 found that harvesting stover in NT, continuous corn systems can trigger important near-term tradeoffs in surface and near-surface soils. Although corn under 120 kg N ha−1 accrued SOC under both stover harvest treatments after 9 years in surface soils (0–30 cm), ΔSOC was significantly smaller when stover was harvested (Follett et al., 2012). This trend of limited near-term SOC gains under stover removal persisted even after 12 years (Jin et al., 2015), but not after 16 years (0.5 ± 0.3 and 0.7 ± 0.4 under residue removal and no residue removal, respectively) (Jin et al., 2019). Reside removal also reduced aboveground C inputs and decreased root contributions to SOC (Stewart et al., 2016). In addition to limiting near-term SOC gains, harvesting stover increased soil erosion potential (Osborne et al., 2014; Jin et al., 2015), decreasing aggregate size and stability (Stewart et al., 2015). Harvesting stover for 12 years decreased the largest size class of dry aggregates (>2.0 mm) in all soil surface depths (at 0–5 cm, from 55% to 40%; at 5–10 cm, from 72% to 60%; at 10–30 cm, from 72% to 68%) with concomitant increases in all smaller size fractions except for the small size class (<0.053 mm) (Jin et al., 2015). The impact of stover harvest on the distribution of dry aggregates led to increased erodible fractions (Osborne et al., 2014). Given how important aggregate formation is to the stabilization of SOM (Six et al., 1998), both the decrease in ΔSOC and the changes in soil physical properties should be considered with determining the amount of stover to be harvested.
Perspective
Lessons learned on perennializing marginal cropland
The SOC dynamics in 9804 highlight the importance of long-term studies with sub-surface soil samples. Treatment driven ΔSOC are best detected in long-term studies (Follett et al., 2012; Stewart et al., 2015; Jin et al., 2019; Stewart et al., 2022) and studies sampling subsurface soils (Follett et al., 2012). While SOC storage increased under both corn and switchgrass, SOC storage was non-significantly greater under switchgrass after 16 years. This non-signifcant differences could be due to differnces in plant root architecture, depth, and turnover (De Deyn et al., 2008; Fissore et al., 2008; Stewart et al., 2016). Corn and switchgrass differ in their spatiotemporal C additions primarily due to their rooting systems and C allocation strategies. Switchgrass, compared to corn, (1) has a deeper rooting system (2.7–3.3 and 1.2 m, respectively) (Weaver and Darland, 1949; Rosa et al., 2019), (2) allocates more C to root biomass, evident in differing root-to-shoot ratios of C (1.5–6.1 for switchgrass and 0.33–0.55 for fertilized corn) (Ma et al., 2001; Bonifas et al., 2005; Johnson et al., 2006), and (3) can have greater belowground biomass, belowground root biomass C and N, and a higher belowground root biomass C:N ratio (Stewart et al., 2016). Given that (1) the net balance of GHGs depends on SOC storage and N2O emissions and (2) ΔSOC were similar in switchgrass under 60 kg N ha−1 and 120 kg N ha−1, differences in N2O emissions dictated the GHG potential difference of switchgrass under 60 kg N ha−1and 120 kg N ha−1. After 16 years, switchgrass under 60 kg N ha−1 was a GHG sink. Switchgrass under 120 kg N ha−1, as well as continuous corn with or without residue removal, were net GHG neutral. Lastly, this study was one of the first to document near-term differences in SOC accrual rates due to harvesting stover. As part of multisite study, the stover harvest outcomes of this study supported the conclusion that harvesting stover on marginal land could trigger important tradeoffs (Johnson et al., 2014).
Knowledge gaps on perennializing marginal cropland
While C storage and GHG emissions are important indicators of sustainability, other indicators such as biodiversity need additional investigation. The expansion of annual row crops onto marginally productive lands in the U.S. Midwest has decreased insect, avian, and wildlife biodiversity via habitat loss (Lark et al., 2020) triggering important ecosystem services tradeoffs. For example, abundant and diverse arthropod communities serve as an important source of food for other wildlife (Capinera, 2010) and as natural enemies of crop pests (Begg et al., 2017), and play a key role in ecosystem level decomposition and nutrient cycling (Nichols et al., 2008). Other ecosystem services engendered by biodiversity, such as avian biodiversity, include human wellbeing via recreation and hunting (Whelan et al., 2008). Increased corn acreage can decrease insect biodiversity and subsequently the biological control of crop pests (Landis et al., 2008). Diversifying agricultural landscapes by including perennial warm-season grasses can enhance insect, avian, and wildlife biodiversity and their concomitant ecosystem services. For example, Meehan et al. (2010) forecasted that replacing annual crops with diverse, perennial bioenergy crops would increases avian richness between 12% and 207% across 20% of the Midwestern region. Werling et al. (2014) compared biodiversity in a combination of corn, switchgrass, and prairie plantings across Michigan and Wisconsin. They found that herbivorous and predatory arthropods, bee species, and breeding bird species were greater in switchgrass and prairie compared to corn. However, a key knowledge gap remains: how can these ecosystem service provisioned by perennializing bioenergy feedstocks—in this case, enhanced biodiversity—be economically valuated and considered when adopting perennial bioenergy crops on marginally productive cropland?
