- 1Centre of Advanced Study in Botany, Banaras Hindu University, Varanasi, India
- 2Department of Botany, Government Ramanuj Pratap Singhdev Post Graduate College, Sarguja University, Baikunthpur, India
- 3Ranjan Plant Physiology and Biochemistry Laboratory, Department of Botany, University of Allahabad, Allahabad, India
- 4D.D. Pant Interdisciplinary Research Laboratory, Department of Botany, University of Allahabad, Allahabad, India
Alleviation of abiotic stress by silicon (Si) is well documented. But mitigation of abiotic stress by silicon nanoparticles (SiNPs) is not much known. Therefore, hydroponic experiments were conducted to investigate if SiNPs are more effective than Si in mitigation of arsenate (AsV; 25 and 50 μM) toxicity in maize cultivar and hybrid differing in AsV tolerance. Under AsV stress, reduction in growth was accompanied by enhanced level of As and oxidative stress. AsV inhibited activities of antioxidant enzymes like ascorbate peroxidase, glutathione reductase, and dehydroascorbate reductase (except superoxide dismutase). The redox status of ascorbate and glutathione was disturbed by AsV as indicated by a steep decline in their reduced/oxidized ratios. However, addition of Si and SiNp ameliorates AsV toxicity in maize. Si and SiNp both could reduce AsV toxicity in maize cultivar and hybrid, which could be related with decreased accumulation of As and oxidative stress, and enhanced components of the ascorbate-glutathione cycle (AsA-GSH cycle). But lowering in the accumulation of As and oxidative stress markers, and enhancement in components of the AsA-GSH cycle was prominent in SiNp fed seedlings under AsV stress. The results also showed that SiNp are more effective in reducing AsV toxicity than Si, which is due to their greater availability to seedlings. Comparing responses of cultivar and hybrid, maize cultivar shows more resistance against AsV than hybrid.
Introduction
In last two decades, millions of people are exposed to arsenic (As) poisoning due to drinking of As-contaminated water (Nordstrom, 2002; Patel et al., 2005; Zhao et al., 2009; Singh R. et al., 2015; Singh et al., 2015a). Arsenic is found in different oxidation states, with the most dominant forms being trivalent (AsIII) and penta-valent (AsV), which are classified as potent carcinogens (Zhao et al., 2009). The potential sources of As in soil and water include both natural (e.g., weathering reactions, biological activity, volcanic emissions, etc.) and anthropogenic (mining, use of pesticides and wood preservatives, fossil fuels combustion, etc.; Patel et al., 2005; Zhao et al., 2009). Continuous increment in its concentration in the environment is one of the major public health problems all over the world. High As concentrations have been reported in different countries including, India, Bangladesh, China, Vietnam, Nicaragua, Brazil, France, USA, and several other countries (Patel et al., 2005; McClintock et al., 2012; Singh R. et al., 2015). In India, large area of water bodies and agricultural lands is highly polluted with As, being its concentrations between 50–300 mg L−1 and 32–105 mg kg−1, respectively (Patel et al., 2005; Singh et al., 2015a,b). The level of As in drinking water is also higher (0.3–0.5 mg L−1) than the prescribed limit (0.05 mg L−1; Singh et al., 2015b). Studies have demonstrated that As adversely influences growth, development and metabolic process of plants, and consequently yield (Li et al., 2006; Ding et al., 2009; Singh et al., 2015b).
The transportation of AsV into the plant cell takes place through phosphate transporters and thus, affects homeostasis of main source of energy i.e., ATP, while AsIII transportation occurs through aquaporins (nodulin26-like intrinsic protein family), and obstructs plant nutrient uptake, metabolism, and renovation of oxidative DNA damage (Kertulis-Tartar et al., 2009). There are increasing evidences, which show that As accelerates production of reactive oxygen species (ROS) such as superoxide radical () hydrogen peroxide (H2O2), and hydroxyl radicals (•OH), causing major damage to the plant cell. Several studies demonstrated that As influences photosynthetic pigments, membrane system of chloroplasts, chlorophyll fluorescence, and RUBISCO enzyme (Li et al., 2006; Ding et al., 2009; Singh et al., 2015a,b). Thus, As causes adverse effects on plants from molecular to whole plant level.
Silicon (Si) is recognized as second most abundant element on the earth's crust and also one of the most prominent mineral constituents of some plants (Epstein, 1999). In the soil, it comprises more than 25% of the earth's crust (Currie and Perry, 2007) and is available to plants in the form of monosilicic acid [Si(OH)4]. Since few decades, physiological role of Si in the regulation of abiotic and biotic stresses in plants has made huge interest among researchers (Savant et al., 1997; Currie and Perry, 2007). Several studies demonstrated that Si has numerous direct and indirect beneficial impacts on plant growth and development. For instance, it appreciably enhances resistance of plants to several toxic metals such as aluminum (Al), chromium (Cr), cadmium (Cd) iron (Fe), manganese (Mn), and zinc (Zn) (Horiguchi and Morita, 1987; Shi et al., 2005; Singh et al., 2011; Tripathi et al., 2012, 2015). There may be various strategies by which exogenous application of Si affects heavy metal resistance in plant species. It has been reported that Si supply could decrease the uptake of Mn (Horiguchi and Morita, 1987). However, Kidd et al. (2001) showed that Si stimulates maize root exudation of phenolics, which can chelate rhizosphere Al and thus, reduces its absorption. The valuable function of Si in resisting metal stress is well recognized in plants. However, an involvement of Si-nanoparticles (SiNp) in the regulation of metal stress is not yet well-known.
In last decade, nanotechnology has emerged as a prominent tool for enhancing agricultural productivity. Nanotechnology includes processing, generating, manipulating and deploying nanomaterials (having one or more proportions in the order of 100 nm or less). It has been documented that nanomaterials show size dependent behavior, distinctive optical properties and high surface area, thus exhibit significant role in plant protection and nutrition (Liu et al., 2009; Nair et al., 2010). In agro-nanotechnological research, efforts are being made to identify remedies for various stresses including heavy metals. Despite the availability of ample literature on metal stress, a relationship between metal stress and nanoparticle interaction, and nanoparticle-mediated alleviation of metal stress are still not well-known.
In past few decades, silicon (Si) has emerged as one of the beneficial elements that involved in the regulation of various abiotic stresses in plants. However, little is known about involvement of silicon nanoparticles (SiNp) in the regulation of metal toxicity in plants. Therefore, in the present study our aims were to: (1) investigate effect of Si and SiNp on maize cultivar and hybrid (2) confirm whether SiNp or Si are more effective in alleviating AsV toxicity in maize cultivar and hybrid (3) investigate the comparative impact of Si and nano-Si on antioxidant response of maize cultivar and hybrid under AsV stress and (4) elucidate possible mechanisms by which nano-Si alleviates AsV toxicity in maize seedlings.
Materials and Methods
Synthesis and Characterization of Silicon Nano-Particles (SiNp)
Silicon nano-particles (SiNp) were synthesized by sol-gel synthesis method using sodium silicate (Na2SiO3) according to the method of Liu et al. (2009) with some modifications. Briefly, Na2SiO3 was dissolved firstly in 475 mL distilled water (5 mM), and then the pH was adjusted to 3 with 1 mM HCl. After this, solution was stirred on water bath at 75⋅C for 2 h. The pH of the acidified Na2SiO3 was adjusted to 7.0 with NH4OH at 75⋅C and solution mixed thoroughly for about 1.5 h on water bath. The obtained Si sol was further dialyzed with dialysis bag to remove impurities such as Na+, , etc. and then evaporated to form the Si gel. The Si gel was further calcinated in furnace to obtain the SiNp powder. All solutions were freshly prepared at room temperature. The characterization of SiNp was performed by using scanning electron microscope (SEM), energy-dispersive X-ray spectroscopy (EDS), UV-Vis spectrophotometer, and X-ray diffraction (XRD) analysis.
XRD was performed on Rigaku D/max-2200 PC diffractometer operated at 40 kV/40 mA, using CuKα1 radiation with wavelength of 1.54 Å in the wide angle region from 20 to 80⋅ on 2θ scale. SEM was performed on Jeol JXA 8100 EPMA at 15 Kv and the UV-Vis spectrum was recorded by systronic double beam spectrophotometer. EDS was also performed for the detection of elemental analysis.
