- Post Graduate Department of Biotechnology, St. Xavier's College (Autonomous), Kolkata, India
Reactive oxygen species (ROS) were initially recognized as toxic by-products of aerobic metabolism. In recent years, it has become apparent that ROS plays an important signaling role in plants, controlling processes such as growth, development and especially response to biotic and abiotic environmental stimuli. The major members of the ROS family include free radicals like O•−2, OH• and non-radicals like H2O2 and 1O2. The ROS production in plants is mainly localized in the chloroplast, mitochondria and peroxisomes. There are secondary sites as well like the endoplasmic reticulum, cell membrane, cell wall and the apoplast. The role of the ROS family is that of a double edged sword; while they act as secondary messengers in various key physiological phenomena, they also induce oxidative damages under several environmental stress conditions like salinity, drought, cold, heavy metals, UV irradiation etc., when the delicate balance between ROS production and elimination, necessary for normal cellular homeostasis, is disturbed. The cellular damages are manifested in the form of degradation of biomolecules like pigments, proteins, lipids, carbohydrates, and DNA, which ultimately amalgamate in plant cellular death. To ensure survival, plants have developed efficient antioxidant machinery having two arms, (i) enzymatic components like superoxide dismutase (SOD), catalase (CAT), ascorbate peroxidase (APX), guaiacol peroxidase (GPX), glutathione reductase (GR), monodehydroascorbate reductase (MDHAR), and dehydroascorbate reductase (DHAR); (ii) non-enzymatic antioxidants like ascorbic acid (AA), reduced glutathione (GSH), α-tocopherol, carotenoids, flavonoids, and the osmolyte proline. These two components work hand in hand to scavenge ROS. In this review, we emphasize on the different types of ROS, their cellular production sites, their targets, and their scavenging mechanism mediated by both the branches of the antioxidant systems, highlighting the potential role of antioxidants in abiotic stress tolerance and cellular survival. Such a comprehensive knowledge of ROS action and their regulation on antioxidants will enable us to develop strategies to genetically engineer stress-tolerant plants.
Introduction
Molecular oxygen was introduced to the early reducing atmosphere of the Earth about 2.7 billion years ago by O2- evolving photosynthetic organisms, causing the advent of the reactive oxygen species (ROS) as unwanted byproducts (Halliwell, 2006). Aerobic metabolism constantly generates ROS which are confined to the different plant cellular compartments, like the chloroplast, mitochondria and peroxisomes. Recent findings also shed light on the role of apoplast as a site for ROS generation (Jubany-Marí et al., 2009; Roychoudhury and Basu, 2012). Under favorable conditions, ROS is constantly being generated at basal levels. However, they are unable to cause damage, as they are being scavenged by different antioxidant mechanisms (Foyer and Noctor, 2005). The delicate balance between ROS generation and ROS scavenging is disturbed by the different types of stress factors like salinity, drought, extreme temperatures, heavy metals, pollution, high irradiance, pathogen infection, etc (Figure 1). The survival of the plants, therefore depends on many important factors like change in growth conditions, severity and duration of stress conditions and the capacity of the plants to quickly adapt to changing energy equation (Miller et al., 2010). Estimates show that only 1–2% of the O2 consumption by plant tissues, leads to the formation of ROS.
The ROS mainly comprise of 1O2, H2O2, O•−2, and OH•. These are very lethal and causes extensive damage to protein, DNA and lipids and thereby affects normal cellular functioning (Apel and Hirt, 2004; Foyer and Noctor, 2005). Redox homeostasis in plants during stressful conditions, is maintained by two arms of the antioxidant machinery—the enzymatic components comprising of the superoxide dismutase (SOD), ascorbate peroxidase (APX), guaiacol peroxidase (GPX), glutathione-S-transferase (GST), and catalase (CAT), and the non-enzymatic low molecular compounds like ascorbic acid (AA), reduced glutathione (GSH), α-tocopherol, carotenoids, phenolics, flavonoids, and proline (Gill and Tuteja, 2010; Miller et al., 2010; Gill et al., 2011). The omnipresent nature of both arms of the antioxidant machinery underlies the necessity of detoxification of ROS for cellular survival (Gill et al., 2011). In this review, we will primarily delve deeper into the domains of ROS, the antioxidant machinery and how they synergistically counteract the effects of environmental stress.
Types of ROS
Phototrophs convert light energy from the sun into biochemical energy and therefore are crucial for sustaining life on Earth. The price they have to pay for this is to face the risk of oxidative damages, because of the different types of ROS, namely, 1O2(singlet oxygen), H2O2(hydrogen peroxide), O•−2 (superoxide radical), and OH• (hydroxyl radical), generated as unwanted byproducts (Table 1). These are generated from only 1–2% of total O2 consumed by plants (Bhattacharjee, 2005). The reactions generating the different ROS members are shown (Figure 2).
Superoxide Radical (O•−2)
The ROS is being constantly generated in the chloroplasts due to partial reduction of O2 or as a result of transfer of energy to O2. The superoxide radical (O•−2) is formed mainly in the thylakoid-localized PSI during non-cyclic electron transport chain (ETC), as well as other cellular compartments. Normally, H2O is generated when cytochrome c oxidase interacts with O2. Occasionally, O2 reacts with the different ETC components to give rise to the O•−2. It is usually the first ROS to be formed. Superoxide radical (O•−2) can also undergo further reactions to generate other members of the ROS family.
O•−2 being moderately reactive with a short half-life of 2–4 μs, does not cause extensive damage by itself. Instead, it undergoes transformation into more reactive and toxic OH• and 1O2 and cause membrane lipid peroxidation (Halliwell, 2006).
Singlet Oxygen (1O2)
Singlet Oxygen is an atypical ROS which is generated not by electron transfer to O2, but rather by the reaction of chlorophyll (Chl) triplet state in the antenna system with O2.
Environmental stresses like salinity, drought and heavy metals cause stomatal closure, leading to insufficient intracellular CO2 concentration. This favors the formation of 1O2. Singlet oxygen can cause severe damages to both the photosystems, PSI and PSII, and puts the entire photosynthetic machinery into jeopardy. Even though 1O2 has a short half-life of about 3 μs (Hatz et al., 2007), it can manage to diffuse some 100 nanometers and causes damage to wide range of targets. These include molecules like proteins, pigments, nucleic acids and lipids (Wagner et al., 2004; Krieger-Liszkay et al., 2008), and is the major ROS responsible for light-induced loss of PSII activity, eliciting cellular death. Plants have managed to efficiently scavenge 1O2 with the help of β-carotene, tocopherol, plastoquinone, and can also react with the DI protein of PSII. Alternatively, singlet oxygen plays a role in up regulating genes which are responsible for providing protection against photo-oxidative stress (Krieger-Liszkay et al., 2008).
Hydrogen Peroxide (H2O2)
Hydrogen peroxide, a moderately reactive ROS is formed when O•−2 undergoes both univalent reduction as well as protonation. It can occur both non-enzymatically by being dismutated to H2O2 under low pH conditions, or mostly by a reaction catalyzed by SOD.
H2O2 is produced in plant cells not only under normal conditions, but also by oxidative stress, caused by factors like drought, chilling, intense light, UV radiation, wounding, and pathogen infection (Sharma et al., 2012). Due to stomatal closure and low availability of CO2 and its limited fixation, Ribulose 1, 5-bisphosphate (RuBP) oxygenation is favored and thus photorespiration is enhanced. This accounts for more than 70% of the H2O2 produced as a result of drought stress (Noctor et al., 2002). The major sources of H2O2 production in plant cells include the ETC in the chloroplast, mitochondria, ER, cell membrane, β-oxidation of fatty acid and photorespiration. Additional sources comprise of different reactions involving photo-oxidation by NADPH oxidase and xanthine oxidase (XOD).
H2O2 in plants behaves like double-edged sword; it is beneficial at low concentrations, but damaging at higher concentrations in the cell. At low intracellular concentrations, it acts as a regulatory signal for essential physiological processes like senescence (Peng et al., 2005), photorespiration and photosynthesis (Noctor et al., 2002), stomatal movement (Bright et al., 2006), cell cycle and growth and development (Tanou et al., 2009a,b). Due to its significantly longer half-life of 1 ms, compared to other ROS members, it can traverse longer distances and cross plant cell membranes. It can cross membranes via aquaporins and cover considerable lengths within the cell (Bienert et al., 2007) and cause oxidative damage. H2O2 at high intracellular concentration oxidizes both cysteine (-SH) and methionine (-SCH3) residues and inactivates Calvin cycle enzymes, Cu/Zn SOD and Fe-SOD by oxidizing their thiol groups (Halliwell, 2006). It causes 50% loss in activity of different enzymes like fructose 1, 6 bisphosphatase, sedoheptulose 1, 7 bisphosphatase and phosphoribulokinase, at concentrations of 10 μM H2O2 and is also responsible for programmed cell death at high cellular concentrations (Dat et al., 2000). However, like O•−2, H2O2 is moderately reactive; therefore, its damage is fully realized only when it is converted into more reactive species.
Hydroxyl Radical (OH•)
Among its family members, hydroxyl radical (OH•) is the most reactive and the most toxic ROS known. It is generated at neutral pH by the Fenton reaction between H2O2 and O•−2 catalyzed by transition metals like Fe (Fe2+, Fe3+).
It has the capability to damage different cellular components by lipid peroxidation (LPO), protein damage and membrane destruction. Since there is no existing enzymatic system to scavenge this toxic radical, excess accumulation of OH• causes the cellular death (Pinto et al., 2003).
Sites of ROS Production in Plant Cells
The ROS is being produced under both normal and stressful conditions at various locations in the chloroplasts, mitochondria, peroxisomes, plasma membranes, ER and the cell wall. In presence of light, chloroplasts and peroxisomes are the major sources of ROS production, while the mitochondrion is the leading producer of ROS under dark conditions (Choudhury et al., 2013).
Chloroplast
The chloroplast comprises of an extremely ordered system of thylakoid membranes which houses the light capturing photosynthetic machinery as well as anatomical requirements for efficient light harvesting (Pfannschmidt, 2003). The photosystems, PSI and PSII which form the core of the light harvesting system in the thylakoids are the major sources of ROS production. Abiotic stress factors like drought, salinity, temperature extremes, all of which cause water stress and limit CO2 concentrations, coupled with excess light, leads to the formation of O•−2 at the PS, via the Mehler reaction.