Another indicator of sustainability that needs to be further explored in bioenergy systems is the impact of bioenergy corn and switchgrass crop water use on groundwater resources. Irrigated agriculture in the US Great Plains depends on the High Plains-Ogallala Aquifer, which is the largest source of groundwater in the US and lies underneath eight states in the US Great Plains region (Smidt et al., 2016). Groundwater levels across large areas of the High Plains-Ogallala Aquifer have been decreasing for decades due to agricultural irrigation (Haacker et al., 2016). Global climate models project warmer summers with less precipitation in this region which is expected to exacerbate the depletion of the High Plains-Ogallala Aquifer (Anandhi et al., 2023). Hoover et al. (2023) compared the WUE of corn and switchgrass in 9804 using aboveground biomass and grain yield as productivity indicators and growing season precipitation as the water use indictor and found that switchgrass (17.0 and 23.6 under 60 and 120 kg N ha−1, respectively) had a higher WUE than corn grain (10.7 and 13.1 under 60 and 120 kg N ha−1, respectively). Given the potential differences in crop water use between bioenergy corn and switchgrass, and given the concerns surrounding the High Plains-Ogallala Aquifer depletion, more research is needed to determine how perennializing bioenergy feedstocks would impact, specifically, the Ogallala Aquifer.
Lastly, another under explored area concerning, specifically, bioenergy switchgrass is farm revenue and economic risk. A basic economic comparison of perennial grasses and corn on marginal land was conducted using a crop enterprise budget reflective of the central Plains (Klein and McClure, 2023). These crop budgets were modified to match management practices and long-term yields from 9804. Land costs were not considered in this analysis. Crop prices of $0.20 and $0.11 kg–1 were assumed for corn and switchgrass, respectively, as they reflect crop price in the United States. The price of switchgrass was assumed to be similar to the price of hay. Mean (2000–2018) corn (under no residue removal) and switchgrass (harvested post killing frost) yields from 9804 were used in this analysis. Net return above total costs were 385.44 and 155.82 per $ ha–1 for corn under 120 and 180 kg N ha–1, respectively, and 109.52, 530.36 and 815.22 $ ha–1 for switchgrass under 0, 60, and 120 kg N ha–1, respectively (Table 1). Economic results indicate that perennial grass returns can exceed that of corn when optimally managed on marginal cropland, but more extensive economic analyses are needed.
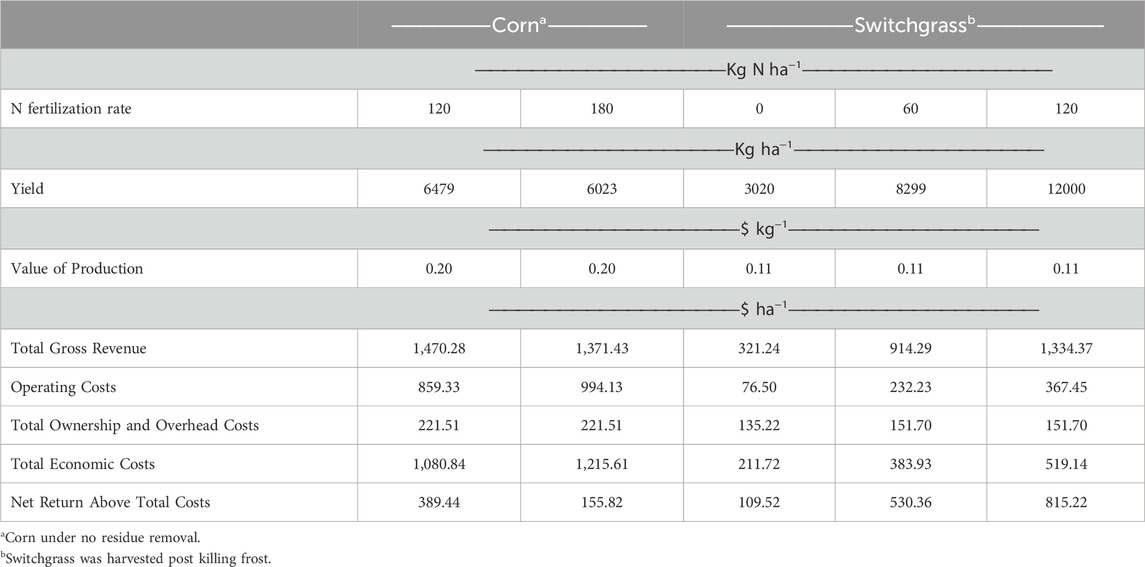
TABLE 1. Long-term economic overview of corn and switchgrass systems under different levels of N fertilization from 9804.