Plant Materials and Growth Conditions
Seeds of maize cultivar (Zea mays L. cv. Nootan) and hybrid (Z. mays L. hyb. Shaktiman-4) were purchased from certified supplier of local market. Seeds were surface sterilized in 10% (v/v) sodium hypochlorite solution for 5 min and then washed thoroughly and soaked for 2–4 h in distilled water. After sterilization and soaking, uniform sized seeds were sown in plastic trays containing sterilized sand. Thereafter, trays were kept in the dark for seed germination at 25 ± 2⋅C. When germination reached maximum, seedlings were grown in a growth chamber (CDR model GRW-300 DGe, Athens) under photosynthetically active radiation (PAR) of 350 μmol photons m−2 s−1 and 86–90% relative humidity with 16:8 h day-night regime at 26 ± 1⋅C for 15 days. During seedlings' growth, seedlings were sprayed with water whenever required. Uniformed sized seedlings were used to analyze the impact of Si and SiNp on various physiological and biochemical parameters of maize cultivar and hybrid under AsV stress.
SiNp, Si, and Asv Treatments
Uniform sized seedlings gently up rooted from sand and their roots were washed in tap water. Thereafter, seedlings were acclimatized in half strength Hoagland's nutrient solution for 24 h. After this, AsV, Si, and SiNp treatments were given. Sodium arsenate (Na2HAsO4. 7H2O) was used as a source of AsV while sodium silicate (Na2SiO3) was used as a source of Si and its nanoparticles. The AsV concentrations (25 and 50 μM) which have been used in the present study are environmentally relevant while selection of Si and SiNp dose (10 μM) was based on screening experiments. The treatments include: control (no added AsV, Si, and SiNp), Si (10 μM), SiNp (10 μM), 25 μM AsV, 25 μM AsV + 10 μM Si, 25 μM AsV + 10 μM SiNp, 50 μM AsV, 50 μM AsV +10 μM Si, and 50 μM AsV + 10 μM SiNp. In case of AsV + Si and AsV + SiNp treatments, seedlings were pre-treated with Si and SiNp for 24 h and then they were given AsV treatments. Just after AsV and Si and SiNp treatments, seedlings were further grown in a growth chamber for 7 days under 350 μmol photons m−2 s−1 of PAR and 86–90% relative humidity with 16:8 h day-night regime at 26 ± 1⋅C. During this growth period, medium of various treatments was changed twice and aerated daily to avoid root anoxia. After 7 days of AsV, Si, and SiNp treatments, seedlings were harvested and various parameters were analyzed immediately.
Estimation of Growth and Photosynthetic Pigments
Growth was measured in terms of root and shoots length and plant fresh weight. Seedlings were selected randomly from control and treated samples, divided into root and shoot and then their fresh weight and length were determined.
For the estimation of photosynthetic pigments, 20 mg fresh leaves from control and treated seedlings were crushed in 5 ml of 80% acetone and pigments were extracted and centrifuged. The absorbance of pigment extract was read at 663, 646, and 470 nm. The amount of chlorophyll a, chlorophyll b, and carotenoids was calculated following the method of Lichtenthaler (1987).
Estimation of as Content
For the determination of As content, dried root and shoot samples (50 mg) from control and treated seedlings were digested in mixed acid (HNO3:HClO4; 85:15, v/v) until transparent solution was obtained. The volume of digested sample was maintained up to 30 mL with double distilled water. The content of As in digested samples was estimated by atomic absorption spectrometer (iCE3000 Series, model-3500 AAS, Thermo scientific, UK), fitted with specific lamp of particular metal using appropriate drift blank.
Chlorophyll a Fluorescence Measurements
For the assessment of photosynthetic performance, chlorophyll a fluorescence measurements were taken in the dark adapted (30 min) leaves of control and treated seedlings using hand held leaf fluorometer (FluorPen FP 100, Photon System Instrument, Czech Republic). The following fluorescence parameters: variable/maximum fluorescence ratio (Fv/Fm), photochemical quenching (qP) and non-photochemical quenching (NPQ) were measured. Measurements were taken in second leaf of six different plants of each treatment (Strasser et al., 2000).
Estimation of Superoxide Radical and Hydrogen Peroxide
Superoxide radicals (SOR; ) in control and treated seedlings were determined following the method of Elstner and Heupel (1976). This assay is based on formation of from hydroxylamine in the presence of . Fresh leaves (50 mg) were crushed in 65 mM potassium phosphate buffer (pH 7.8) and centrifuged at 10,000 g for 10 min at 4⋅C. The reaction mixture consisted of 65 mM potassium phosphate buffer (pH 7.8), 10 mM hydroxylamine hydrochloride and leaf extract and then incubated for 20 min at 25⋅C. After this, 17 mM sulfanilamide and 7 mM naphthylethylene diamine dihydrochloride were mixed to the incubated reaction mixture. After 15 min of reaction, diethyl ether was mixed to the same reaction mixture gently and centrifuged at 2000 g for 5 min. The absorbance of the colored aqueous phase was recorded at 530 nm. A standard curve was prepared with NaNO2 and used to calculate the production of . The production of is equivalent to generation of .
For the estimation of H2O2, fresh leaf samples (50 mg) from control and treated seedlings were crushed in 0.1% (w/v) trichloroacetic acid (Velikova et al., 2000). The reaction mixture (2 ml) contained tissue extract (0.5 ml), 10 mM potassium phosphate buffer (pH 7.0), and 1 M KI solution. Absorbance of reaction mixture was recorded at 390 nm to read increase in turbidity. Hydrogen peroxide concentration was calculated by using a standard curve prepared with H2O2.
Estimation of Lipid Peroxidation
Lipid peroxidation as malondialdehyde (MDA) content was estimated according to the method of Heath and Packer (1968). Fresh leaves (50 mg) from control and treated seedlings were crushed in 5% (w/v) TCA and centrifuged at 10,000 g for 10 min at 4⋅C. Reaction mixture contained thiobarbituric acid (0.5%)-TCA (20%) solution and tissue extract was boiled for 20 min in water bath. After cooling, the absorbance of reaction mixture (TBA-MDA) was recorded at 532 and 600 nm. The values of non-specific absorption recorded at 600 nm were subtracted from the values recorded at 532 nm. The content of MDA was determined by using an extinction coefficient of 155 mM−1 cm−1.
Estimation of SOD, APX, GR, and DHAR Activities
Superoxide dismutase (SOD; EC 1.15.1.1) activity was measured according to the method of Giannopolitis and Reis (1977). Fresh leaves (50 mg) from control and treated seedlings were homogenized in EDTA-phosphate buffer (50 mM; pH 7.8) and centrifuged at 15,000 g for 20 min at 4⋅C and supernatant was used as enzyme extract. Reaction mixture (3 ml) contained 50 mM potassium phosphate buffer (pH 7.8), 1.3 μM riboflavin, 0.1 mM EDTA, 13 mM methionine, 63 μM nitroblue tetrazolium (NBT), 0.05 M sodium carbonate (pH 10.2), and enzyme extract (0.1 ml). The reaction mixtures were illuminated for 20 min under white light intensity of 100 μmol photons m−2 s−1. The photoreduction of NBT (formation of purple formazone) was recorded spectrophotometrically at 560 nm and compared with blank samples having no enzyme extract. One unit (U) of SOD activity is defined as the amount of enzyme required to cause 50% inhibition in reduction of NBT.
Ascorbate peroxidase (APX; EC 1.11.1.11) activity was determined according to the method of Nakano and Asada (1981). Fresh leaves (50 mg) from control and treated seedlings were homogenized in 50 mM potassium phosphate buffer (pH 7.0) containing 1 mM EDTA, centrifuged at 15,000 g for 20 min at 4⋅C and supernatant was used as enzyme. Reaction mixture contained 50 mM potassium phosphate buffer (pH 7.0) containing 1 mM EDTA, 0.5 mM ascorbate, 0.1 mM H2O2, and enzyme extract. The decrease in absorbance due to H2O2 decomposition was measured at 290 nm. The enzyme activity was calculated by using an extinction coefficient of 2.8 mM−1cm−1. One unit (U) of enzyme activity is defined as 1 nmol ascorbate oxidized min−1.
Glutathione reductase (GR; EC 1.6.4.2) activity was assayed according to the method of Schaedle and Bassham (1977). The decrease in absorbance due to NADPH oxidation was read at 340 nm, and GR activity was calculated using an extinction coefficient of 6.2 mM−1 cm−1. One unit (U) of enzyme activity is defined as 1 nmol NADPH oxidized min−1.
Dehydroascorbate reductase (DHAR; EC 1.8.5.1) activity was assayed by the method of Nakano and Asada (1981). An increase in absorbance due reduced ascorbate formation was read at 265 nm, and DHAR activity was calculated using an extinction coefficient of 7.0 mM−1 cm−1. One unit (U) of enzyme activity is defined as 1 nmol DHA reduced min−1.