Subsequently, a membrane-bound Cu/Zn SOD at the PSI converts O•−2 into H2O2 (Miller et al., 2010). The other accomplices of leaking electrons from the ETC of PSI are the 2Fe-2S and the 4Fe-4S clusters. In the PSII, seepage of electrons occurs, via the QA and QB electron acceptors and is responsible for the generation of O•−2. The superoxide radical then goes onto converting itself into more toxic ROS like OH• via H2O2 intermediate by the Fenton reaction at the Fe-S centers. The PSII is also responsible for the generation of 1O2 and this occurs in two ways. Firstly, when environmental stress upsets the delicate balance between light harvesting and energy utilization, it leads to the formation of triplet Chl (3Chl*) which on reacting with dioxygen (3O2) liberates singlet oxygen (1O2) (Karuppanapandian et al., 2011). Secondly, when the ETC is over reduced, the light harvesting complex (LHC) at the PSII generates 1O2 (Asada, 2006). The 1O2 accumulating in the chloroplast causes peroxidation of membrane lipids, and especially Polyunsaturated Fatty Acids (PUFA) and damages membrane proteins which put the P680 reaction center of PSII at risk. It could also directly lead to cell death (Møller etal., 2007; Triantaphylidès et al., 2008). The involvement of the chloroplast in oxidative stress-induced programmed cell death was revealed when animal anti-apoptotic Bcl-2 was expressed in transgenic tobacco (Chen and Dickman, 2004). The 1O2 can also initiate a genetic program, via the EXECUTOR1 and EXECUTOR2 pathways and lead to growth inhibition in plants (Lee et al., 2007). Thus, the chloroplast is a major source of ROS production in plants. To ensure the continual survival of plants under stress, controlling and scavenging the ROS in the chloroplast is very essential, as shown in transgenic plants, as well in stress-tolerant cultivars (Tseng et al., 2007).
Mitochondria
Mitochondria are also the site of generation of harmful ROS, like H2O2 and O•−2 (Navrot et al., 2007), though in a smaller scale. Plant mitochondria differ from animal counterparts in having O2 and carbohydrate-rich environment (Rhoads et al., 2006) and also being involved in photorespiration. The mitochondrial ETC (mtETC) is the major culprit as it houses sufficiently energized electrons to reduce O2 to form the ROS. The two major components of the mtETC responsible for producing ROS are Complex I and Complex III (Møller etal., 2007; Noctor et al., 2007). The NADH Dehydrogenase or Complex I directly reduces O2 to O•−2 in its flavoprotein region. The ROS production at Complex I is further enhanced when there is reverse electron flow from Complex III to Complex I due to lack of NAD+-linked substrates. This reverse flow of electrons is controlled by ATP hydrolysis (Turrens, 2003). In Complex III, ubiquinone in its fully reduced form donates an electron to Cytochrome c1 leaving behind an unstable ubisemiquinone semi-radical which favors leakage of electrons to O2, thereby generating O•−2 (Murphy, 2009). Other sources of ROS production in the mitochondria are the various enzymes present in the mitochondrial matrix. This include enzymes like aconitase which directly produces ROS and others like 1-Galactono-γ-lactone dehydrogenase (GAL) which indirectly produce ROS by feeding electrons to the ETC (Rasmusson et al., 2008). Even though O•−2 is the leading ROS in the mitochondria, it is converted to H2O2 by the Mn-SOD and the APX (Sharma et al., 2012). Estimates show that 1–5% of the total O2 consumption by the mitochondria is diverted toward production of H2O2. Mitochondrion generally produces ROS during normal conditions, but is greatly boosted at times of abiotic stress conditions (Pastore et al., 2007). Such stressful conditions affect the tight coupling of ETC and ATP synthesis, leading to over reduction of electron carriers like ubiquinone (UQ) pool, thereby generating ROS (Rhoads et al., 2006; Blokhina and Fagerstedt, 2010). Since respiratory rate increases during drought, the mitochondrial ATP synthesis increases to compensate for the lower rate of chloroplast ATP synthesis, enhancing the mitochondrial ROS production (Atkin and Macherel, 2009). To counteract this oxidative stress in the mitochondria, two enzymes, Mitochondrial Alternative Oxidase (AOX) and Mitochondrial SOD (Mn-SOD) are very crucial. The AOX maintains the reduced state of the UQ pool and cuts down the ROS production. Its importance is evident from the fact that Arabidopsis lacking a functional AOX is sensitive to drought stress and has altered transcription profiles of different components of the antioxidant machinery (Ho et al., 2008). On the other hand, the higher activity of Mn-SOD clearly made the difference between a salt-tolerant cultivar and a salt-sensitive cultivar of tomato under salinity stress (Mittova et al., 2003).
Peroxisomes
Peroxisomes are single-membrane-bound spherical microbodies and are the major sites of intracellular H2O2 production due to their integral oxidative metabolism (Luis et al., 2006; Palma et al., 2009). They also produce O•−2, like chloroplasts and mitochondria during the course of various metabolic process. The O•−2 is generated at two different locations. The Xanthine oxidase (E.C.1.17.3.2), located in the peroxisomal matrix, metabolizes both xanthine and hypoxanthine into uric acid and generate O•−2 as a by-product. Second is the NADPH-dependent small ETC, composed of NADH and Cyt b localized in the peroxisomal membrane which utilizes O2 as the electron acceptor and releases O•−2 into the cytosol. Additionally, Peroxisomal Membrane Polypeptides (PMPs) of molecular masses 18, 29, and 132 kDa are the three integral membrane proteins responsible for O•−2 production. The NADH acts as the electron donor of the 18 and 32 kDa PMPs, whereas the 29 kDa PMP uses the NADPH as the electron donor to reduce Cytochrome c. During stressful conditions, when the availability of water is low and stomata remains closed, the ratio of CO2 to O2 reduces considerably which causes increased photorespiration leading to glycolate formation. This glycolate is oxidized by the glycolate oxidase in peroxisome to release H2O2, making it the leading producer of H2O2 during photorespiration (Noctor et al., 2002). Besides, there are other supplemental metabolic processes like β-oxidation of fatty acids, flavin oxidase pathway and the disproportionation of O•−2 radicals for peroxisomal ROS production.
Apoplast
Apoplast, the diffusible space around the plant cell membrane is responsible for converting the incoming CO2 into a soluble, diffusible form which enters the cytosol to undergo photosynthesis. At times of adverse environmental conditions, stress signals combined with abscisic acid (ABA) make the apoplast a prominent site for H2O2 production (Hu et al., 2006). The NADPH oxidases expressed by the AtRbohD and AtRbohF in the guard cells and the mesophyll cells of Arabidopsis, account for generating the apoplastic ROS which is required for ABA-induced stomatal closure (Kwak et al., 2003). Besides these enzymes, there are additional ROS-generating enzymes which comprise of pH dependent peroxidases (POXs), cell wall-linked oxidases, germin-like oxalate oxidases and polyamine oxidases, all of which mainly produce H2O2.
Plasma Membranes
Plasma membrane which surrounds the entire plant cell plays an important role in interacting with the ever changing environmental conditions and provides information necessary for the continual survival of the cell. The NADPH-dependent-oxidases which are localized in the plasma membrane are in the spotlight due to their gene expression and presence of different homologs during different stress conditions (Apel and Hirt, 2004). The NADPH oxidase produces O•−2 by transferring electrons from cytosolic NADPH to O2, which either spontaneously dismutates to H2O2 or is catalyzed by SOD. The fact that NADPH oxidase plays an important role in plant defense against pathogenic infection and abiotic stress conditions (Kwak et al., 2003) is well supported.
Cell Walls
During stress, the cell wall-localized lipoxygenase (LOX) causes hydroperoxidation of polyunsaturated fatty acids (PUFA) making it active source of ROS like OH•, O•−2, H2O2, and 1O2. The cell wall-localized diamine oxidases utilize diamines or polyamines to generate ROS in the cell wall. During pathogen attack, lignin precursors undergo extensive cross-linking, via H2O2-mediated pathways to reinforce the cell wall with lignin (Higuchi, 2006).
Endoplasmic Reticulum (ER)
The NADPH-mediated electron transport involving CytP450, localized in the ER generates O•−2 (Mittler, 2002). Organic substrate, RH interacts with the CytP450 followed by reduction by a flavoprotein to give rise to a free radical intermediate (Cyt P450 R−). This intermediate promptly reacts with triplet oxygen (3O2) to form an oxygenated complex (Cyt P450-ROO−). The complex may occasionally decompose to Cyt P450-Rh by generating O•−2 as byproduct.
Targets of ROS
ROS is known to cause damages to biomolecules such as lipids, proteins and DNA (Figure 3).
Lipids
Lipids form a major portion of the plasma membrane which envelopes the cell and helps it to adapt to the changing environment. However, under stressful conditions, when the level of ROS rise above the threshold value, LPO becomes so damaging that it is often considered as the single parameter to gauge lipid destruction. LPO starts a chain reaction and further exacerbates oxidative stress by creating lipid radicals which damages proteins and DNA. The two main targets of the ROS in membrane phospholipids are the double bond between C-atoms and the ester linkage between glycerol and fatty acids. The PUFA which are important components of the plasma membrane are the hotspots for ROS damage. PUFAs like linoleic and linolenic acid are specifically prone to attack by ROS like 1O2 and OH•. The hydroxyl radical (OH•) is the most damaging member as it has the ability to trigger a cyclic chain reaction and cause further peroxidation of other PUFAs.
The entire process of LPO can be divided into three distinct phases, Initiation, Progression, and Termination. Initiation involves energizing the O2 (a rate limiting step) to give rise to radicals like O•−2 and OH•. These ROS react with the methylene groups of the PUFA, yielding conjugated dienes, lipid peroxyl radicals and hydroperoxides (Smirnoff, 2000).
The PUFA peroxyl radical once formed possesses the ability to further propagate the LPO by extracting one H-atom from adjoining PUFA side chains.
The lipid hydroperoxide (PUFA-OOH) undergoes cleavage by reacting with reduced metals such as Fe2+.
The lipid hydroperoxides can also undergo decomposition to form different reactive species such as lipid alkoxyl radicals, aldehydes, alkanes, lipid epoxides, and alcohols. LPO terminates with the formation of different lipid dimers caused by different lipid derived radicals.
Overall, the LPO increases membrane fluidity causing the membrane to be leaky to substances which otherwise enter the cell through special channels, damage the membrane proteins, deactivate the membrane receptors, membrane-localized enzymes and ion-channels.