Research inspired by 9804
The 9804 study has inspired other perennial grass bioenergy projects in the central United States. These include CenUSA as well as on-farm, regional switchgrass trials (Liebig et al., 2008; Perrin et al., 2008; Schmer et al., 2008). CenUSA was a USDA National Institute of Food and Agriculture (NIFA) funded coordinated agricultural project. The goal of CenUSA was to investigate the creation of Midwestern sustainable biofuels and bioproducts systems (Moore et al., 2014; Porter et al., 2015). Another research trial inspired by 9804 is the Expanding the Conversion of HAbitat in the Northern Great Plains Ecosystem (EXCHANGE) project. The goal of EXCHANGE is to fill previously discussed knowledge gaps by characterizing the monetary value of the ecosystem services provisioned by incorporating perennial bioenergy crops into primary irrigated landscapes. Small-plot and on-farm experiments have been established west of the 100 Meridian in Nebraska, United States. The ecosystems services that are characterized in EXCHANGE include potential changes in SOC and GHG emissions, avian and arthropod biodiversity, and modeled impacts to the High Plains Ogallala Aquifer. A technoeconomic analysis will be conducted to quantify the monetary value of ecosystem services. Characterizing the monetary value of ecosystem services provisioned by perennializing bioenergy feedstock will identify potential incentives and obstacles for the adoption of perennial bioenergy feedstocks.
Continued research from 9804 and related studies will aid in the design of diverse, multifunctional, climate resilient agricultural landscapes. Rather than assigning land the dichotomous roles of either food or fuel production (Schulte et al., 2022), perennializing marginal lands or integrating perennial bioenergy feedstocks in portions of current row crop systems could provide both. Multifunctional production systems—those that provide standard commodities (i.e., food, fuel and fiber) as well as ecological services—will also play a role in supporting and sustaining a growing bioeconomy (Jarecki et al., 2020). Integrated annual row crop and perennial systems can also increase recreational opportunities and protect biodiversity and water resources (Jordan et al., 2007). A challenge, however, remains identifying lands that should be targeted for bioenergy feedstock cultivation while avoiding negative land-use change. The Scaling Up Perennial Bioenergy Economics and Ecosystem Services Tool (SUPERBEEST) is being developed to facilitate the adoption of perennial bioenergy crops in the U.S Midwest. This tool is comparable to what is reported in Brandes et al. (2018) but for a larger region of the Unites States. Economically and/or environmentally marginal cropland is identified and marginalities are based on criteria identified by Ssegane and Negri (2016). National Commodity Crop Productivity Index (Albers et al., 2022) data are used for economic assessment, Soil Survey Geographic database data (USDA–NRCS, 2023) are used for soil marginalities, and U.S. Geological Survey information (Weary and Doctor, 2014; Yager et al., 2019) is used for nitrate and pesticide leaching to shallow groundwater (Keefer, 1995). The SUPERBEEST tool can be used in both irrigated and non-irrigated areas to integrate perennial bioenergy crops into existing cropland fields.
Multiple pathways for a sustainable bioeconomy
Significant research in the early 21st century focused on conversion of cellulosic feedstocks to liquid fuels, mainly ethanol. Despite these research and development efforts, pretreatment and conversion of cellulosic feedstock materials to biofuels, at scale, has not been widely implemented. Engineering constraints during the pretreatment process are one of the largest bottlenecks for cellulosic feedstock adoption to liquid fuel conversion and higher-value products. Research to overcome the recalcitrance of lignocellulosic feedstocks such as switchgrass via breeding (Vogel et al., 2013; Xu et al., 2022; Eudes et al., 2023) or pretreatment chemicals (Balan et al., 2009; Dien et al., 2013; Wang et al., 2020) is ongoing. Processing perennial grasses within a biorefinery context could result in a spectrum of marketable products such as acetic acid, acetone, butanol, polyhydroxyalkanoates, enzymes, biochar, bio-oil, biomethane, poly (butylene succinate) composites, hydrogen, isoprenol, isopentenol, xylitol and carotenoids can be obtained in addition to biofuel (Larnaudie et al., 2022).
Additionally, increased ethanol demands for the United States ground transportation sector is unlikely with the expected increase in light-duty electrical vehicles. However, specific modes of transportation, namely, maritime and aviation, will require higher density fuels. The development of sustainable aviation fuels is required to achieve a viable pathway for net-zero emissions in the aviation sector (Bergero et al., 2023). Sustainable aviation fuels derived from perennial grasses is expected to provide sufficient fuels at scale in North America (Vardon et al., 2022). Pamula et al. (2021) conducted a life cycle assessment of potential jet fuel feedstocks and the switchgrass pathway had 44% lower GHG emissions compared to petroleum jet fuel. Gautam et al. (2023) forecasted that jet fuel derived from perennial feedstocks would become more economically competitive due to changing fuel prices in conjunction with emerging C markets. Other emerging technologies that may continue to lower the carbon intensity of biofuels from perennial crops are those that improve carbon capture and storage/utilization (Shahbaz et al., 2021). Implementing carbon capture and storage/utilization at the conversion phase would further reduce the carbon intensity from perennial bioenergy crops while the use of biochar from perennial grass conversion can also contribute to soil health and C mitigation.