Determination of Ascorbate and Glutathione
Total ascorbate (AsA+DHA), reduced ascorbate (AsA), and dehydroascorbate (DHA) were determined by the method of Gossett et al. (1994). This assay is based on the reduction of Fe3+ to Fe2+ with ascorbic acid in acidic solution followed by formation of red chelate between Fe2+ and 2,2-bipyridyl. Ascorbate content was calculated using a standard curve prepared with L-ascorbic acid.
Total (GSH+GSSG), reduced (GSH) and oxidized glutathione (GSSG) contents were estimated by the enzyme recycling method of Brehe and Burch (1976). This method is based on sequential oxidation of GSH by 5, 5-dithiobis-2-nitrobenzoic acid (DTNB) and reduction of GSSG in the presence of NADPH and glutathione reductase (GR; Type III from bakers' yeast; Sigma Chemical Company). The amount of glutathione was determined by using a standard curve prepared with GSH.
Statistical Analysis
Results were statistically analyzed by analysis of variance (ANOVA). Duncan's multiple range test was applied for mean separation for significant differences among treatments at P < 0.05 significance level. The results presented are the means ± standard error of six replicates (n = 6).
Results
Characterization of SiNp
The morphology of synthesized SiNp was investigated by SEM, which shows almost spherical nanoparticles with size ranges from 150 to 200 nm (Figure 1A). The Figure 1B demonstrated the size distributions of SiNps with an average diameter of 170 ± 10 nm, calculated by measuring more than 100 particles in SEM images. The XRD pattern of the synthesized SiNp is shown in Figure 1C, which appears as broad band's with expression at 2″ = 22.8⋅ representing that synthesized material is amorphous and composed of SiO2 (Sooksaen et al., 2008). Elemental analysis of the sample using energy-dispersive energy-dispersive X-ray spectroscopy (EDS) is shown in Figure 1D. The analysis clearly indicates presence of silicon, carbon and oxygen in SiNPs. The UV-Vis spectroscopic analysis of synthesized SiNp was carried out in the range of 320–800 nm and shown in Figure 1E. The UV-Vis spectrum of SiNp clearly shows the absorption peak around ~445 nm (Wilder et al., 1998).
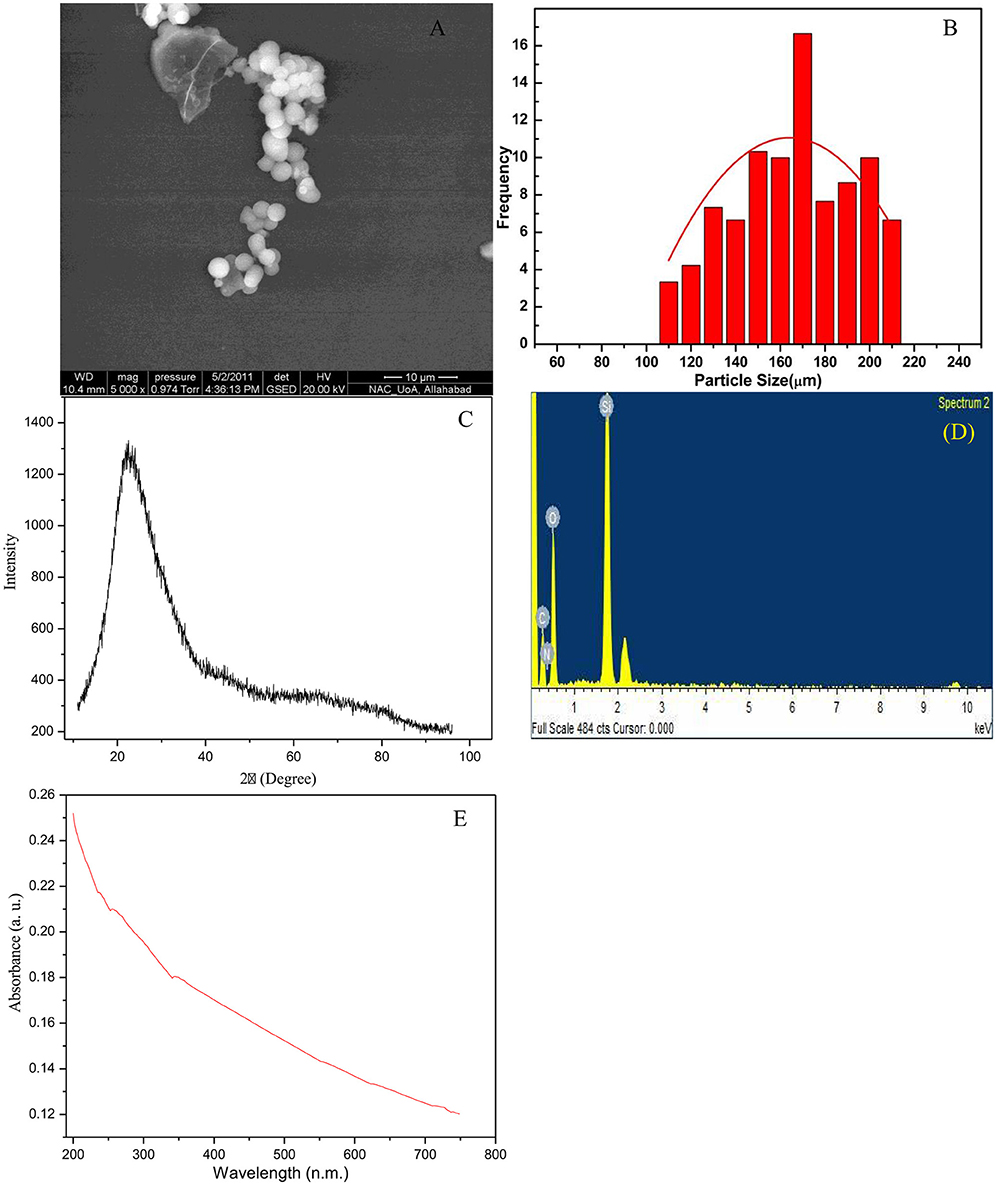
Figure 1. Characterization of silicon nano-particles (SiNp) by various techniques. SEM micrograph (A), demonstration of the size distributions of SiNps with an average diameter of 170 ± 10 nm, calculated by measuring more than 100 particles in SEM images (B), XRD pattern of synthesized silicon nanoparticles (C), X-ray spectroscopy (EDS) (D), and UV-Vis absorption spectrum (E).
Impact of Si and SiNp on Growth and As Accumulation under Asv Stress
Growth measured in terms of fresh weight was reduced significantly (P < 0.05) following AsV treatments in both maize cultivar and hybrid (Figure 2). Treatment with 25 and 50 μM of AsV reduced growth by 14 and 29% in maize cultivar while 19 and 34% in hybrid, respectively compared to the control. On the other hand, Si addition along with AsV treatments (25 and 50 μM) alleviated AsV-induced reduction in growth of maize cultivar and hybrid as percentage decline was only 7 and 19% and 8 and 22%, respectively. However, the addition of Si alone enhanced fresh mass of maize cultivar and hybrid by 6 and 7%, respectively over the value of control. Besides this, addition of SiNp along with 25 and 50 μM of AsV also significantly (P < 0.05) alleviated AsV-induced reduction in growth of maize cultivar and hybrid as reductions were only 4 and 12% and 5 and 16%, respectively. Thus, data indicate that SiNp were more effective in reducing (P < 0.05) AsV toxicity than Si. Furthermore, the addition of SiNp alone significantly increased fresh mass of maize cultivar and hybrid by 10 and 9%, respectively over the respective value of control (Figure 2).
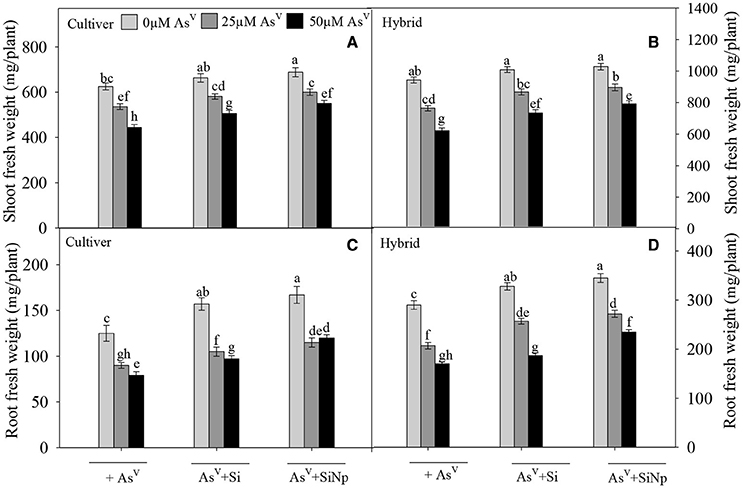
Figure 2. Effects of Si and silicon nanoparticles (SiNp) on growth (fresh weight of roots and shoots), of maize cultivar (A,C) and hybrid exposed (B,D) to AsV stress. Data are means ± standard error of three independent experiments with two replicates in each experiment (n = 6). Bars followed by different letter(s) show significant difference at P < 0.05 significance level according to the Duncan's multiple range test.