Proteins
The ROS produced during stress conditions causes the oxidation of proteins. The protein undergoes different types of modifications which may either be direct or indirect. During direct modifications, the activity of the protein becomes varied as a result of different chemical modifications such as nitrosylation, carboxylation, disulfide bond formation, and glutathionylation. Protein carbonylation is often used as a marker for evaluating protein oxidation (Møller etal., 2007). Indirect modification of proteins can occur as a result of interaction with the products of LPO. The ROS concentration, on crossing its threshold value, leads to the site-specific modification of amino acids like Arg, Lys, Pro, Thr, and Trp, and increased susceptibility to proteolytic degradation (Møller etal., 2007). The amino acids differ in their susceptibility to ROS attack. Amino acids containing thiol groups and sulfur are the most vulnerable. The Cys and Met are both prone to damage by the reactive 1O2 and OH•. The enzymes containing iron-sulfur centers are irreversibly inactivated on getting oxidized by O•−2. The oxidized proteins thus become better targets for proteolytic digestion by getting primed for ubiquitination-mediated proteosomal degradation.
DNA
Since the plant nuclear DNA is well protected by histones and associated proteins, both mitochondrial and chloroplastic DNA bears the brunt of the ROS attack due to lack of protective histones as well as the close proximity to ROS production machinery. Oxidative damage of DNA as a result of ROS occurs at multiple levels which include oxidation of the deoxyribose sugar residue, modification of the nucleotide base, abstraction of a nucleotide, breaks in either DNA strand, and cross-linking of the DNA and protein. The hydroxyl radical not only damages the deoxyribose sugar backbone by extracting H-atom, but also reacts with double bonds of the purine and pyrimidine bases (Halliwell, 2006). The ROS abstracts the C-4 H-atom of the deoxyribose sugar and forms a deoxyribose radical which reacts further to cause single strand breaks in the DNA (Evans et al., 2004). The damaged products as a result of base oxidation include the most common 8-hydroxyquinine and other less common ones like hydroxyl methyl urea, dehydro-2′-deoxyguanosine, thymine glycol, and thymine and adenine ring opened. The OH• is also notorious for creating DNA-protein cross-links when it reacts with either DNA or associated proteins. These cross-links are not easily reparable and may be lethal to the plant cell, if not repaired in time before commencement of critical cellular processes like replication or transcription.
ROS Defense Machinery
The ROS defense mechanism consists of the antioxidant machinery which helps to mitigate the above mentioned oxidative stress-induced damages. The antioxidant machinery has two arms with the enzymatic components and non-enzymatic antioxidants (Table 2).
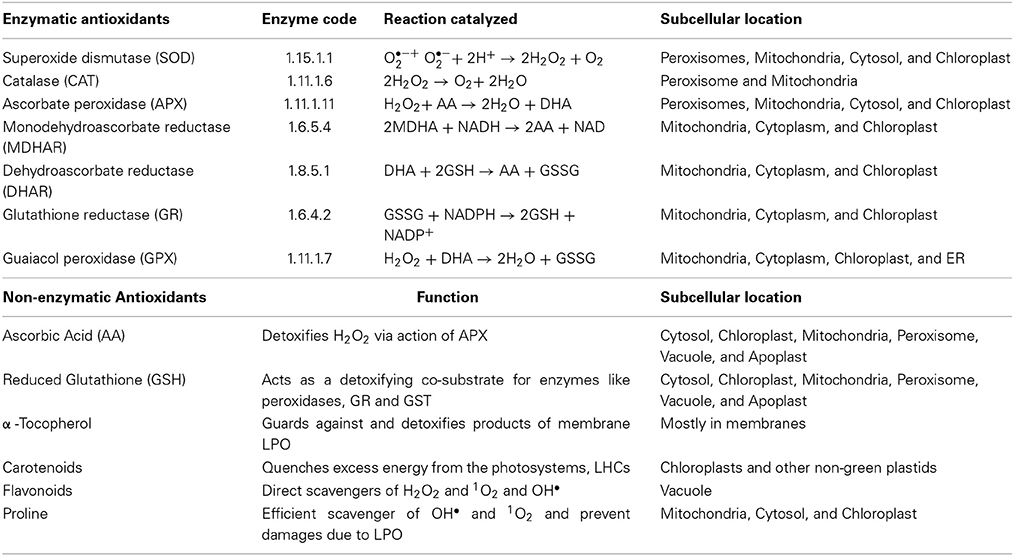
Table 2. List of all the enzymatic and non-enzymatic antioxidants along with their functions and cellular localization.
Enzymatic Antioxidants
The enzymes localized in the different subcellular compartments and comprising the antioxidant machinery include Superoxide Dismutase (SOD), Catalase (CAT), Ascorbate Peroxidase (APX), Monodehydroascorbate reductase (MDHAR), Dehydroascorbate reductase (DHAR), Glutathione Reductase (GR), and Guaiacol Peroxidase (GPX).
Superoxide Dismutase (SOD)
SOD (E.C.1.15.1.1) belongs to the family of metalloenzymes omnipresent in all aerobic organisms. Under environmental stresses, SOD forms the first line of defense against ROS-induced damages. The SOD catalyzes the removal of O•−2 by dismutating it into O2 and H2O2. This removes the possibility of OH• formation by the Haber-Weiss reaction. SODs are classified into three isozymes based on the metal ion it binds, Mn-SOD (localized in mitochondria), Fe-SOD (localized in chloroplasts), and Cu/Zn-SOD (localized in cytosol, peroxisomes, and chloroplasts) (Mittler, 2002). SOD has been found to be up regulated by abiotic stress conditions (Boguszewska et al., 2010).
Catalase (CAT)
CAT (E.C.1.11.1.6) is a tetrameric heme-containing enzyme responsible for catalyzing the dismutation of H2O2 into H2O and O2. It has high affinity for H2O2, but lesser specificity for organic peroxides (R-O-O-R). It has a very high turnover rate (6 × 106 molecules of H2O2 to H2O and O2 min−1) and is unique amongst antioxidant enzymes in not requiring a reducing equivalent. Peroxisomes are the hotspots of H2O2 production due to β-oxidation of fatty acids, photorespiration, purine catabolism and oxidative stress (Mittler, 2002). However, recent reports suggest that CAT is also found in other subcellular compartments such as the cytosol, chloroplast and the mitochondria, though significant CAT activity is yet to be seen (Mhamdi et al., 2010). Angiosperms have been reported to have three CAT genes. CAT1 is expressed in pollens and seeds (localized in peroxisomes and cytosol), CAT2 predominantly expressed in photosynthetic tissues but also in roots and seeds (localized in peroxisomes and cytosol) and finally CAT3 is found to be expressed in leaves and vascular tissues (localized in the mitochondria). Stressful conditions demand greater energy generation and expenditure of the cell. This is fulfilled by increased catabolism which generates H2O2. CAT removes the H2O2 in an energy efficient way.
Ascorbate peroxidase (APX)
APX (E.C.1.1.11.1) is an integral component of the Ascorbate-Glutathione (ASC-GSH) cycle. While CAT predominantly scavenges H2O2 in the peroxisomes, APX performs the same function in the cytosol and the chloroplast. The APX reduces H2O2 to H2O and DHA, using Ascorbic acid (AA) as a reducing agent.
The APX family comprises of five isoforms based on different amino acids and locations, viz., cytosolic, mitochondrial, peroxisomal, and chloroplastid (stromal and thylakoidal) (Sharma and Dubey, 2004). Since APX is widely distributed and has a better affinity for H2O2 than CAT, it is a more efficient scavenger of H2O2 at times of stress.
Monodehydroascorbate reductase (MDHAR)
MDHAR (E.C.1.6.5.4) is responsible for regenerating AA from the short-lived MDHA, using NADPH as a reducing agent, ultimately replenishing the cellular AA pool. Since it regenerates AA, it is co-localized with the APX in the peroxisomes and mitochondria, where APX scavenges H2O2 and oxidizes AA in the process (Mittler, 2002). MDHAR has several isozymes which are confined in chloroplast, mitochondria, peroxisomes, cytosol, and glyoxysomes.
Dehydroascorbate reductase (DHAR)
DHAR (M.C.1.8.5.1) reduces dehydroascorbate (DHA) to AA using Reduced Glutathione (GSH) as an electron donor (Eltayeb et al., 2007). This makes it another agent, apart from MDHAR, which regenerates the cellular AA pool. It is critical in regulating the AA pool size in both symplast and apoplast, thus maintaining the redox state of the plant cell (Chen and Gallie, 2006). DHAR is found abundantly in seeds, roots and both green and etiolated shoots.
Glutathione Reductase (GR)
GR (E.C.1.6.4.2) is a flavoprotein oxidoreductase which uses NADPH as a reductant to reduce GSSG to GSH. Reduced glutathione (GSH) is used up to regenerate AA from MDHA and DHA, and as a result is converted to its oxidized form (GSSG). GR, a crucial enzyme of ASC-GSH cycle catalyzes the formation of a disulfide bond in glutathione disulfide to maintain a high cellular GSH/GSSG ratio. It is predominantly found in chloroplasts with small amounts occurring in the mitochondria and cytosol. GSH is a low molecular weight compound which plays the role of a reductant to prevent thiol groups from getting oxidized, and react with detrimental ROS members like 1O2 and OH•.
Guaiacol peroxidase (GPX)
GPX (E.C.1.11.1.7) is a heme-containing enzyme composed of 40–50 kDa monomers, which eliminates excess H2O2 both during normal metabolism as well as during stress. It plays a vital role in the biosynthesis of lignin as well as defends against biotic stress by degrading indole acetic acid (IAA) and utilizing H2O2 in the process. GPX prefers aromatic compounds like guaiacol and pyragallol (Asada, 1999) as electron donors. Since GPX is active intracellularly (cytosol, vacuole), in the cell wall and extracellularly, it is considered as the key enzyme in the removal of H2O2.
Non-Enzymatic Antioxidants
The non-enzymatic antioxidants form the other half of the antioxidant machinery, comprising of AA, GSH, α-tocopherol, carotenoids, phenolics, flavonoids, and amino acid cum osmolyte proline. They not only protect different components of the cell from damage, but also play a vital role in plant growth and development by tweaking cellular process like mitosis, cell elongation, senescence and cell death (de Pinto and De Gara, 2004).