Conclusion
Whereas herbaceous biomass once powered transportation and agriculture via draft animals, it can now sustainably and reliably power modern forms of transportation. Research on 9804 has demonstrated that switchgrass can be a sustainable and dependable bioenergy feedstock. The collective research conducted on 9804 highlights how the potential benefits of perennializing current cropping systems may only be elucidated in the long-term. Perennial bioenergy systems will not replace annual row crops on prime agricultural land or fully replace existing conservation programs. To assess the benefits of perennializing current cropping systems, indicators of sustainability (i.e., enhanced ecosystem services such as biodiversity and the protection of water resources) in addition to changes in SOC over time must be examined. Ongoing work on 9804 will continue monitor changes in SOC and GHG emissions on an even longer-term scale, while results from newer projects will provide ecosystem service knowledge at the landscape level. Future development of perennial bioenergy crops should occur considering the opportunity to integrate them into current agricultural landscapes, and their success should be continuously monitored using a diverse suite of assessment tools to maximize sustainable and economic indicators.
Data availability statement
The original contributions presented in the study are included in the article/Supplementary Material, further inquiries can be directed to the corresponding author.
Author contributions
SR: Conceptualization, Writing–review and editing, Investigation, Writing–original draft. MS: Conceptualization, Data curation, Supervision, Writing–review and editing. VJ: Conceptualization, Investigation, Resources, Writing–review and editing. RM: Conceptualization, Investigation, Project administration, Resources, Writing–review and editing. CS: Conceptualization, Investigation, Writing–review and editing. JP: Formal Analysis, Investigation, Methodology, Writing–original draft, Writing–review and editing. DR: Conceptualization, Funding acquisition, Resources, Writing–review and editing. JQ: Conceptualization, Methodology, Writing–original draft. GV: Data curation, Writing–review and editing. KV: Conceptualization, Investigation, Methodology, Writing–review and editing. RF: Conceptualization, Investigation, Methodology, Writing–review and editing.
Funding
The author(s) declare financial support was received for the research, authorship, and/or publication of this article. This research was funded by the U.S. Department of Energy (U.S. DOE), Energy Efficiency and Renewable Energy (EERE), Bioenergy Technologies Office (BETO) Grant number DE-EE0009279. SUPERBEEST development is funded by BETO under Award No. DE-EE0022598. Argonne National Laboratory is managed by UChicago Argonne, LLC, for the U.S. DOE under contract DE-AC02-06CH11357.
Acknowledgments
The U.S. Department of Agriculture (USDA) prohibits discrimination in all its programs and activities on the basis of race, color, national origin, age, disability, and where applicable, sex, marital status, family status, parental status, religion, sexual orientation, genetic information, political beliefs, reprisal, or because all or part of an individual’s income is derived from any public assistance program. (Not all prohibited bases apply to all programs.) USDA is an equal opportunity provider and employer. Mention of commercial products and organizations in this manuscript is solely to provide specific information. It does not constitute endorsement by USDA-ARS over other products and organizations not mentioned.
Conflict of interest
The authors declare that the research was conducted in the absence of any commercial or financial relationships that could be construed as a potential conflict of interest.
Publisher’s note
All claims expressed in this article are solely those of the authors and do not necessarily represent those of their affiliated organizations, or those of the publisher, the editors and the reviewers. Any product that may be evaluated in this article, or claim that may be made by its manufacturer, is not guaranteed or endorsed by the publisher.
Supplementary material
The Supplementary Material for this article can be found online at: https://www.frontiersin.org/articles/10.3389/fenrg.2023.1272877/full#supplementary-material
References
Albers, M. A., Dobos, R. R., and Robotham, M. P. (2022). User guide for the national commodity crop productivity Index (NCCPI). Washington, DC. Version 3.0.