Similar to the AsV-induced reduction in growth, the accumulation of As was significantly (P < 0.05) higher in AsV-stressed maize cultivar and hybrid (Figure 3). Maize cultivar and hybrid seedlings grown under 25 and 50 μM of AsV treatments accumulated about 124 and 272 μg As g−1 dry weight and 156 and 312 μg As g−1 dry weight, respectively. However, with the addition of Si (25 μM AsV + Si and 50 μM AsV + Si) metal accumulation significantly reduced and it was only 110 and 226 μg As g−1 dry weight and 136 and 246 μg As g−1 dry weight in maize cultivar and hybrid seedlings, respectively (Figure 3). Similarly, the addition of SiNp with 25 and 50 μM of AsV also significantly (P < 0.05) reduced the accumulation of As in maize cultivar and hybrid as accumulation was only 104 and 212 μg As g−1 dry weight and 126 and 234 μg As g−1 dry weight, respectively.
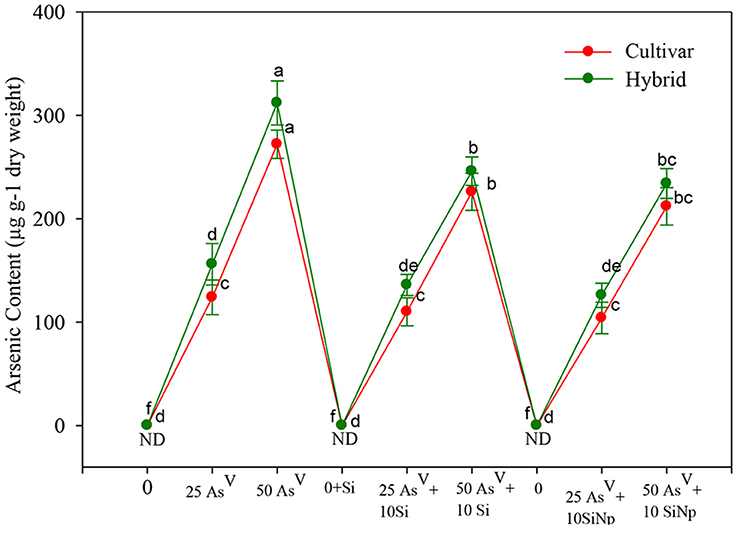
Figure 3. Effects of Si and silicon nanoparticles (SiNp) on the accumulation of As in maize cultivar and hybrid exposed to AsV stress. Data are means ± standard error of three independent experiments with two replicates in each experiment (n = 6). Bars followed by different letter(s) show significant difference at P < 0.05 significance level according to the Duncan's multiple range test.
Impact of Si and SiNp on Photosynthetic Pigments under Asv Stress
Data pertaining to the photosynthetic pigments i.e., total chlorophyll, carotenoids, and protein are presented in Table 1. The results reveal that AsV (25 and 50 μM) treatments significantly (P < 0.05) declined chlorophyll contents of maize cultivar by 8 and 16% while in hybrid it was declined by 11 and 19%, respectively. Similar to the results of chlorophyll content, carotenoids, and total protein were also significantly decreased in maize cultivar and hybrid. Under 25 and 50 μM of AsV treatments, carotenoids and protein were decreased by 6 and 13% and 16 and 24% in cultivar and by 9 and 16% and 18 and 27%, respectively in hybrid (Table 1). On the other hand, the addition of Si and SiNp in nutrient medium alleviated (P < 0.05) AsV-induced decline in total chlorophyll, carotenoids and total protein in comparison to the AsV treatments alone (Table 1).
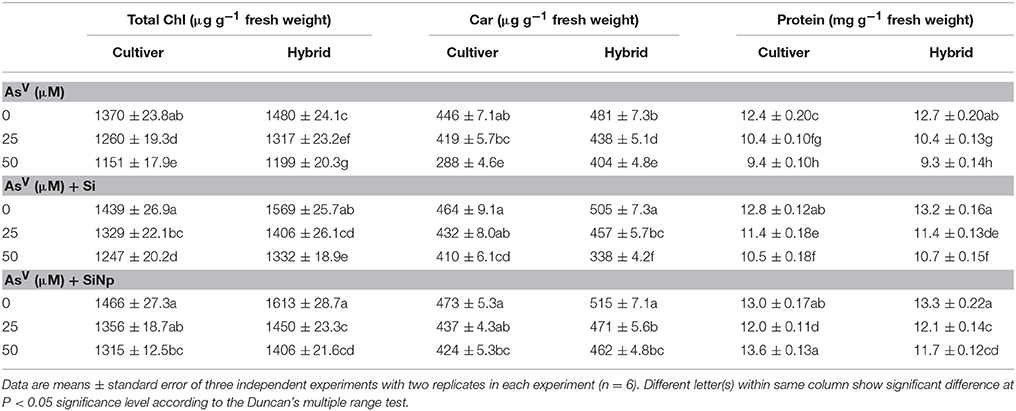
Table 1. Effects of Si and silicon nanoparticles (SiNp) on total chlorophyll (total Chl), carotenoids (Car) and protein content of maize cultivar and hybrid exposed to AsV stress.
Impact of Si and SiNp on Photosynthetic Performance under Asv Stress
The results pertaining to changes in chlorophyll a fluorescence parameters such as Fv/Fm, qP, and NPQ are shown in Figures 4A–F. The results reveal that AsV (25 and 50 μM) exposure decreased Fv/Fm and qP by 11 and 19% and 14 and 21% in maize cultivar while by 14 and 22% and 16 and 24% in hybrid, respectively as compared to the control (Figures 4A–D). In contrast to this, treatment of plants with AsV at 25 and 50 μM increased NPQ by 18 and 25% in maize cultivar and by 21 and 28%, respectively in hybrid (Figures 4E,F). On the other hand, Si and SiNP treatments alone did not bring significant (P < 0.05) changes in chlorophyll a fluorescence parameters. Furthermore, the addition of Si and SiNp with AsV significantly (P < 0.05) ameliorated AsV-induced negative impacts on Fv/Fm, qP, and NPQ in comparison to the AsV treatments alone (Figure 4).
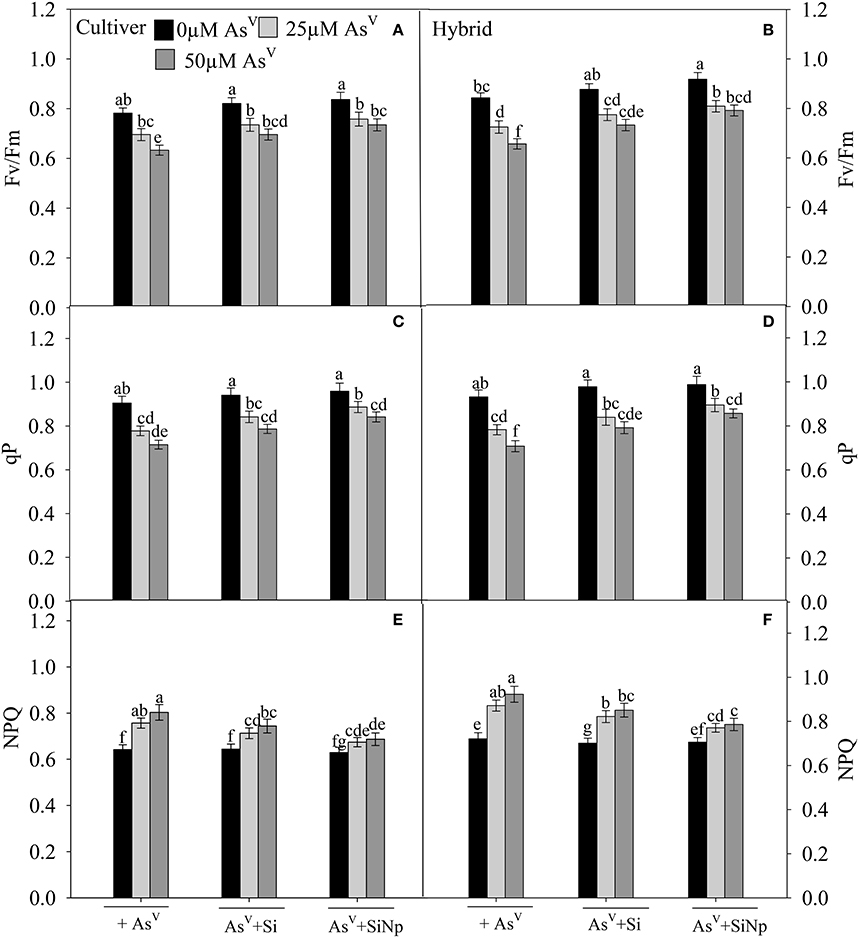
Figure 4. Effects of Si and silicon nanoparticles (SiNp) on maximum photochemical efficiency of PS II (Fv/Fm) (A,B), photochemical quenching (qP) (C,D) and non-photochemical quenching (NPQ) (E,F) in maize cultivar and hybrid exposed to AsV stress. Data are means ± standard error of three independent experiments with two replicates in each experiment (n = 6). Bars followed by different letter(s) show significant difference at P < 0.05 significance level according to the Duncan's multiple range test.