Ascorbic Acid (AA)
AA is the most abundant and the most extensively studied antioxidant compound. It is considered powerful as it can donate electrons to a wide range of enzymatic and non-enzymatic reactions. Majority of AA in plant cells is the result of Smirnoff-Wheeler pathway, catalyzed by L-galactano-γ-lactone dehydrogenase in the plant mitochondria, with the remaining being generated from D-galacturonic acid. 90% of the AA pool is concentrated not only in the cytosol, but also substantially in apoplast, thus making it the first line of defense against ROS attack (Barnes et al., 2002). AA is oxidized in two successive steps, starting with oxidation into MDHA, which if not reduced immediately to ascorbate, disproportionates to AA and DHA. It reacts with H2O2, OH•, O•−2, and regenerates α-tocopherol from tocopheroxyl radical, thereby protecting the membranes from oxidative damage (Shao et al., 2005). It also protects and preserves the activities of metal-binding enzymes. AA in its reduced state acts as the cofactor of violaxanthine de-epoxidase and maintains the dissipation of the excess excitation energy (Smirnoff, 2000). AA has also been reported to be involved in preventing photo-oxidation by pH-mediated modulation of PSII activity and its down regulation, associated with zeaxanthine formation.
Reduced glutathione (GSH)
Glutathione is a low molecular weight thiol tripeptide (γ-glutamyl-cysteinyl-glycine) abundantly found in almost all cellular compartments like cytosol, ER, mitochondria, chloroplasts, vacuoles, peroxisomes, and even the apoplast. It is involved in a wide range of processes like cell differentiation, cell growth/division, cell death and senescence, regulation of sulfate transport, detoxification of xenobiotics, conjugation of metabolites, regulation of enzymatic activity, synthesis of proteins and nucleotides, synthesis of phytochelatins and finally expression of stress responsive genes (Mullineaux and Rausch, 2005). This versatility of GSH is all due to its high reductive potential. A central cysteine residue with nucleophilic character is the source of its reducing power. GSH scavenges H2O2, 1O2, OH•, and O•−2 and protects the different biomolecules by forming adducts (glutathiolated) or by reducing them in presence of ROS or organic free radicals and generating GSSG as a by-product. GSH also plays a vital role in regenerating AA to yield GSSG. The GSSG thus generated is converted back to GSH, either by de novo synthesis or enzymatically by GR. This ultimately replenishes the cellular GSH pool. GSH also helps in the formation of phytochelatins via phytochelatin synthase (Roychoudhury et al., 2012a), which helps to chelate heavy metal ions and thus scavenges another potential source of ROS formation in plants (Roy Choudhury et al., 2012b). Therefore, the delicate balance between GSH and GSSG is necessary for maintaining the redox state of the cell.
α-tocopherol
The α-tocopherol belongs to a family of lipophilic antioxidants which are efficient scavengers of ROS and lipid radicals, making them indispensable protectors and essential components of biological membranes (Holländer-Czytko et al., 2005; Kiffin et al., 2006). The α-tocopherol has the highest antioxidant capability among the four isomers (α-, β-, γ-, δ-). The tocopherols are synthesized only by photosynthetic organisms and thus only present in green tissues of plants. The α-tocopherol is synthesized from γ-tocopherol by γ- tocopherol-methyl-transferase (γ-TMT encoded by VTE4). Tocopherols are known for their ability to protect lipids and other membrane constituents of the chloroplasts by reacting with O2 and quenching its excess energy, thus protecting the PSII, both structurally and functionally. Tocopherol also serves as an effective free radical trap by halting the chain propagation step of the LPO cycle. It reacts with the lipid radicals RO•, ROO•, and RO* at the membrane-water interface, where α-tocopherol reduces them and itself gets converted into TOH•. The TOH• radical undergoes recycling to its reduced form by interacting with GSH and AA (Igamberdiev et al., 2004).
Carotenoids
Carotenoids belong to family of lipophilic antioxidants which are localized in the plastids of both photosynthetic and non-photosynthetic plant tissues. They are found not only in plants, but also in micro-organisms. They belong to a group of antennae molecules which absorbs light in the 450–570 nm and transfers the energy to the chlorophyll molecule. Carotenoids exhibit their antioxidative activity by protecting the photosynthetic machinery in four ways, (a) reacting with LPO products to end the chain reactions, (b) scavenging 1O2 and generating heat as a by-product, (c) preventing the formation of 1O2 by reacting with 3Chl* and excited chlorophyll (Chl*), and (d) dissipating the excess excitation energy, via the xanthophyll cycle.
Flavonoids
Flavonoids are widely found in the plant kingdom occurring commonly in the leaves, floral organs and pollen grains. Flavonoids can be classified into four classes on the basis of their structure, flavonols, flavones, isoflavones, and anthocyanins. They have diverse roles in providing pigmentation in flowers, fruits and seeds involved in plant fertility and germination of pollen and defense against plant pathogens. Flavonoids have been considered as a secondary ROS scavenging system in plants experiencing damage to the photosynthetic apparatus, due to the excess excitation energy (Fini et al., 2011). They also have a role in scavenging 1O2 and alleviate the damages caused to the outer envelope of the chloroplastic membrane (Agati et al., 2012).
Proline
Proline, an osmolyte is also regarded as a powerful antioxidant. It is widely used across the different kingdoms as a non-enzymatic antioxidant to counteract the damaging effects of different ROS members. Proline is synthesized using glutamic acid as a substrate, via a pyrroline 5-carboxylate (P5C) intermediate. This pathway in plants is catalyzed by two enzymes, ð1-pyrroline-5-carboxylate synthetase (P5CS) and Pyrroline-5-carboxylate reductase (P5CR). It is an efficient scavenger of OH• and 1O2 and can inhibit the damages due to LPO. During stress, proline accumulates in plants in large amounts which is either due to enhanced synthesis or reduced degradation (Verbruggen and Hermans, 2008).
Antioxidant Regulation for Environmental Stress Tolerance
Increased SOD activity in response to drought stress was detected in three different cultivars of Phaseolus vulgaris (Zlatev et al., 2006) and Oryza sativa (Sharma and Dubey, 2005a,b). The SOD activity was found to be heightened during drought stress in the leaves of white clover, viz., Trifolium repens L. (Chang-Quan and Rui-Chang, 2008). The SOD activity was found to be up regulated during salt stress in many plants like chickpea (Kukreja et al., 2005) and tomato (Gapiñska et al., 2008). All three isoforms of SOD have been found to be expressed in chickpea in response to salinity stress (Eyidogan and Öz, 2007). Transgenic Arabidopsis overexpressing Mn-SOD was found to have enhanced salt tolerance (Wang et al., 2004). SOD activity was increased by UV-B radiation in pea, wheat, Arabidopsis and rice, but not affected in barley and soybean. In a field study, supplemental UV-B increased SOD activity in wheat and mungbean, and caused differential responses among soybean cultivars (Agrawal et al., 2009). The CAT activity was found to increase especially in drought-sensitive varieties of wheat (Simova-Stoilova et al., 2010). Cicer arietinum under salt stress also have increased CAT activity in both leaves (Eyidogan and Öz, 2007) and roots (Kukreja et al., 2005). Increased CAT activity under cadmium stress has been reported in Phaseolus aureus, Pisum sativum, Lemna minor, barley and sunflower (Sreedevi and Krishnan, 2012). When the antioxidant profile of drought-tolerant and drought-susceptible genotypes of wheat were compared, it was found out that the drought-tolerant genotype C306 showed higher APX and CAT activity, and AA content with lower H2O2 and MDA content than the drought-susceptible genotype, HD2329 (Sairam et al., 1998). When APX was overexpressed in the chloroplasts of Nicotiana tabacum, it reduced the toxic effects of H2O2 and generated drought tolerance (Badawi et al., 2004). There was also an enhancement in their tolerance to salt stress. UV-B radiation increased APX activity in Arabidopsis thaliana (Rao et al., 1996). The activity of APX positively correlated with Pb treatment in Eichhornia crassipes (water hyacinth) seedlings (Malar et al., 2014). Roychoudhury et al. (2012c) reported that the activities of antioxidative enzymes like GPX and APX increased both in IR-29 (salt-sensitive) and Nonabokra (salt-tolerant) rice varieties during CdCl2 stress; however, the activity was more enhanced in Nonabokra. The CAT activity during Cd stress showed a different trend, with a marked decrease in IR-29, while marked increase in Nonabokra at higher Cd concentration. The activity of peroxidase and CAT increased progressively with the increase in CdCl2 concentration in Vigna radiata (Roychoudhury and Ghosh, 2013). Vaccinium myrtillus L. is regarded as a species which is a successful colonist of acid- and heavy metal-contaminated soil. Upon analysis of the antioxidant response of this plant from heavily polluted sites (immediate vicinity of zinc smelter, iron smelter and power plant), it was found that the contents of GSH, non-protein thiols, proline and activity of GPX were elevated. The GPX activity seemed to be universal, sensitive and correlated well with heavy metal stress (Kandziora-Ciupa et al., 2013). Overexpression of MDHAR in tobacco (Eltayeb et al., 2007) and DHAR in Arabidopsis (Ushimaru et al., 2006) resulted in improved salt tolerance. Stressed rice seedlings displayed increased activity of the enzymes MDHAR, DHAR and GR, all of which are involved in the regeneration of AA (Sharma and Dubey, 2005a,b). Under salt stress, APX and GR activities were found to be higher in salt-tolerant cultivars of potato, while being markedly diminished in salt-sensitive varieties. This sensitivity was attributed to the reduction of APX and GR activity during saline conditions (Aghaei et al., 2009). Marked drought-induced increase in GPX activity was noted in both the sensitive rice varieties IR-29 and Pusa Basmati (Basu et al., 2010a). Exogenous application of AA to wheat cultivars resulted in higher chlorophyll contents, net photosynthesis and growth, compared to the non-treated plants challenged with drought stress (Malik and