Anandhi, A., Deepa, R., Bhardwaj, A., and Misra, V. (2023). Temperature, precipitation, and agro-hydro-meteorological indicator based scenarios for decision making in Ogallala aquifer region. Water 15 (3), 600. doi:10.3390/w15030600
Asbjornsen, H., Hernandez-Santana, V., Liebman, M., Bayala, J., Chen, J., Helmers, M., et al. (2014). Targeting perennial vegetation in agricultural landscapes for enhancing ecosystem services. Renew. Agric. Food Syst. 29 (2), 101–125. doi:10.1017/S1742170512000385
Balan, V., Bals, B., Chundawat, S. P. S., Marshall, D., and Dale, B. E. (2009). “Lignocellulosic biomass pretreatment using AFEX,” in Biofuels: methods and protocols. Editor J. R. Mielenz (Totowa, NJ: Humana Press), 61–77. doi:10.1007/978-1-60761-214-8_5
Begg, G. S., Cook, S. M., Dye, R., Ferrante, M., Franck, P., Lavigne, C., et al. (2017). A functional overview of conservation biological control. Crop Prot. 97, 145–158. doi:10.1016/j.cropro.2016.11.008
Bergero, C., Gosnell, G., Gielen, D., Kang, S., Bazilian, M., and Davis, S. J. (2023). Pathways to net-zero emissions from aviation. Nat. Sustain. 6 (4), 404–414. doi:10.1038/s41893-022-01046-9
Bonifas, K. D., Walters, D. T., Cassman, K. G., and Lindquist, J. L. (2005). Nitrogen supply affects root:shoot ratio in corn and velvetleaf (Abutilon theophrasti). Weed Sci. 53 (5), 670–675. doi:10.1614/WS-05-002R.1
Brandes, E., McNunn, G. S., Schulte, L. A., Muth, D. J., VanLoocke, A., and Heaton, E. A. (2018). Targeted subfield switchgrass integration could improve the farm economy, water quality, and bioenergy feedstock production. GCB Bioenergy 10 (3), 199–212. doi:10.1111/gcbb.12481
Capinera, J. L. (2010). “Food resources for wildlife,” in Insects and wildlife, 83–104. doi:10.1002/9781444317688.ch3
Csikós, N., and Tóth, G. (2023). Concepts of agricultural marginal lands and their utilisation: a review. Agric. Syst. 204, 103560. doi:10.1016/j.agsy.2022.103560
De Deyn, G. B., Cornelissen, J. H. C., and Bardgett, R. D. (2008). Plant functional traits and soil carbon sequestration in contrasting biomes. Ecol. Lett. 11 (5), 516–531. doi:10.1111/j.1461-0248.2008.01164.x
Dien, B. S., O'Bryan, P. J., Hector, R. E., Iten, L. B., Mitchell, R. B., Qureshi, N., et al. (2013). Conversion of switchgrass to ethanol using dilute ammonium hydroxide pretreatment: influence of ecotype and harvest maturity. Environ. Technol. 34 (13-14), 1837–1848. doi:10.1080/09593330.2013.833640
Eudes, A., Lin, C.-Y., De Ben, C., Ortega, J., Lee, M. Y., Chen, Y.-C., et al. (2023). Field performance of switchgrass plants engineered for reduced recalcitrance. Front. Plant Sci. 14, 1181035. doi:10.3389/fpls.2023.1181035
Fargione, J. E., Cooper, T. R., Flaspohler, D. J., Hill, J., Lehman, C., Tilman, D., et al. (2009). Bioenergy and wildlife: threats and opportunities for grassland conservation. BioScience 59 (9), 767–777. doi:10.1525/bio.2009.59.9.8
Fissore, C., Giardina, C. P., Kolka, R. K., Trettin, C. C., King, G. M., Jurgensen, M. F., et al. (2008). Temperature and vegetation effects on soil organic carbon quality along a forested mean annual temperature gradient in North America. Glob. Change Biol. 14 (1), 193–205. doi:10.1111/j.1365-2486.2007.01478.x
Follett, R. F., Vogel, K. P., Varvel, G. E., Mitchell, R. B., and Kimble, J. (2012). Soil carbon sequestration by switchgrass and No-till maize Grown for bioenergy. BioEnergy Res. 5 (4), 866–875. doi:10.1007/s12155-012-9198-y
Gautam, S., Baral, N. R., Mishra, U., and Scown, C. D. (2023). Impact of bioenergy feedstock carbon farming on sustainable aviation fuel viability in the United States. Proc. Natl. Acad. Sci. 120 (51), e2312667120. doi:10.1073/pnas.2312667120
Gopalakrishnan, G., Cristina Negri, M., and Snyder, S. W. (2011). A novel framework to classify marginal land for sustainable biomass feedstock production. J. Environ. Qual. 40 (5), 1593–1600. doi:10.2134/jeq2010.0539
Haacker, E. M. K., Kendall, A. D., and Hyndman, D. W. (2016). Water level declines in the high Plains aquifer: predevelopment to resource senescence. Groundwater 54 (2), 231–242. doi:10.1111/gwat.12350
Hoover, D. L., Abendroth, L. J., Browning, D. M., Saha, A., Snyder, K., Wagle, P., et al. (2023). Indicators of water use efficiency across diverse agroecosystems and spatiotemporal scales. Sci. Total Environ. 864, 160992. doi:10.1016/j.scitotenv.2022.160992
Jarecki, M., Kariyapperuma, K., Deen, B., Graham, J., Bazrgar, A. B., Vijayakumar, S., et al. (2020). The potential of switchgrass and miscanthus to enhance soil organic carbon sequestration—predicted by DayCent model. Land 9 (12), 509. doi:10.3390/land9120509
Jin, V. L., Schmer, M. R., Stewart, C. E., Mitchell, R. B., Williams, C. O., Wienhold, B. J., et al. (2019). Management controls the net greenhouse gas outcomes of growing bioenergy feedstocks on marginally productive croplands. Sci. Adv. 5 (12), eaav9318. doi:10.1126/sciadv.aav9318
Jin, V. L., Schmer, M. R., Wienhold, B. J., Stewart, C. E., Varvel, G. E., Sindelar, A. J., et al. (2015). Twelve years of stover removal increases soil erosion potential without impacting yield. Soil Sci. Soc. Am. J. 79 (4), 1169–1178. doi:10.2136/sssaj2015.02.0053
Johnson, J. M. F., Allmaras, R. R., and Reicosky, D. C. (2006). Estimating source carbon from crop residues, roots and rhizodeposits using the national grain-yield database. Agron. J. 98 (3), 622–636. doi:10.2134/agronj2005.0179
Johnson, J. M. F., Novak, J. M., Varvel, G. E., Stott, D. E., Osborne, S. L., Karlen, D. L., et al. (2014). Crop residue Mass needed to maintain soil organic carbon levels: can it Be determined? BioEnergy Res. 7 (2), 481–490. doi:10.1007/s12155-013-9402-8
Jordan, N., Boody, G., Broussard, W., Glover, J. D., Keeney, D., McCown, B. H., et al. (2007). Sustainable development of the agricultural bio-economy. Science 316 (5831), 1570–1571. doi:10.1126/science.1141700
Karlen, D. L. (2010). Corn stover feedstock trials to support predictive modeling. GCB Bioenergy 2 (5), 235–247. doi:10.1111/j.1757-1707.2010.01061.x
Keefer, D. A. (1995). Potential for agricultural chemical contamination of aquifers in Illinois: 1995 revision. Ill. State Geol. Surv. Environ. Geol. 148.