Impact of Si and SiNp on Reactive Oxygen Species and Lipid Peroxidation under Asv Stress
It has been reported in earlier studies that AsV stress was associated with the production of ROS, which ultimately cause damage to macromolecules. Therefore, in order to investigate oxidative stress in both maize cultivar and hybrid, we examined levels of SOR and H2O2 in plants whose growth was suppressed substantially by AsV (25 and 50 μM). The results show that 25 and 50 μM of AsV enhanced SOR and H2O2 content by 18 and 27% and 24 and 32% in maize cultivar and by 23 and 32% and 28 and 37%, respectively in hybrid (Figures 5A–D). Contrary to AsV, Si, and SiNp treatments alone did not significantly (P < 0.05) change SOR and H2O2 contents in maize cultivar and hybrid (Figures 5A–D). Further, when Si and SiNp was added jointly with AsV, they significantly (P < 0.05) reduced AsV-mediated enhancement in SOR and H2O2. Under 25 μM AsV +Si, 50 μM AsV +Si, 25 μM AsV +SiNp, and 50 μM AsV +SiNp combinations, SOR declined up to 11 and 17%, 6 and 10% in cultivar and 15 and 20% and 7 and 11% in hybrid while H2O2 decreased up to 14 and 21% and 6 and 11% in cultivar and 18 and 24% and 9 and 14%, respectively in hybrid (Figures 5A–D).
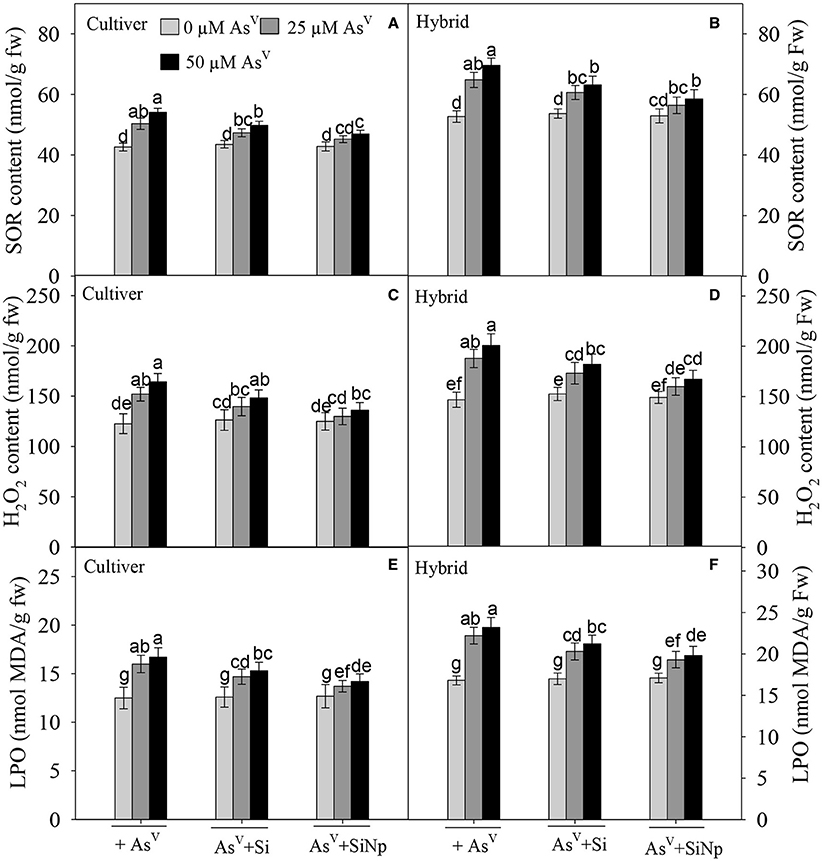
Figure 5. Effects of Si and silicon nanoparticles (SiNp) on superoxide radicals (SOR) (A,B), hydrogen peroxide (H2O2) (C,D) and lipid peroxidation (as MDA, malondialdehyde) (E,F) in maize cultivar and hybrid exposed to AsV stress. Data are means ± standard error of three independent experiments with two replicates in each experiment (n = 6). Bars followed by different letter(s) show significant difference at P < 0.05 significance level according to the Duncan's multiple range test.
Lipid peroxidation is a marker to measure the effect of abiotic stresses such as AsV stress on lipids. Accordingly, 25 and 50 μM of AsV also enhanced lipid peroxidation (MDA content) by 28 and 34% in maize cultivar and by 32 and 38%, respectively in hybrid (Figures 5E,F). On the other hand, the addition of Si and SiNp alone did not influence the production of MDA in maize cultivar and hybrid. Besides this, they significantly (P < 0.05) lowered AsV-induced lipid peroxidation in maize cultivar and hybrid as MDA content declined up to 18 and 10% in cultivar and 21 and 15% in hybrid under 25 μM AsV +Si and 25 μM AsV +SiNp combinations and 24 and 14% in cultivar and 26 and 18% in hybrid, respectively, under 50 μM AsV +Si and 50 μM AsV + SiNp combination (Figures 5E,F).
Impact of Si and SiNp on the Activities of Enzymatic Antioxidants Under Asv Stress
In order to investigate the role of Si and SiNp in the regulation of antioxidant enzymes and the relationship between ROS and the cellular defense system, the activities of SOD, APX, GR, and DHAR were examined in AsV-stressed maize cultivar and hybrid with and without Si and SiNp supplementation. The results related to the activities of enzymatic antioxidants are shown in Table 2. The results show that AsV treatments (25 and 50 μM) significantly (P < 0.05) increased SOD activity in maize cultivar and hybrid. In contrast to SOD activity, 25 and 50 μM of AsV significantly (P < 0.05) inhibited the activities of APX (24 and 31% and 31 and 38%), GR (28 and 34% and 32 and 39%), and DHAR (34 and 39% and 38 and 44%) in maize cultivar and hybrid, respectively as compared to the value of control (Table 2). In contrast to this, Si and SiNp alone significantly (P < 0.05) increased the activity of all enzymes studied in maize cultivar and hybrid. Moreover, the addition of Si and SiNp with AsV significantly alleviated AsV-mediated inhibitions in APX, GR, and DHAR (Table 2).
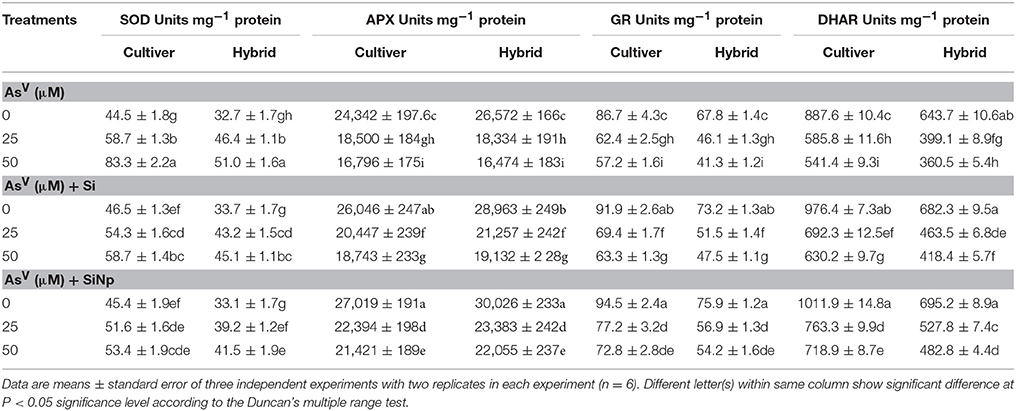
Table 2. Effects of Si and silicon nanoparticles (SiNp) on the activities of superoxide dismutase (SOD), ascorbate peroxidase (APX), glutathione reductase (GR), and dehydroascorbate reductase (DHAR) in maize cultivar and hybrid exposed to AsV stress.