Ashraf, 2012). It has also been seen that priming Carthamus tinctorius seeds with AA significantly relieved the harsh effects of drought stress on seedling growth (Razaji et al., 2012). When AA was exogenously applied, prior to and during salt stress in tomato seedlings, it helped expedite the recovery process and ensured long-term survival (Shalata and Neumann, 2001). AA also helped to relieve oxidative damage in wheat, by improving photosynthetic capacity and sustaining ion homeostasis (Athar et al., 2008). The greater susceptibility of the sensitive varieties IR-29 and Pusa Basmati to water scarcity was also reflected by considerable decrease in GSH/GSSG ratio, as compared to the tolerant variety Pokkali (Basu et al., 2010b). Both AA and GSH were found to have enhanced levels in salt-tolerant cultivar Pokkali than in the sensitive cultivar Pusa Basmati (Vaidyanathan et al., 2003). Arsenic (III) significantly decreased the GSH content in rice roots, due to its conversion to phytochelatins. The GSH supplementation resulted in partial protection against arsenic stress, reducing the MDA content and restoring the seedling growth of arsenic (V) exposed seedlings (Roychoudhury and Basu, 2012). GSH was also found to lessen the oxidative damage in rice chloroplasts caused due to salinity stress (Wang et al., 2014). Under low UV-B doses, increases in AA and GSH pools, as well as AA regeneration ability functioned to keep the balance of cellular H2O2 (Roychoudhury and Basu, 2012). Studies on heat-acclimated vs. non-acclimated cool season turfgrass species suggested that the former had lower production of ROS, as a result of enhanced synthesis of AA and GSH. In wheat, it was established that heat stress induced the accumulation of GSH levels and increased the activity of the enzymes involved in GSH synthesis and the GSH/GSSG ratio (Hasanuzzaman et al., 2013). When transgenic tobacco overexpressing Arabidopsis VTE1 (encoding tocopherol biosynthesis enzyme) were subjected to drought conditions, they showed decreased LPO, electrolyte leakage and H2O2 content, but had increased chlorophyll compared with the wild type (Liu et al., 2008). Arabidopsis vte1 and vte4 mutants lacking α-tocopherol are particularly sensitive to salt stress, as evident by their reduced growth and increased oxidative stress. This is because α-tocopherol maintains the cellular Na+/K+ homeostasis and hormonal balance (Ellouzi et al., 2013). Acute exposure of UV-B leads to decrease in α-tocopherol levels in plants, possibly reflecting reactions with lipid radicals (Jain et al., 2003). In drought-resistant plants, the number of carotenoid molecules per chlorophyll unit increased under drought stress, thus providing photo-protection from oxidative damages (Munné-Bosch and Alegre, 2000). Water deficit, induced by 20% polyethylene glycol (PEG 6000) treatment to rice seedlings led to increment in antioxidants like flavonoids and phenolics, which were several folds higher in the tolerant cultivar Pokkali, as compared to the sensitive varieties like IR-29 and Pusa Basmati (Basu et al., 2010a). The two isolines of soybean cv. Clark, the normal line with moderate levels of flavonoids and the magenta line with reduced flavonoid levels, were grown in the field with or without natural levels of UV-B. Solar UV-B radiation caused oxidative stress in both the lines and altered ROS metabolism, primarily by decreasing SOD activity and increasing the activities of APX, CAT, and GR. This resulted in decreased AA content and increased DHA content. The magenta line had greater oxidative stress than the normal line, in spite of its enhanced oxidative defense capacity as compared to the normal line, even under UV-B exclusion. These results indicate enhanced sensitivity in the magenta line, especially under UV-B exclusion that was likely due to the absence of flavonoid epidermal screening compounds and subsequent increased penetration of solar ultraviolet radiation into the leaf (Xu et al., 2008). Proline, an osmoprotectant as well as a sink for energy to regulate redox potentials, was found to have increased accumulation in drought-tolerant cultivars of chickpea than sensitive cultivars under both control and drought stress conditions (Mafakheri et al., 2010). In case of rice seedlings, exposed to high salt stress (200 mM NaCl), the antioxidants like anthocyanin and proline showed the highest level in the salt-tolerant cultivar Nonabokra, as compared to the salt-sensitive cultivars like M-1-48 and Gobindobhog (Roychoudhury et al., 2008). The content of flavonoids and proline were also found to be enhanced in salt-tolerant cultivars of indica rice than in the salt-sensitive cultivars, as evident by the reduced membrane damage caused by LPO (Chutipaijit et al., 2009).
Conclusion
The ROS plays the double role of being the inevitable by-product of aerobic metabolism on one hand and serving as a marker during stressful conditions on the other hand. They not only serve as agents of damages in plants, but also trigger stress-signaling components to prevent further damages. ROS synthesis is widespread, with production sites being present in both intracellular and extracellular locations. The damage caused by ROS is extensive and the targets include all biomolecules like lipids, proteins and DNA, damaging the integrity of the cell and ultimately leading to its death. However, evolution has equipped plants with a wider range of defense measures which include changes at the morphological, metabolic and genetic level to adapt to the adverse environmental conditions. This review gives an insight into how both arms of the antioxidant machinery; the antioxidant enzymes and the non-antioxidant metabolites, work in conjunction to alleviate the damaging effects of ROS and develop tolerance against various environmental stress conditions. Although significant progress has been achieved in recent years, there are still ambiguities and gaps in our understanding of ROS formation and how they affect plants, primarily due to their short half-life and highly reactive nature. Although the highly compartmentalized nature of antioxidants is well defined, the sensing and response mechanism as well as the control of the delicate balance between production and scavenging need to be better explored. Several issues remain unanswered, like the interaction between ROS and calcium signaling and the regulation of ROS during multiple environmental stresses. In future, advanced imaging techniques like the markers for Ca2+ imaging can lead to better understanding of ROS metabolism. Advanced functional genomics, coupled with proteomics and metabolomics will offer detailed insights into ROS network and its related responses. There is no doubt that transgenic approach for overexpression of antioxidant gene cassettes can lead to enhanced tolerance to multiple stresses in future (Oztetik, 2012).
Conflict of Interest Statement
The authors declare that the research was conducted in the absence of any commercial or financial relationships that could be construed as a potential conflict of interest.
Acknowledgments
Financial support from Science and Engineering Research Board (SERB), Department of Science and Technology, Government of India through the research grant (SR/FT/LS-65/2010) to Dr. Aryadeep Roychoudhury is gratefully acknowledged.
References
Agati, G., Azzarello, E., Pollastri, S., and Tattini, M. (2012). Flavonoids as antioxidants in plants: location and functional significance. Plant Sci. 196, 67–76. doi: 10.1016/j.plantsci.2012.07.014
Pubmed Abstract | Pubmed Full Text | CrossRef Full Text | Google Scholar
Aghaei, K., Ehsanpour, A. A., and Komatsu, S. (2009). Potato responds to salt stress by increased activity of antioxidant enzymes. J. Integr. Plant Biol. 51, 1095–1103. doi: 10.1111/j.1744-7909.2009.00886.x
Pubmed Abstract | Pubmed Full Text | CrossRef Full Text | Google Scholar
Agrawal, S. B., Singh, S., and Agrawal, M. (2009). Ultraviolet-B inducedchanges in gene expression and antioxidants in plants. Adv. Bot. Res. 52, 47–86. doi: 10.1016/S0065-2296(10)52003-2
Apel, K., and Hirt, H. (2004). Reactive oxygen species: metabolism, oxidative stress, and signal transduction. Annu. Rev. Plant Biol. 55, 373–399. doi: 10.1146/annurev.arplant.55.031903.141701
Pubmed Abstract | Pubmed Full Text | CrossRef Full Text | Google Scholar
Asada, K. (1999). The water-water cycle in chloroplasts: scavenging of active oxygens and dissipation of excess photons. Annu. Rev. Plant Biol. 50, 601–639. doi: 10.1146/annurev.arplant.50.1.601
Pubmed Abstract | Pubmed Full Text | CrossRef Full Text | Google Scholar
Asada, K. (2006). Production and scavenging of reactive oxygen species in chloroplasts and their functions. Plant Physiol. 141, 391–396. doi: 10.1104/pp.106.082040
Pubmed Abstract | Pubmed Full Text | CrossRef Full Text | Google Scholar
Athar, H. U. R., Khan, A., and Ashraf, M. (2008). Exogenously applied ascorbic acid alleviates salt-induced oxidative stress in wheat. Environ. Exp. Bot. 63, 224–231. doi: 10.1016/j.envexpbot.2007.10.018
Atkin, O. K., and Macherel, D. (2009). The crucial role of plant mitochondria in orchestrating drought tolerance. Ann. Bot. 103, 581–597. doi: 10.1093/aob/mcn094
Pubmed Abstract | Pubmed Full Text | CrossRef Full Text | Google Scholar
Badawi, G. H., Kawano, N., Yamauchi, Y., Shimada, E., Sasaki, R., Kubo, A., et al. (2004). Over−expression of ascorbate peroxidase in tobacco chloroplasts enhances the tolerance to salt stress and water deficit. Physiol. Plant. 121, 231–238. doi: 10.1111/j.0031-9317.2004.00308.x
Pubmed Abstract | Pubmed Full Text | CrossRef Full Text | Google Scholar
Barnes, J., Zheng, Y., and Lyons, T. (2002). “Plant resistance to ozone: the role of ascorbate,” in Air Pollution and Plant Biotechnology—Prospects for Phytomonitoring and Phytoremediation, eds K. Omasa, H. Saji, S. Youssefian, and N. Kondo (Tokyo: Springer-Verlag), 235–252.
Basu, S., Roychoudhury, A., Saha, P. P., and Sengupta, D. N. (2010a). Differential antioxidative responses of indica rice cultivars to drought stress. Plant Growth Regul. 60, 51–59. doi: 10.1007/s10725-009-9418-4
Basu, S., Roychoudhury, A., Saha, P. P., and Sengupta, D. N. (2010b). Comparative analysis of some biochemical responses of three indica rice varieties during polyethylene glycol-mediated water stress exhibits distinct varietal differences. Acta Physiol. Plant. 32, 551–563. doi: 10.1007/s11738-009-0432-y
Bhattacharjee, S. (2005). Reactive oxygen species and oxidative burst: roles in stress, senescence and signal. Curr. Sci. 89, 1113–1121.