Klein, R., and McClure, G. (2023). Agricultural budget calculator. Available at: https://agbudget.unl.edu/.
Landis, D. A., Gardiner, M. M., van der Werf, W., and Swinton, S. M. (2008). Increasing corn for biofuel production reduces biocontrol services in agricultural landscapes. Proc. Natl. Acad. Sci. 105 (51), 20552–20557. doi:10.1073/pnas.0804951106
Lark, T. J., Spawn, S. A., Bougie, M., and Gibbs, H. K. (2020). Cropland expansion in the United States produces marginal yields at high costs to wildlife. Nat. Commun. 11 (1), 4295. doi:10.1038/s41467-020-18045-z
Larnaudie, V., Ferrari, M. D., and Lareo, C. (2022). Switchgrass as an alternative biomass for ethanol production in a biorefinery: perspectives on technology, economics and environmental sustainability. Renew. Sustain. Energy Rev. 158, 112115. doi:10.1016/j.rser.2022.112115
Liebig, M. A., Schmer, M. R., Vogel, K. P., and Mitchell, R. B. (2008). Soil carbon storage by switchgrass Grown for bioenergy. BioEnergy Res. 1 (3), 215–222. doi:10.1007/s12155-008-9019-5
Ma, Z., Wood, C. W., and Bransby, D. I. (2001). Impact of row spacing, nitrogen rate, and time on carbon partitioning of switchgrass. Biomass Bioenergy 20 (6), 413–419. doi:10.1016/S0961-9534(01)00008-3
Meehan, T. D., Hurlbert, A. H., and Gratton, C. (2010). Bird communities in future bioenergy landscapes of the Upper Midwest. Proc. Natl. Acad. Sci., 107(43), 18533–18538. doi:10.1073/pnas.1008475107
Mitchell, R., Vogel, K. P., and Sarath, G. (2008). Managing and enhancing switchgrass as a bioenergy feedstock. Biofuels, Bioprod. Biorefining 2 (6), 530–539. doi:10.1002/bbb.106
Mitchell, R., Vogel, K. P., and Uden, D. R. (2012). The feasibility of switchgrass for biofuel production. Biofuels 3 (1), 47–59. doi:10.4155/bfs.11.153
Mitchell, R. B., and Vogel, K. P. (2016). Grass invasion into switchgrass managed for biomass energy. BioEnergy Res. 9 (1), 50–56. doi:10.1007/s12155-015-9656-4
Mitchell, R. B., Vogel, K. P., Berdahl, J., and Masters, R. A. (2010). Herbicides for establishing switchgrass in the central and northern Great Plains. BioEnergy Res. 3 (4), 321–327. doi:10.1007/s12155-010-9084-4
Moore, K. J., Birrell, S., Brown, R. C., Casler, M. D., Euken, J. E., Hanna, H. M., et al. (2014). Midwest vision for sustainable fuel production. Biofuels 5 (6), 687–702. doi:10.1080/17597269.2015.1015312
Nichols, E., Spector, S., Louzada, J., Larsen, T., Amezquita, S., and Favila, M. E. (2008). Ecological functions and ecosystem services provided by Scarabaeinae dung beetles. Biol. Conserv. 141 (6), 1461–1474. doi:10.1016/j.biocon.2008.04.011
Osborne, S. L., Johnson, J. M. F., Jin, V. L., Hammerbeck, A. L., Varvel, G. E., and Schumacher, T. E. (2014). The impact of corn residue removal on soil aggregates and particulate organic matter. BioEnergy Res. 7 (2), 559–567. doi:10.1007/s12155-014-9413-0
Pamula, A. S. P., Lampert, D. J., and Atiyeh, H. K. (2021). Well-to-wake analysis of switchgrass to jet fuel via a novel co-fermentation of sugars and CO2. Sci. Total Environ. 782, 146770. doi:10.1016/j.scitotenv.2021.146770
Perrin, R., Vogel, K., Schmer, M., and Mitchell, R. (2008). Farm-scale production cost of switchgrass for biomass. BioEnergy Res. 1 (1), 91–97. doi:10.1007/s12155-008-9005-y
Porter, P. A., Mitchell, R. B., and Moore, K. J. (2015). Reducing hypoxia in the Gulf of Mexico: reimagining a more resilient agricultural landscape in the Mississippi River Watershed. J. Soil Water Conservation 70 (3), 63A–68A. doi:10.2489/jswc.70.3.63A