Impact of Si and SiNp on Ascorbate and Glutathione Levels under Asv Stress
The results related to amounts of ascorbate and glutathione and their redox status are shown in Tables 3, 4. Under AsV (25 and 50 μM) treatments, though total ascorbate increased by 14 and 25% in cultivar and 13 and 21% in hybrid and total glutathione content enhanced by 17 and 29% in cultivar and 12 and 24%, respectively in hybrid, however, their oxidized forms increased (p < 0.05) by 154 and 263% in cultivar and 168 and 199% in hybrid and 166 and 371% in cultivar and 175 and 350%, respectively in hybrid (Tables 3, 4). This results into a steep decline in reduced ascorbate/dehydroascorbate and reduced glutathione/oxidized glutathione ratios as they were only 6.8 and 4.2 in cultivar and 4.6 and 3.8 in hybrid and 5.4 and 3.4 in cultivar and 4.6 and 2.8 in hybrid, respectively following AsV (25 and 50 μM) exposure (Tables 3, 4). On the other hand, Si and SiNp alone enhanced levels of reduced ascorbate and glutathione as well as their reduced/oxidized ratios in maize cultivar and hybrid (Tables 3, 4). Furthermore, application of Si and SiNp together with AsV (25 and 50 μM) attenuated AsV-induced diminish in levels of reduced ascorbate and glutathione as well as their reduced/oxidized ratios in both maize cultivar and hybrid seedlings (Tables 3, 4).
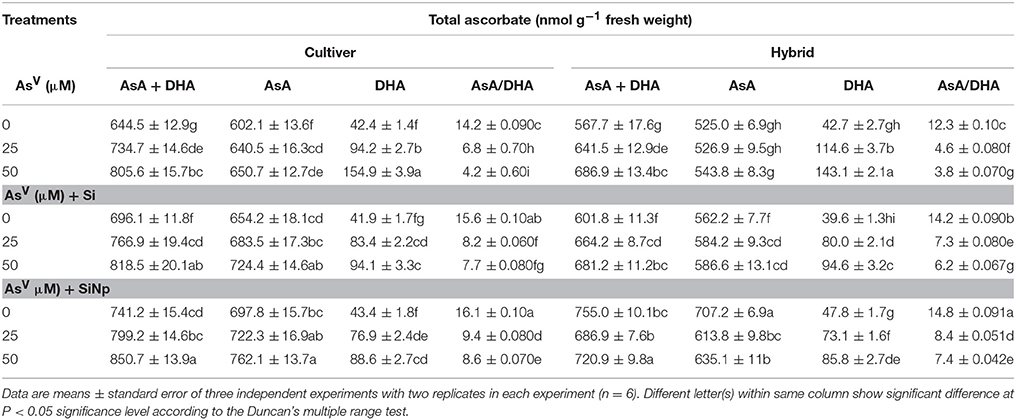
Table 3. Effects of Si and silicon nanoparticles (SiNp) on total ascorbate (AsA+DHA), reduced ascorbate (AsA), dehydroascorbate (DHA), and reduced ascorbate/dehydroascorbate ratio (AsA/DHA) in maize cultivar and hybrid exposed to AsV stress.
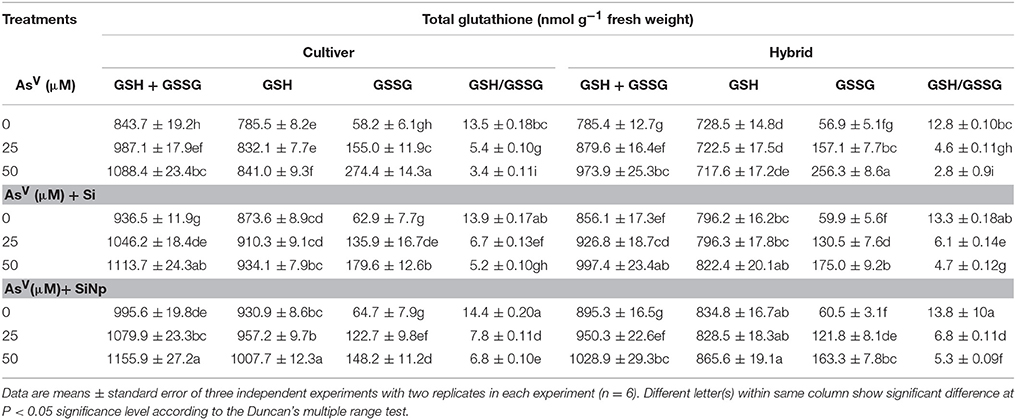
Table 4. Effects of Si and silicon nanoparticles (SiNp) on total glutathione (GSH+GSSG), reduced glutathione (GSH), oxidized glutathione (GSSG) and reduced glutathione/oxidized glutathione ratio (GSH/GSSG) in maize cultivar and hybrid exposed to AsV stress.
Discussion
Similar to previous studies, the results of the present study revealed that AsV significantly (P < 0.05) reduced growth of maize cultivar and hybrid seedlings, which has occurred due to the enhancement in the accumulation of As in seedlings (Figures 2, 3). This kind of AsV toxicity response is well-documented (Li et al., 2006; Singh R. et al., 2015; Singh et al., 2015a,b). Additionally, the results revealed that AsV negatively affects pigments, total protein, photosynthesis, and the status of antioxidants (SOD, APX, GR, and DHAR, reduced ascorbate and glutathione), and significantly increased level of oxidative stress markers like SOR, H2O2, and damage to lipids (Figures 4, 5; Tables 1–4). To explain AsV-induced toxicity in plants, numerous hypotheses, and mechanisms have been proposed but in the present investigation AsV-mediated toxicity to maize cultivar and hybrid seedlings may be associated with its harmful impacts on pigments, photosynthesis and macromolecules such as lipids due to higher accumulation of As.
On contrary, the addition of Si and SiNp significantly (P < 0.05) reduced toxicity level of AsV in maize cultivar and hybrid by ameliorating AsV-induced negative effects on growth, pigments, photosynthetic process and protein, which was accompanied by a decreased level of As accumulation (Figure 3; Table 1). Si-mediated alleviation of AsV toxicity complies with mechanisms, which were reported in earlier studies (Horiguchi and Morita, 1987; Tripathi et al., 2015; Wang et al., 2015). Regarding SiNp-mediated alleviation of AsV toxicity, somewhat similar mechanisms have been observed as reported for Si. For instance, lowering in the accumulation of As and oxidative damage and enhancement in antioxidant defense system (Figures 3, 5; Tables 2–4). In recent years, nanotechnology has been established as a powerful technology and shown a greater potential as an environment cleaner technology including alleviation of toxicities of various heavy metals (Dickinson and Scott, 2010). Further various studies also demonstrated metal nanoparticles mediated alleviation of metal toxicity in plants. (Dubchak et al., 2010; Wang et al., 2012). However, the role of nanoparticles and their mechanisms of action in plant growth and development is not yet well known. But their high surface to volume ratio that increases their reactivity and possible biochemical activity of nanoparticles might play a role in their action (Adrees et al., 2015). Considering greater potential of SiNp in alleviating AsV stress than Si, it is reported that SiNp have greater availability to seedlings than Si (unpublished data), thus exerting greater ameliorative effect in maize cultivar and hybrid.