Bienert, G. P., Møller, A. L., Kristiansen, K. A., Schulz, A., Møller, I. M., Schjoerring, J. K., et al. (2007). Specific aquaporins facilitate the diffusion of hydrogen peroxide across membranes. J. Biol. Chem. 282, 1183–1192. doi: 10.1074/jbc.M603761200
Pubmed Abstract | Pubmed Full Text | CrossRef Full Text | Google Scholar
Blokhina, O., and Fagerstedt, K. V. (2010). Reactive oxygen species and nitric oxide in plant mitochondria: origin and redundant regulatory systems. Physiol. Plant. 138, 447–462. doi: 10.1111/j.1399-3054.2009.01340.x
Pubmed Abstract | Pubmed Full Text | CrossRef Full Text | Google Scholar
Boguszewska, D., Grudkowska, M., and Zagdañska, B. (2010). Drought-responsive antioxidant enzymes in potato (Solanum tuberosum L.). Potato Res. 53, 373–382. doi: 10.1007/s11540-010-9178-6
Bright, J., Desikan, R., Hancock, J. T., Weir, I. S., and Neill, S. J. (2006). ABA−induced NO generation and stomatal closure in Arabidopsis are dependent on H2O2 synthesis. Plant J. 45, 113–122. doi: 10.1111/j.1365-313X.2005.02615.x
Pubmed Abstract | Pubmed Full Text | CrossRef Full Text | Google Scholar
Chang-Quan, W., and Rui-Chang, L. (2008). Enhancement of superoxide dismutase activity in the leaves of white clover (Trifolium repens L.) in response to polyethylene glycol-induced water stress. Acta Physiol. Plant. 30, 841–847. doi: 10.1007/s11738-008-0189-8
Chen, S., and Dickman, M. B. (2004). Bcl-2 family members localize to tobacco chloroplasts and inhibit programmed cell death induced by chloroplast-targeted herbicides. J. Exp. Bot. 55, 2617–2623. doi: 10.1093/jxb/erh275
Pubmed Abstract | Pubmed Full Text | CrossRef Full Text | Google Scholar
Chen, Z., and Gallie, D. R. (2006). Dehydroascorbate reductase affects leaf growth, development, and function. Plant Physiol. 142, 775–787. doi: 10.1104/pp.106.085506
Pubmed Abstract | Pubmed Full Text | CrossRef Full Text | Google Scholar
Choudhury, S., Panda, P., Sahoo, L., and Panda, S. K. (2013). Reactive oxygen species signaling in plants under abiotic stress. Plant Signal. Behav. 8:e23681. doi: 10.4161/psb.23681
Pubmed Abstract | Pubmed Full Text | CrossRef Full Text | Google Scholar
Chutipaijit, S., Cha-Um, S., and Sompornpailin, K. (2009). Differential accumulations of proline and flavonoids in indica rice varieties against salinity. Pak. J. Bot. 41, 2497–2506.
Dat, J., Vandenabeele, S., Vranová, E., Van Montagu, M., Inzé, D., and Van Breusegem, F. (2000). Dual action of the active oxygen species during plant stress responses. Cell. Mol. Life Sci. 57, 779–795. doi: 10.1007/s000180050041
Pubmed Abstract | Pubmed Full Text | CrossRef Full Text | Google Scholar
de Pinto, M. C., and De Gara, L. (2004). Changes in the ascorbate metabolism of apoplastic and symplastic spaces are associated with cell differentiation. J. Exp. Bot. 55, 2559–2569. doi: 10.1093/jxb/erh253
Pubmed Abstract | Pubmed Full Text | CrossRef Full Text | Google Scholar
Ellouzi, H., Hamed, K., Cela, J., Müller, M., Abdelly, C., and Munné-Bosch, S. (2013). Increased sensitivity to salt stress in tocopherol-deficient Arabidopsis mutants growing in a hydroponic system. Plant Signal. Behav. 8:e23136. doi: 10.4161/psb.23136
Pubmed Abstract | Pubmed Full Text | CrossRef Full Text | Google Scholar
Eltayeb, A. E., Kawano, N., Badawi, G. H., Kaminaka, H., Sanekata, T., Shibahara, T., et al. (2007). Overexpression of monodehydroascorbate reductase in transgenic tobacco confers enhanced tolerance to ozone, salt and polyethylene glycol stresses. Planta 225, 1255–1264. doi: 10.1007/s00425-006-0417-7
Evans, M. D., Dizdaroglu, M., and Cooke, M. S. (2004). Oxidative DNA damage and disease: induction, repair and significance. Mutat. Res. 567, 1–61. doi: 10.1016/j.mrrev.2003.11.001
Pubmed Abstract | Pubmed Full Text | CrossRef Full Text | Google Scholar
Eyidogan, F., and Öz, M. T. (2007). Effect of salinity on antioxidant responses of chickpea seedlings. Acta Physiol. Plant. 29, 485–493. doi: 10.1007/s11738-007-0059-9
Fini, A., Brunetti, C., Di Ferdinando, M., Ferrini, F., and Tattini, M. (2011). Stress-induced flavonoid biosynthesis and the antioxidant machinery of plants. Plant Signal. Behav. 6, 709–711. doi: 10.4161/psb.6.5.15069
Pubmed Abstract | Pubmed Full Text | CrossRef Full Text | Google Scholar
Foyer, C. H., and Noctor, G. (2005). Redox homeostasis and antioxidant signaling: a metabolic interface between stress perception and physiological responses. Plant Cell 17, 1866–1875. doi: 10.1105/tpc.105.033589
Pubmed Abstract | Pubmed Full Text | CrossRef Full Text | Google Scholar
Gapiñska, M., Skłodowska, M., and Gabara, B. (2008). Effect of short-and long-term salinity on the activities of antioxidative enzymes and lipid peroxidation in tomato roots. Acta Physiol. Plant. 30, 11–18. doi: 10.1007/s11738-007-0072-z
Gill, S. S., Khan, N. A., Anjum, N. A., and Tuteja, N. (2011). “Amelioration of cadmium stress in crop plants by nutrients management: morphological, physiological and biochemical aspects,” in Plant Nutrition and Abiotic Stress Tolerance III, Plant Stress 5 (Special Issue 1), eds N. A. Anjum and F. Lopez-Lauri, (Ikenobe: Global Science Books Ltd., UK), 1–23.
Gill, S. S., and Tuteja, N. (2010). Reactive oxygen species and antioxidant machinery in abiotic stress tolerance in crop plants. Plant Physiol. Biochem. 48, 909–930. doi: 10.1016/j.plaphy.2010.08.016
Pubmed Abstract | Pubmed Full Text | CrossRef Full Text | Google Scholar
Halliwell, B. (2006). Reactive species and antioxidants. Redox biology is a fundamental theme of aerobic life. Plant Physiol. 141, 312–322. doi: 10.1104/pp.106.077073
Pubmed Abstract | Pubmed Full Text | CrossRef Full Text | Google Scholar
Hasanuzzaman, M., Nahar, K., Alam, M. M., Roychowdhury, R., and Fujita, M. (2013). Physiological, biochemical, and molecular mechanisms of heat stress tolerance in plants. Int. J. Mol. Sci. 14, 9643–9684. doi: 10.3390/ijms14059643
Pubmed Abstract | Pubmed Full Text | CrossRef Full Text | Google Scholar
Hatz, S., Lambert, J. D., and Ogilby, P. R. (2007). Measuring the lifetime of singlet oxygen in a single cell: addressing the issue of cell viability. Photochem. Photobiol. Sci. 6, 1106–1116. doi: 10.1039/b707313e
Pubmed Abstract | Pubmed Full Text | CrossRef Full Text | Google Scholar
Higuchi, E. T. (2006). Look back over the studies of lignin biochemistry. J. Wood Sci. 52, 2–8. doi: 10.1007/s10086-005-0790-z
Ho, L. H., Giraud, E., Uggalla, V., Lister, R., Clifton, R., Glen, A., et al. (2008). Identification of regulatory pathways controlling gene expression of stress-responsive mitochondrial proteins in Arabidopsis. Plant Physiol. 147, 1858–1873. doi: 10.1104/pp.108.121384
Pubmed Abstract | Pubmed Full Text | CrossRef Full Text | Google Scholar
Holländer-Czytko, H., Grabowski, J., Sandorf, I., Weckermann, K., and Weiler, E. W. (2005). Tocopherol content and activities of tyrosine aminotransferase and cystine lyase in Arabidopsis under stress conditions. J. Plant Physiol. 162, 767–770. doi: 10.1016/j.jplph.2005.04.019
Pubmed Abstract | Pubmed Full Text | CrossRef Full Text | Google Scholar
Hu, X., Zhang, A., Zhang, J., and Jiang, M. (2006). Abscisic acid is a key inducer of hydrogen peroxide production in leaves of maize plants exposed to water stress. Plant Cell Physiol. 47, 1484–1495. doi: 10.1093/pcp/pcl014
Pubmed Abstract | Pubmed Full Text | CrossRef Full Text | Google Scholar
Igamberdiev, A. U., Seregelyes, C., Manac, N., and Hill, R. D. (2004). NADH-dependent metabolism of nitric oxide in alfalfa root cultures expressing barley hemoglobin. Planta 219, 95–102. doi: 10.1007/s00425-003-1192-3
Pubmed Abstract | Pubmed Full Text | CrossRef Full Text | Google Scholar
Jain, K., Kataria, S., and Guruprasad, K. N. (2003). Changes in antioxidant defenses of cucumber cotyledons in response to UV-B and to the free radicals generating compound AAPH. Plant Sci. 165, 551–557. doi: 10.1016/S0168-9452(03)00214-0
Jubany-Marí, T., Munné-Bosch, S., López-Carbonell, M., and Alegre, L. (2009). Hydrogen peroxide is involved in the acclimation of the Mediterranean shrub, Cistus albidus L., to summer drought. J. Exp. Bot. 60, 107–120. doi: 10.1093/jxb/ern274
Pubmed Abstract | Pubmed Full Text | CrossRef Full Text | Google Scholar
Kandziora-Ciupa, M., Ciepal, R., Nadgórska-Socha, A., and Barczyk, G. (2013). A comparative study of heavy metal accumulation and antioxidant responses in Vaccinium myrtillus L. leaves in polluted and non-polluted areas. Environ. Sci. Pollut. Res. 20, 4920–4932. doi: 10.1007/s11356-012-1461-4
Pubmed Abstract | Pubmed Full Text | CrossRef Full Text | Google Scholar
Karuppanapandian, T., Moon, J. C., Kim, C., Manoharan, K., and Kim, W. (2011). Reactive oxygen species in plants: their generation, signal transduction, and scavenging mechanisms. Aust. J. Crop Sci. 5, 709–725.