Ramirez, S., Schmer, M. R., Jin, V. L., Mitchell, R. B., and Eskridge, K. M. (2023). Near-term effects of perennial grasses on soil carbon and nitrogen in eastern Nebraska. Environments 10 (5), 80. doi:10.3390/environments10050080
Rosa, A. T., Diaz, D. A. R., Hansel, F. D., Sebastian, J. S. V., and Adee, E. A. (2019). Genotypic variation on root growth and nutrient uptake in corn and soybean. Agrosystems, Geosciences Environ. 2 (1), 1–12. doi:10.2134/age2019.03.0018
Schmer, M. R., Jin, V. L., and Wienhold, B. J. (2015). Sub-surface soil carbon changes affects biofuel greenhouse gas emissions. Biomass Bioenergy 81, 31–34. doi:10.1016/j.biombioe.2015.05.011
Schmer, M. R., Mitchell, R. B., Vogel, K. P., Schacht, W. H., and Marx, D. B. (2010). Spatial and temporal effects on switchgrass stands and yield in the Great Plains. BioEnergy Res. 3 (2), 159–171. doi:10.1007/s12155-009-9045-y
Schmer, M. R., Vogel, K. P., Mitchell, R. B., and Perrin, R. K. (2008). Net energy of cellulosic ethanol from switchgrass. Proc. Natl. Acad. Sci., 105(2), 464–469. doi:10.1073/pnas.0704767105
Schmer, M. R., Vogel, K. P., Varvel, G. E., Follett, R. F., Mitchell, R. B., and Jin, V. L. (2014). Energy potential and greenhouse gas emissions from bioenergy cropping systems on marginally productive cropland. PLOS ONE 9 (3), e89501. doi:10.1371/journal.pone.0089501
Schulte, L. A., Dale, B. E., Bozzetto, S., Liebman, M., Souza, G. M., Haddad, N., et al. (2022). Meeting global challenges with regenerative agriculture producing food and energy. Nat. Sustain. 5 (5), 384–388. doi:10.1038/s41893-021-00827-y
Shahbaz, M., AlNouss, A., Ghiat, I., McKay, G., Mackey, H., Elkhalifa, S., et al. (2021). A comprehensive review of biomass based thermochemical conversion technologies integrated with CO2 capture and utilisation within BECCS networks. Resour. Conservation Recycl. 173, 105734. doi:10.1016/j.resconrec.2021.105734
Six, J., Elliott, E. T., Paustian, K., and Doran, J. W. (1998). Aggregation and soil organic matter accumulation in cultivated and native grassland soils. Soil Sci. Soc. Am. J. 62 (5), 1367–1377. doi:10.2136/sssaj1998.03615995006200050032x
Smidt, S. J., Haacker, E. M. K., Kendall, A. D., Deines, J. M., Pei, L., Cotterman, K. A., et al. (2016). Complex water management in modern agriculture: trends in the water-energy-food nexus over the High Plains Aquifer. Sci. Total Environ. 566-567, 988–1001. doi:10.1016/j.scitotenv.2016.05.127
Ssegane, H., and Negri, M. C. (2016). An integrated landscape designed for commodity and bioenergy crops for a tile-drained agricultural watershed. J. Environ. Qual. 45 (5), 1588–1596. doi:10.2134/jeq2015.10.0518
Stewart, C. E., Follett, R. F., Pruessner, E. G., Varvel, G. E., Vogel, K. P., and Mitchell, R. B. (2015). Nitrogen and harvest effects on soil properties under rainfed switchgrass and no-till corn over 9 years: implications for soil quality. GCB Bioenergy 7 (2), 288–301. doi:10.1111/gcbb.12142
Stewart, C. E., Follett, R. F., Pruessner, E. G., Varvel, G. E., Vogel, K. P., and Mitchell, R. B. (2016). N fertilizer and harvest impacts on bioenergy crop contributions to SOC. GCB Bioenergy 8 (6), 1201–1211. doi:10.1111/gcbb.12326
Stewart, C. E., Miner, G. L., Jin, V., Schmer, M. R., Williams, C., and Mitchell, R. B. (2022). Soil Carbon and Agroecosystem Benefits of Conservation Management and Perennial Bioenergy Crop Production. Great Plains Soil Fertility Conference.
USDA–NRCS (2023). Web soil survey. from: https://www.nrcs.usda.gov/wps/portal/nrcs/detail/soils/survey/?cid=nrcs142p2_053627 (Accessed July 25, 2023).