At the present, several mechanisms are known by which Si alleviates abiotic stresses such as heavy metal toxicities, salinity, drought, chilling and freezing stresses. However, several other routes of Si action in mitigation of metal toxicity remain to be investigated. Some mechanisms, which are frequently discussed in the literature regarding Si-mediated alleviation of heavy metal stresses comprise: (1) stimulation of antioxidant systems, (2) complexation or co-precipitation of toxic metal ions with Si, (3) immobilization of toxic metal ions in growth media, (4) uptake processes, (5) compartmentation of metal ions within plants, and (6) Si may modify the cation binding capacity of the cell wall (Kopittke et al., 2012). The results of the present study revealed that the addition of SiNp reduced As accumulation in maize cultivar and hybrid seedlings, which resulted into better photosynthetic performance, decreased levels of oxidative stress markers (ROS, H2O2, and MDA) and improved antioxidant defense system (Figures 2–5; Tables 1–4). The reduction in As accumulation may be described on the basis of formation of barrier in root endodermis for AsV as reported in other studies (Feng et al., 2009; Singh et al., 2013). Moreover, the addition of SiNp reduced membrane lipid peroxidation caused by excess metal and significantly improved cell wall breadth in root epidermis (data not shown), and thus declining As absorption and accumulation. It is reported that nano silica is easily absorbed by plants than inorganic silica (Suriyaprabha et al., 2012), and thus justifying greater protective impact of SiNp than Si under AsV stress in maize seedlings.
Application of Si and SiNp lowered the level of oxidative stress. It is further proven by increased activity of superoxide dismutase (SOD). SOD comprises the first line of defense system against ROS which catalyzes dismutation of superoxide anion into H2O2 and O2 in chloroplast. Significant enhancement of SOD has been reported in an earlier study (Kuniak and Skodowska, 2001). In the present study, the activity of SOD in cultivar and hybrid maize treated with AsV and Si and SiNp together showed enhanced activity, which suggested the important role of SOD in scavenging ROS. However, application of Si and SiNp decreased SOD activity in cultivar and hybrid maize (Table 2). These results imply that it might be due to lower accumulation of superoxide anion in cultivar and hybrid maize seedlings. It has been well documented that the ascorbate-glutathione (AsA-GSH cycle) cycle is the main mechanism which scavenges ROS successfully in plants under abiotic and biotic stresses (Schmidt et al., 1999; Liang et al., 2003; Asada, 2006). The activity of APX eliminates H2O2. Moreover, GR and DHAR both are principally accountable for providing substrate (reduced glutathione and ascorbate, respectively) for APX during scavenging of H2O2. The present study indicated that APX, DHAR, and GR were inhibited by excess AsV, and (Table 2) in accordance with the change of their activities in barley and bentgrass (Liang et al., 2003; Mittler et al., 2004; Foyer and Noctor, 2011). However, application of Si and SiNp enhanced their activities under AsV stress demonstrating that rising AsA-GSH cycle components is one of key mechanisms to counteract AsV stress in maize cultivar and hybrid as evidenced from data of oxidative stress (Table 2).
Furthermore, ascorbate and glutathione are recognized as the heart of the redox hub. Both antioxidants are abundant and stable with proper redox potentials that act together in several pathways and maintained in a commonly reduced condition (Foyer and Noctor, 2011). Mittler et al. (2004) demonstrated that both antioxidants are the element of an extremely complex and complicated plant antioxidative system. In the present study, oxidative stress caused by AsV in maize seedlings and defense provided by both Si and SiNp may be linked with high levels of reduced ascorbate and glutathione, which are crucial non-enzymatic antioxidant having low molecular weight and act in coordinative way in AsA-GSH cycle for the elimination of H2O2 (Tables 3, 4). Oxidative stress induced by AsV (25 and 50 μM) in maize cultivar and hybrid were further correlated with the redox status of ascorbate (AsA+DHA, AsA, and DHA) and glutathione (GSH+GSSG, GSH, and GSSG). It has been shown that ascorbate (AsA) plays an important role in scavenging ROS directly and indirectly (Foyer and Noctor, 2011; Singh et al., 2015a). Furthermore, the protective role of ascorbate (AsA) against oxidative stress has been demonstrated in Arabidopsis ascorbate-deficient (vtc1) mutant (Gao and Zhang, 2008). Another antioxidant, GSH, plays an important role in the synthesis of phytochelatins and in the removal of ROS (Kalinowska and Pawlik-Skowrónska, 2010) and also acts as a substrate in the detoxification of peroxidation products of lipids and proteins via the activity of glutathione-S-transferase (Li et al., 2006; Gajewska and Skłodowska, 2010). Chen et al. (2010) have reported that exogenous application of GSH alleviates Cd-induced accumulation of , H2O2, and MDA in barley seedlings. The results of the present study showed that AsV (25 and 50 μM) severely alters the redox status of AsA and GSH (Tables 3, 4). Though AsA+DHA and GSH+GSSG increased by AsV (25 and 50 μM) exposures, however, DHA and GSSG were greatly increased, which result into a steep decline in AsA/DHA and GSH/GSSG ratios (Tables 3, 4). These results clearly reveal that AsV (25 and 50 μM) stress causes alteration in the redox status of the cell by interfering with AsA and GSH pools. However, addition of Si and SiNp restores the redox status of AsA and GSH pools as indicated by high ratios of AsA/DHA and GSH/GSSG and thus maintaining the redox buffering in maize cultivar and hybrid seedling (Tables 3, 4). The results of enhanced AsA and GSH contents correspond with the enhanced activities of the AsA-GSH cycle enzymes i.e., GR and DHAR and thus, suggesting a role of Si and SiNp in triggering up-regulation of antioxidant defense system. Further, these results show that application of Si and SiNp under AsV (25 and 50 μM) stress re-establishes the redox status of the cell and thus mitigates AsV toxicity.
Conclusion
The results showed that AsV (25 and 50 μM) caused decreases in growth of maize cultivar and hybrid seedlings by interfering with the photosynthesis, contents of photosynthetic pigments, SOD, APX, GR, DHAR, AsA, and GSH by enhancing oxidative damage through the accumulation of As. However, application of Si and SiNp protects maize cultivar and hybrid seedlings against AsV by decreasing the accumulation of As and ROS, and enhancing levels of SOD, APX, GR, DHAR, AsA, and GSH, which re-establish the redox status of the cell and thus mitigate AsV toxicity. Comparing results of Si and SiNp, it was observed that reductions in the accumulation of As and oxidative stress markers were higher under SiNp and AsV combinations than Si, which is due to greater availability of SiNp to seedlings than Si. Further, results show that levels of components of the AsA-GSH cycle were higher in SiNp and AsV combinations than Si, which subsequently counterbalance ROS mediated damage to macromolecules more efficiently. Thus, the present study provides evidence that SiNp were more effective than Si in reducing AsV stress in maize cultivar and hybrid. Further, the results of the present study may be interesting from agronomical point of view in crop breeding.
Author Contributions
DKT, VPS, SMP, DKC, and NKD designed experiments. DKT and SS performed experiments. DKT, SS and VPS, analyzed data and wrote the manuscript.
Conflict of Interest Statement
The authors declare that the research was conducted in the absence of any commercial or financial relationships that could be construed as a potential conflict of interest.
Acknowledgments
DKT is thankful to the University Grants Commission, New Delhi for providing Dr. D. S. Kothari Post Doctoral Fellowship. The authors are thankful to Prof. A. C. Pandey and Dr. Prashant Kumar Singh, Nanotechnology Application Centre, University of Allahabad, Allahabad for providing SEM and XRD facilities to characterize SiNp. Authors are extended their gratitude to IIT, BHU for providing the EDX facility.
Abbreviations
AsA, reduced ascorbate; APX, ascorbate peroxidase; DHA, dehydroascorbate; DHAR, dehydrateascorbate reductase; Fv/Fm, maximum photochemical efficiency of PS II; GR, glutathione reductase; GSH, reduced glutathione; GSSG, oxidized glutathione; H2O2, hydrogen peroxide; MDA, malondialdehyde; NPQ, non-photochemical quenching; qP, photochemical quenching; ROS, reactive oxygen species; Si, silicon; SiNp, silicon nanoparticle; SOD, superoxide dismutase; SOR, superoxide radical.