Kiffin, R., Bandyopadhyay, U., and Cuervo, A. M. (2006). Oxidative stress and autophagy. Antioxid. Redox Signal. 8, 152–162. doi: 10.1089/ars.2006.8.152
Pubmed Abstract | Pubmed Full Text | CrossRef Full Text | Google Scholar
Krieger-Liszkay, A., Fufezan, C., and Trebst, A. (2008). Singlet oxygen production in photosystem II and related protection mechanism. Photosyn. Res. 98, 551–564. doi: 10.1007/s11120-008-9349-3
Pubmed Abstract | Pubmed Full Text | CrossRef Full Text | Google Scholar
Kukreja, S., Nandwal, A. S., Kumar, N., Sharma, S. K., Unvi, V., and Sharma, P. K. (2005). Plant water status, H2O2 scavenging enzymes, ethylene evolution and membrane integrity of Cicer arietinum roots as affected by salinity. Biol. Plant. 49, 305–308. doi: 10.1007/s10535-005-5308-4
Kwak, J. M., Mori, I. C., Pei, Z. M., Leonhardt, N., Torres, M. A., Dangl, J. L., et al. (2003). NADPH oxidase AtrbohD and AtrbohF genes function in ROS−dependent ABA signaling in Arabidopsis. EMBO J. 22, 2623–2633. doi: 10.1093/emboj/cdg277
Pubmed Abstract | Pubmed Full Text | CrossRef Full Text | Google Scholar
Lee, K. P., Kim, C., Landgraf, F., and Apel, K. (2007). EXECUTER1-and EXECUTER2-dependent transfer of stress-related signals from the plastid to the nucleus of Arabidopsis thaliana. Proc. Natl. Acad. Sci. U.S.A. 104, 10270–10275. doi: 10.1073/pnas.0702061104
Pubmed Abstract | Pubmed Full Text | CrossRef Full Text | Google Scholar
Liu, X., Hua, X., Guo, J., Qi, D., Wang, L., Liu, Z., et al. (2008). Enhanced tolerance to drought stress in transgenic tobacco plants overexpressing VTE1 for increased tocopherol production from Arabidopsis thaliana. Biotechnol. Lett. 30, 1275–1280. doi: 10.1007/s10529-008-9672-y
Pubmed Abstract | Pubmed Full Text | CrossRef Full Text | Google Scholar
Luis, A., Sandalio, L. M., Corpas, F. J., Palma, J. M., and Barroso, J. B. (2006). Reactive oxygen species and reactive nitrogen species in peroxisomes. Production, scavenging, and role in cell signaling. Plant Physiol. 141, 330–335. doi: 10.1104/pp.106.078204
Pubmed Abstract | Pubmed Full Text | CrossRef Full Text | Google Scholar
Mafakheri, A., Siosemardeh, A., Bahramnejad, B., Struik, P. C., and Sohrabi, Y. (2010). Effect of drought stress on yield, proline and chlorophyll contents in three chickpea cultivars. Aust. J. Crop Sci. 4, 580–585.
Malar, S., Vikram, S. S., Favas, P. J. C., and Perumal, V. (2014). Lead heavy metal toxicity induced changes on growth and antioxidative enzymes level in water hyacinths [Eichhornia crassipes (Mart.)]. Bot. Stud. 55, 54. doi: 10.1186/s40529-014-0054-6
Malik, S., and Ashraf, M. (2012). Exogenous application of ascorbic acid stimulates growth and photosynthesis of wheat (Triticum aestivum L.) under drought. Soil Environ. 31, 72–77.
Mhamdi, A., Queval, G., Chaouch, S., Vanderauwera, S., Van Breusegem, F., and Noctor, G. (2010). Catalase function in plants: a focus on Arabidopsis mutants as stress-mimic models. J. Exp. Bot. 61, 4197–4220. doi: 10.1093/jxb/erq282
Pubmed Abstract | Pubmed Full Text | CrossRef Full Text | Google Scholar
Miller, G., Suzuki, N., Ciftci-Yilmaz, S., and Mittler, R. (2010). Reactive oxygen species homeostasis and signalling during drought and salinity stresses. Plant Cell Environ. 33, 453–467. doi: 10.1111/j.1365-3040.2009.02041.x
Pubmed Abstract | Pubmed Full Text | CrossRef Full Text | Google Scholar
Mittler, R. (2002). Oxidative stress, antioxidants and stress tolerance. Trends Plant Sci. 7, 405–410. doi: 10.1016/S1360-1385(02)02312-9
Pubmed Abstract | Pubmed Full Text | CrossRef Full Text | Google Scholar
Mittova, V., Tal, M., Volokita, M., and Guy, M. (2003). Up−regulation of the leaf mitochondrial and peroxisomal antioxidative systems in response to salt−induced oxidative stress in the wild salt−tolerant tomato species Lycopersicon pennellii. Plant Cell Environ. 26, 845–856. doi: 10.1046/j.1365-3040.2003.01016.x
Pubmed Abstract | Pubmed Full Text | CrossRef Full Text | Google Scholar
Møller, I. M., Jensen, P. E., and Hansson, A. (2007). Oxidative modifications to cellular components in plants. Annu. Rev. Plant Biol. 58, 459–481. doi: 10.1146/annurev.arplant.58.032806.103946
Pubmed Abstract | Pubmed Full Text | CrossRef Full Text | Google Scholar
Mullineaux, P. M., and Rausch, T. (2005). Glutathione, photosynthesis and the redox regulation of stress-responsive gene expression. Photosyn. Res. 86, 459–474. doi: 10.1007/s11120-005-8811-8
Pubmed Abstract | Pubmed Full Text | CrossRef Full Text | Google Scholar
Munné-Bosch, S., and Alegre, L. (2000). Changes in carotenoids, tocopherols and diterpenes during drought and recovery, and the biological significance of chlorophyll loss in Rosmarinus officinalis plants. Planta 210, 925–931. doi: 10.1007/s004250050699
Pubmed Abstract | Pubmed Full Text | CrossRef Full Text | Google Scholar
Murphy, M. (2009). How mitochondria produce reactive oxygen species. Biochem. J. 417, 1–13. doi: 10.1042/BJ20081386
Pubmed Abstract | Pubmed Full Text | CrossRef Full Text | Google Scholar
Navrot, N., Rouhier, N., Gelhaye, E., and Jacquot, J. P. (2007). Reactive oxygen species generation and antioxidant systems in plant mitochondria. Physiol. Plant. 129, 185–195. doi: 10.1111/j.1399-3054.2006.00777.x
Noctor, G., De Paepe, R., and Foyer, C. H. (2007). Mitochondrial redox biology and homeostasis in plants. Trends Plant Sci. 12, 125–134. doi: 10.1016/j.tplants.2007.01.005
Pubmed Abstract | Pubmed Full Text | CrossRef Full Text | Google Scholar
Noctor, G., Veljovic-Jovanovic, S., Driscoll, S., Novitskaya, L., and Foyer, C. H. (2002). Drought and oxidative load in the leaves of C3 plants: a predominant role for photorespiration? Ann. Bot. 89, 841–850. doi: 10.1093/aob/mcf096
Pubmed Abstract | Pubmed Full Text | CrossRef Full Text | Google Scholar
Oztetik, E. (2012). “An introduction to oxidative stress in plants and the role of non-enzymatic antioxidants,” in Oxidative Stress in Plants: Causes, Consequences and Tolerance, eds N. A. Anjum, S. Umar, and A. Ahmad (New Delhi: IK International Publishers), 1–50.
Palma, J. M., Corpas, F. J., and del Río, L. A. (2009). Proteome of plant peroxisomes: new perspectives on the role of these organelles in cell biology. Proteomics 9, 2301–2312. doi: 10.1002/pmic.200700732
Pubmed Abstract | Pubmed Full Text | CrossRef Full Text | Google Scholar
Pastore, D., Trono, D., Laus, M. N., Di Fonzo, N., and Flagella, Z. (2007). Possible plant mitochondria involvement in cell adaptation to drought stress a case study: durum wheat mitochondria. J. Exp. Bot. 58, 195–210. doi: 10.1093/jxb/erl273
Pubmed Abstract | Pubmed Full Text | CrossRef Full Text | Google Scholar
Peng, C. L., Ou, Z. Y., Liu, N., and Lin, G. Z. (2005). Response to high temperature in flag leaves of super high-yielding rice Pei'ai 64S/E32 and Liangyoupeijiu. Rice Sci. 12, 179–186.
Pfannschmidt, T. (2003). Chloroplast redox signals: how photosynthesis controls its own genes. Trends Plant Sci. 8, 33–41. doi: 10.1016/S1360-1385(02)00005-5
Pubmed Abstract | Pubmed Full Text | CrossRef Full Text | Google Scholar
Pinto, E., Sigaud-kutner, T., Leitao, M. A., Okamoto, O. K., Morse, D., and Colepicolo, P. (2003). Heavy metal-induced oxidative stress in algae. J. Phycol. 39, 1008–1018. doi: 10.1111/j.0022-3646.2003.02-193.x
Rao, M. V., Paliyath, G., and Ormrod, D. P. (1996). Ultraviolet-B and ozone-induced biochemical changes in antioxidant enzymes of Arabidopsis thaliana. Plant Physiol. 110, 125–136. doi: 10.1104/pp.110.1.125
Pubmed Abstract | Pubmed Full Text | CrossRef Full Text | Google Scholar
Rasmusson, A. G., Geisler, D. A., and Møller, I. M. (2008). The multiplicity of dehydrogenases in the electron transport chain of plant mitochondria. Mitochondrion 8, 47–60. doi: 10.1016/j.mito.2007.10.004
Pubmed Abstract | Pubmed Full Text | CrossRef Full Text | Google Scholar
Razaji, A., Asli, D. E., and Farzanian, M. (2012). The effects of seed priming with ascorbic acid on drought tolerance and some morphological and physiological characteristics of safflower (Carthamus tinctorius L.). Ann. Biol. Res. 3, 3984–3989.
Rhoads, D. M., Umbach, A. L., Subbaiah, C. C., and Siedow, J. N. (2006). Mitochondrial reactive oxygen species. Contribution to oxidative stress and interorganellar signaling. Plant Physiol. 141, 357–366. doi: 10.1104/pp.106.079129
Pubmed Abstract | Pubmed Full Text | CrossRef Full Text | Google Scholar
Roychoudhury, A., and Basu, S. (2012). “Ascorbate-Glutathione and plant tolerance to various abiotic stresses,” in Oxidative Stress in Plants: Causes, Consequences and Tolerance, eds N. A. Anjum, S. Umar, and A. Ahmad (New Delhi: IK International Publishers), 177–258.