Valentine, J., Clifton-Brown, J., Hastings, A., Robson, P., Allison, G., and Smith, P. (2012). Food vs. fuel: the use of land for lignocellulosic ‘next generation’ energy crops that minimize competition with primary food production. GCB Bioenergy 4 (1), 1–19. doi:10.1111/j.1757-1707.2011.01111.x
Vardon, D. R., Sherbacow, B. J., Guan, K., Heyne, J. S., and Abdullah, Z. (2022). Realizing “net-zero-carbon” sustainable aviation fuel. Joule, 6(1), 16–21. doi:10.1016/j.joule.2021.12.013
Varvel, G. E., Vogel, K. P., Mitchell, R. B., Follett, R. F., and Kimble, J. M. (2008). Comparison of corn and switchgrass on marginal soils for bioenergy. Biomass Bioenergy 32 (1), 18–21. doi:10.1016/j.biombioe.2007.07.003
Vogel, K. P. (1996). Energy production from forages (or American agriculture—back to the future). J. Soil Water Conservation 51 (2), 137–139. https://www.jswconline.org/content/jswc/51/2/137.full.pdf.
Vogel, K. P., Dien, B. S., Jung, H. G., Casler, M. D., Masterson, S. D., and Mitchell, R. B. (2011). Quantifying actual and theoretical ethanol yields for switchgrass Strains using NIRS analyses. BioEnergy Res. 4 (2), 96–110. doi:10.1007/s12155-010-9104-4
Vogel, K. P., Mitchell, R. B., Sarath, G., Jung, H. G., Dien, B. S., and Casler, M. D. (2013). Switchgrass biomass composition altered by six generations of divergent breeding for digestibility. Crop Sci. 53 (3), 853–862. doi:10.2135/cropsci2012.09.0542
Wang, F., Shi, D., Han, J., Zhang, G., Jiang, X., Yang, M., et al. (2020). Comparative study on pretreatment processes for different utilization purposes of switchgrass. ACS Omega 5 (35), 21999–22007. doi:10.1021/acsomega.0c01047
Wayman, S., Bowden, R. D., and Mitchell, R. B. (2014). Seasonal changes in shoot and root nitrogen distribution in switchgrass (Panicum virgatum). BioEnergy Res. 7 (1), 243–252. doi:10.1007/s12155-013-9365-9
Weary, D. J., and Doctor, D. H. (2014). Karst in the United States: a digital map compilation and database. U.S. Geol. Surv. Open-File Rep. doi:10.3133/ofr20141156
Weaver, J. E., and Darland, R. W. (1949). Soil-root relationships of certain native grasses in various soil types. Ecol. Monogr. 19 (4), 303–338. doi:10.2307/1943273
Werling, B. P., Dickson, T. L., Isaacs, R., Gaines, H., Gratton, C., Gross, K. L., et al. (2014). Perennial grasslands enhance biodiversity and multiple ecosystem services in bioenergy landscapes. Proc. Natl. Acad. Sci. 111 (4), 1652–1657. doi:10.1073/pnas.1309492111
Whelan, C. J., Wenny, D. G., and Marquis, R. J. (2008). Ecosystem services provided by birds. Ann. N. Y. Acad. Sci. 1134 (1), 25–60. doi:10.1196/annals.1439.003
Xu, N., Kang, M., Zobrist, J. D., Wang, K., and Fei, S.-z. (2022). Genetic transformation of recalcitrant upland switchgrass using morphogenic genes. Front. Plant Sci. 12, 781565. doi:10.3389/fpls.2021.781565
Yager, R. M., Kauffman, L. J., Soller, D. R., Haj, A. E., Heisig, P. M., Buchwald, C. A., et al. (2019). Characterization and occurrence of confined and unconfined aquifers in Quaternary sediments in the glaciated conterminous United States. U.S. Geol. Surv. Sci. Investig. Rep. doi:10.3133/sir20185091
Keywords: bioeconomy, bioenergy, corn, switchgrass, soil organic carbon
Citation: Ramirez S II, Schmer MR, Jin VL, Mitchell RB, Stewart CE, Parsons J, Redfearn DD, Quinn JJ, Varvel GE, Vogel KP and Follett RF (2024) Perennializing marginal croplands: going back to the future to mitigate climate change with resilient biobased feedstocks. Front. Energy Res. 11:1272877. doi: 10.3389/fenrg.2023.1272877
Received: 04 August 2023; Accepted: 26 December 2023;
Published: 15 January 2024.
Edited by:
Mohammad Rehan, King Abdulaziz University, Saudi ArabiaReviewed by:
Nicholas R. Jordan, University of Minnesota Twin Cities, United StatesCopyright © 2024 Ramirez, Schmer, Jin, Mitchell, Stewart, Parsons, Redfearn, Quinn, Varvel, Vogel and Follett. This is an open-access article distributed under the terms of the Creative Commons Attribution License (CC BY). The use, distribution or reproduction in other forums is permitted, provided the original author(s) and the copyright owner(s) are credited and that the original publication in this journal is cited, in accordance with accepted academic practice. No use, distribution or reproduction is permitted which does not comply with these terms.
*Correspondence: Gary E. Varvel, Z2FyeS52YXJ2ZWxAYXJzLnVzZGEuZ292
†Retired