Roychoudhury, A., Basu, S., Sarkar, S. N., and Sengupta, D. N. (2008). Comparative physiological and molecular responses of a common aromatic indica rice cultivar to high salinity with non-aromatic indica rice cultivars. Plant Cell Rep. 27, 1395–1410. doi: 10.1007/s00299-008-0556-3
Pubmed Abstract | Pubmed Full Text | CrossRef Full Text | Google Scholar
Roychoudhury, A., Basu, S., and Sengupta, D. N. (2012c). Antioxidants and stress-related metabolites in the seedlings of two indica rice varieties exposed to cadmium chloride toxicity. Acta Physiol. Plant. 34, 835–847. doi: 10.1007/s11738-011-0881-y
Roy Choudhury, A., Das, K., Ghosh, S., Mukherjee, R. N., and Banerjee, R. (2012b). Transgenic Plants: benefits and controversies. J. Bot. Soc. Bengal 66, 29–35.
Roychoudhury, A., and Ghosh, S. (2013). Physiological and biochemical responses of mungbean (Vigna radiata L. Wilczek) to varying concentrations of cadmium chloride or sodium chloride. Unique J. Pharm. Biol. Sci. 1, 11–21.
Roychoudhury, A., Pradhan, S., Chaudhuri, B., and Das, K. (2012a). “Phytoremediation of toxic metals and the involvement of Brassica species,” in Phytotechnologies: Remediation of Environmental Contaminants, eds N. A. Anjum, M. E. Pereira, I. Ahmad, A. C. Duarte, S. Umar, and N. A. Khan (Boca Raton, FL: CRC press; Taylor and Francis Group), 219–251.
Sairam, R. K., Deshmukh, P. S., and Saxena, D. C. (1998). Role of antioxidant systems in wheat genotypes tolerance to water stress. Biol. Plant. 41, 387–394. doi: 10.1023/A:1001898310321
Shalata, A., and Neumann, P. M. (2001). Exogenous ascorbic acid (vitamin C) increases resistance to salt stress and reduces lipid peroxidation. J. Exp. Bot. 52, 2207–2211. doi: 10.1093/jexbot/52.364.2207
Pubmed Abstract | Pubmed Full Text | CrossRef Full Text | Google Scholar
Shao, H. B., Liang, Z. S., Shao, M. A., and Sun, Q. (2005). Dynamic changes of anti-oxidative enzymes of 10 wheat genotypes at soil water deficits. Colloids Surf. B Biointerfaces 42, 187–195. doi: 10.1016/j.colsurfb.2005.02.007
Pubmed Abstract | Pubmed Full Text | CrossRef Full Text | Google Scholar
Sharma, P., and Dubey, R. S. (2004). Ascorbate peroxidase from rice seedlings: properties of enzyme isoforms, effects of stresses and protective roles of osmolytes. Plant Sci. 167, 541–550. doi: 10.1016/j.plantsci.2004.04.028
Sharma, P., and Dubey, R. S. (2005a). Drought induces oxidative stress and enhances the activities of antioxidant enzymes in growing rice seedlings. Plant Growth Regul. 46, 209–221. doi: 10.1007/s10725-005-0002-2
Sharma, P., and Dubey, R. S. (2005b). Modulation of nitrate reductase activity in rice seedlings under aluminium toxicity and water stress: role of osmolytes as enzyme protectant. J. Plant Physiol. 162, 854–864. doi: 10.1016/j.jplph.2004.09.011
Pubmed Abstract | Pubmed Full Text | CrossRef Full Text | Google Scholar
Sharma, P., Jha, A. B., Dubey, R. S., and Pessarakli, M. (2012). Reactive oxygen species, oxidative damage, and antioxidative defense mechanism in plants under stressful conditions. J. Bot. 2012:217037. doi: 10.1155/2012/217037
Simova-Stoilova, L., Vaseva, I., Grigorova, B., Demirevska, K., and Feller, U. (2010). Proteolytic activity and cysteine protease expression in wheat leaves under severe soil drought and recovery. Plant Physiol. Biochem. 48, 200–206. doi: 10.1016/j.plaphy.2009.11.003
Pubmed Abstract | Pubmed Full Text | CrossRef Full Text | Google Scholar
Smirnoff, N. (2000). Ascorbic acid: metabolism and functions of a multi-facetted molecule. Curr. Opin. Plant Biol. 3, 229–235. doi: 10.1016/S1369-5266(00)80070-9
Pubmed Abstract | Pubmed Full Text | CrossRef Full Text | Google Scholar
Sreedevi, S., and Krishnan, P. N. (2012). “Detoxification of heavy metal induced oxidative stress in plants, enzymatic and non-enzymatic machanisms- a mini review,” in Oxidative Stress in Plants: Causes, Cosequences and Tolerance, eds N. A. Ajum, S. Umar, and A. Ahmad (New Delhi: IK International Publishing House), 291–307.
Tanou, G., Molassiotis, A., and Diamantidis, G. (2009a). Hydrogen peroxide-and nitric oxide-induced systemic antioxidant prime-like activity under NaCl-stress and stress-free conditions in citrus plants. J. Plant Physiol. 166, 1904–1913. doi: 10.1016/j.jplph.2009.06.012
Pubmed Abstract | Pubmed Full Text | CrossRef Full Text | Google Scholar
Tanou, G., Molassiotis, A., and Diamantidis, G. (2009b). Induction of reactive oxygen species and necrotic death-like destruction in strawberry leaves by salinity. Environ. Exp. Bot. 65, 270–281. doi: 10.1016/j.envexpbot.2008.09.005
Triantaphylidès, C., Krischke, M., Hoeberichts, F. A., Ksas, B., Gresser, G., Havaux, M., et al. (2008). Singlet oxygen is the major reactive oxygen species involved in photooxidative damage to plants. Plant Physiol. 148, 960–968. doi: 10.1104/pp.108.125690
Pubmed Abstract | Pubmed Full Text | CrossRef Full Text | Google Scholar
Tseng, M. J., Liu, C. W., and Yiu, J. C. (2007). Enhanced tolerance to sulfur dioxide and salt stress of transgenic Chinese cabbage plants expressing both superoxide dismutase and catalase in chloroplasts. Plant Physiol. Biochem. 45, 822–833. doi: 10.1016/j.plaphy.2007.07.011
Pubmed Abstract | Pubmed Full Text | CrossRef Full Text | Google Scholar
Turrens, J. F. (2003). Mitochondrial formation of reactive oxygen species. J. Physiol. 552, 335–344. doi: 10.1113/jphysiol.2003.049478
Pubmed Abstract | Pubmed Full Text | CrossRef Full Text | Google Scholar
Ushimaru, T., Nakagawa, T., Fujioka, Y., Daicho, K., Naito, M., Yamauchi, Y., et al. (2006). Transgenic Arabidopsis plants expressing the rice dehydroascorbate reductase gene are resistant to salt stress. J. Plant Physiol. 163, 1179–1184. doi: 10.1016/j.jplph.2005.10.002
Pubmed Abstract | Pubmed Full Text | CrossRef Full Text | Google Scholar
Vaidyanathan, H., Sivakumar, P., Chakrabarty, R., and Thomas, G. (2003). Scavenging of reactive oxygen species in NaCl-stressed rice (Oryza sativa L.)—differential response in salt-tolerant and sensitive varieties. Plant Sci. 165, 1411–1418. doi: 10.1016/j.plantsci.2003.08.005
Verbruggen, N., and Hermans, C. (2008). Proline accumulation in plants: a review. Amino Acids 35, 753–759. doi: 10.1007/s00726-008-0061-6
Pubmed Abstract | Pubmed Full Text | CrossRef Full Text | Google Scholar
Wagner, D., Przybyla, D., op den Camp, R., Kim, C., Landgraf, F., Lee, K. P., et al. (2004). The genetic basis of singlet oxygen–induced stress responses of Arabidopsis thaliana. Science 306, 1183–1185. doi: 10.1126/science.1103178
Pubmed Abstract | Pubmed Full Text | CrossRef Full Text | Google Scholar
Wang, R., Liu, S., Zhou, F., Ding, C., and Hua, C. (2014). Exogenous ascorbic acid and glutathione alleviate oxidative stress induced by salt stress in the chloroplasts of Oryza sativa L. Z. Naturforsch. C 69, 226–236. doi: 10.5560/ZNC.2013-0117
Pubmed Abstract | Pubmed Full Text | CrossRef Full Text | Google Scholar
Wang, Y., Ying, Y., Chen, J., and Wang, X. (2004). Transgenic Arabidopsis overexpressing Mn-SOD enhanced salt-tolerance. Plant Sci. 167, 671–677. doi: 10.1016/j.plantsci.2004.03.032
Xu, C., Sullivan, J. H., Garrett, W. M., Caperna, T. J., and Natarajan, S. (2008). Impact of solar Ultraviolet-B on proteome in soybean lines differing in flavonoid contents. Phytochemistry 69, 38–48. doi: 10.1016/j.phytochem.2007.06.010
Pubmed Abstract | Pubmed Full Text | CrossRef Full Text | Google Scholar
Zlatev, Z. S., Lidon, F. C., Ramalho, J. C., and Yordanov, I. T. (2006). Comparison of resistance to drought of three bean cultivars. Biol. Plant. 50, 389–394. doi: 10.1007/s10535-006-0054-9
Pubmed Abstract | Pubmed Full Text | CrossRef Full Text | Google Scholar
Keywords: antioxidants, oxidative damages, reactive oxygen species, plant redox homeostasis, environmental stress
Citation: Das K and Roychoudhury A (2014) Reactive oxygen species (ROS) and response of antioxidants as ROS-scavengers during environmental stress in plants. Front. Environ. Sci. 2:53. doi: 10.3389/fenvs.2014.00053
Received: 08 October 2014; Accepted: 11 November 2014;
Published online: 02 December 2014.
Edited by:
Naser A. Anjum, University of Aveiro, PortugalReviewed by:
Naser A. Anjum, University of Aveiro, PortugalCarmen Arena, University of Naples Federico II, Italy
Sarvajeet Singh Gill, Maharshi Dayanand University, India
Copyright © 2014 Das and Roychoudhury. This is an open-access article distributed under the terms of the Creative Commons Attribution License (CC BY). The use, distribution or reproduction in other forums is permitted, provided the original author(s) or licensor are credited and that the original publication in this journal is cited, in accordance with accepted academic practice. No use, distribution or reproduction is permitted which does not comply with these terms.
*Correspondence: Aryadeep Roychoudhury, Post Graduate Department of Biotechnology, St. Xavier's College (Autonomous), 30, Mother Teresa Sarani, Kolkata - 700016, West Bengal, India e-mail:YXJ5YWRlZXAucmNAZ21haWwuY29t