References
Adrees, M., Ali, S., Rizwan, M., Zia-ur-Rehman, M., Ibrahim, M., Abbas, F., et al. (2015). Mechanisms of silicon-mediated alleviation of heavy metal toxicity in plants: a review. Ecotoxicol. Environ. Saf. 119, 186–197. doi: 10.1016/j.ecoenv.2015.05.011
Asada, K. (2006). Production and scavenging of reactive oxygen species in chloroplasts and their functions. J. Plant Physiol. 141, 391–396. doi: 10.1104/pp.106.082040
Brehe, J. E., and Burch, H. B. (1976). Enzymatic assay for glutathione. Anal. Biochem. 74, 189–197. doi: 10.1016/0003-2697(76)90323-7
Chen, F., Wang, F., Wu, F., Mao, W., Zhang, G., and Zhou, M. (2010). Modulation of exogenous glutathione in antioxidant defense system against Cd stress in the two barley genotypes differing in Cd tolerance. Plant Physiol. Biochem. 48, 663–672. doi: 10.1016/j.plaphy.2010.05.001
Currie, H. A., and Perry, C. C. (2007). Silica in plants: biological, biochemical and chemical studies. Ann. Bot. 100, 1383–1389. doi: 10.1093/aob/mcm247
Dickinson, M., and Scott, T. B. (2010). The application of zerovalent iron nanoparticles for the remediation of a uranium contaminated waste effluent. J. Hazard. Mater. 178, 171–179. doi: 10.1016/j.jhazmat.2010.01.060
Ding, W., Liu, W., Cooper, K. L., Qin, X. J., de Souza Bergo, P. L., Hudson, L. G., et al. (2009). Inhibition of poly(ADP-ribose) polymerase-1 by arsenite interferes with repair of oxidative DNA damage. J. Biol. Chem. 384, 6809–6817. doi: 10.1074/jbc.M805566200
Dubchak, S., Ogar, A., Mietelski, J. W., and Turnau, K. (2010). Influence of silver and titanium nanoparticles on arbuscular mycorrhiza colonization and accumulation of radio caesium in Helianthus annuus. Span. J. Agric. Res. 8, 103–108. doi: 10.5424/sjar/201008S1-1228
Elstner, E. F., and Heupel, A. (1976). Inhibition of nitrite formation from hydroxyl ammonium chloride: a simple assay for superoxide dismutase. Anal. Biochem. 70, 616–620. doi: 10.1016/0003-2697(76)90488-7
Epstein, E. (1999). Silicon. Annu. Rev. Plant Physiol. Plant Mol. Biol. 50, 641–664. doi: 10.1146/annurev.arplant.50.1.641
Feng, J. P., Shi, Q. H., and Wang, X. F. (2009). Effects of exogenous silicon on photosynthetic capacity and antioxidant enzyme activities in chloroplast of cucumber seedlings under excess manganese. Agric. Sci. China 8, 40–50. doi: 10.1016/S1671-2927(09)60007-9
Foyer, C. H., and Noctor, G. (2011). Ascorbate and glutathione: the heart of the redox hub. Plant Physiol. 155, 2–18. doi: 10.1104/pp.110.167569
Gajewska, E., and Skłodowska, M. (2010). Differential effect of equal copper, cadmium and nickel concentration on biochemical reactions in wheat seedlings. Ecotoxicol. Environ. Saf. 73, 996–1003. doi: 10.1016/j.ecoenv.2010.02.013
Gao, Q., and Zhang, L. (2008). Ultraviolet-B-induced oxidative stress and antioxidant defense system responses in ascorbate-deficient vtc1 mutants of Arabidopsis thaliana. J. Plant Physiol. 165, 138–148. doi: 10.1016/j.jplph.2007.04.002
Giannopolitis, C. N., and Reis, S. K. (1977). Superoxide dismutase. I. Occurrence in higher plants. Plant Physiol. 59, 309–314. doi: 10.1104/pp.59.2.309
Gossett, D. R., Millhollon, E. P., and Cran, L. M. (1994). Antioxidant response to NaCl stress in salt-sensitive cultivars of cotton. Crop Sci. 34, 706–714. doi: 10.2135/cropsci1994.0011183X003400030020x
Heath, R. L., and Packer, L. (1968). Photoperoxidation in isolated chloroplasts I. Kinetics and stoichiometry of fatty acid peroxidation. Arch. Biochem. Biophys. 125, 189–198. doi: 10.1016/0003-9861(68)90654-1
Horiguchi, T., and Morita, S. (1987). Mechanism of manganese toxicity and tolerance of plants VI. Effect of silicon on alleviation of manganese toxicity of barley. J. Plant Nutr. 10, 2299–2310. doi: 10.1080/01904168709363778
Kalinowska, R., and Pawlik-Skowrónska, B. (2010). Response of two terrestrial green microalgae (Chlorophyta, Trebouxiophyceae) isolated from Cu-rich and unpolluted soils to copper stress. Environ. Pollut. 158, 2778–2785. doi: 10.1016/j.envpol.2010.03.003
Kertulis-Tartar, G. M., Rathinasabapathi, B., and Ma, L. Q. (2009). Characterization of glutathione reductase and catalase in the fronds of two Pteris ferns upon arsenic exposure. Plant Physiol. Biochem. 47, 960–965. doi: 10.1016/j.plaphy.2009.05.009
Kidd, P. S., Llugany, M., Poschenrieder, C., Guns, B., and Barcel, J. (2001). The role of root exudates in aluminium resistance and silicon-induced amelioration of aluminium toxicity in three varieties of maize (Zea mays L.). J. Exp. Bot. 52, 1339–1352. doi: 10.1093/jexbot/52.359.1339
Kopittke, P. M., de Jonge, M. D., Menzies, N. W., Wang, P., Donner, E., McKenna, B. A., et al. (2012). Examination of the distribution of arsenic in hydrated and fresh cowpea roots using two-and three-dimensional techniques. Plant Physiol. 159, 1149–1158. doi: 10.1104/pp.112.197277
Kuniak, E., and Skodowska, M. (2001). Ascorbate, glutathione and related enzymes in chloroplasts of tomato leaves infected by Botrytis cinera. Plant Sci. 169, 723–731. doi: 10.1016/S0168-9452(00)00457-X
Li, W., Chen, T., Huang, Z., Lei, M., and Liao, X. (2006). Effect of arsenic on chloroplast ultrastructure and calcium distribution in arsenic hyper accumulator Pteris vittata L. Chemosphere 62, 803–809. doi: 10.1016/j.chemosphere.2005.04.055
Liang, Y. C., Chen, Q., Liu, Q., Zhang, W. H., and Ding, R. X. (2003). Exogenous silicon (Si) increases antioxidant enzyme activity and reduces lipid peroxidation in roots of salt-stressed barley (Hordeun vulgare L.). J. Plant Physiol. 160, 1157–1164. doi: 10.1078/0176-1617-01065
Lichtenthaler, H. K. (1987). Chlorophylls and carotenoids: pigments of photosynthetic biomembranes. Methods Enzymol. 148, 350–382. doi: 10.1016/0076-6879(87)48036-1
Liu, C., Li, F., Luo, C., Liu, X., Wang, S., Liu, T., et al. (2009). Foliar application of two silica sols reduced cadmium accumulation in rice grains. J. Hazard. Mater. 161, 1466–1472. doi: 10.1016/j.jhazmat.2008.04.116
McClintock, T. R., Chen, Y., Bundschuh, J., Oliver, J. T., Navoni, J., Olmos, V., et al. (2012). Arsenic exposure in Latin America: biomarkers, risk assessments and related health effects. Sci. Total Environ. 429, 76–91. doi: 10.1016/j.scitotenv.2011.08.051
Mittler, R., Vanderauwera, S., Gollery, M., and Van Breusegem, F. (2004). Reactive oxygen gene network of plants. Trends Plant Sci. 9, 490–498. doi: 10.1016/j.tplants.2004.08.009
Nair, R., Varghese, S. H., Nair, B. G., Maekawa, T., Yoshida, Y., and Kumar, D. S. (2010). Nanoparticulate material delivery to plants. Plant Sci. 179, 154–163. doi: 10.1016/j.plantsci.2010.04.012
Nakano, Y., and Asada, K. (1981). Hydrogen peroxide is scavenged by ascorbate specific peroxidase in spinach chloroplasts. Plant Cell Physiol. 22, 867–880.
Nordstrom, D. K. (2002). Public health. Worldwide occurrences of arsenic in ground water. Science 296, 2143–2145. doi: 10.1126/science.1072375
Patel, K. S., Shrivas, K., Brandt, R., Jakubowski, N., Corns, W., and Hoffmann, P. (2005). Arsenic contamination in water, soil, sediment and rice of central India. Environ. Geochem. Health 27, 131–145. doi: 10.1007/s10653-005-0120-9
Savant, N. K., Snyder, G. H., and Datnoff, L. E. (1997). Silicon management and sustainable rice production. Adv. Agron. 58, 151–199. doi: 10.1016/S0065-2113(08)60255-2
Schaedle, M., and Bassham, J. A. (1977). Chloroplast glutathione reductase. Plant Physiol. 59, 1011–1022. doi: 10.1104/pp.59.5.1011
Schmidt, R. E., Zhang, X., and Chalmers, D. R. (1999). Response of photosynthesis and superoxide dismutase to silica applied to creeping bentgrass grown under two fertility levels. J. Plant Nutr. 22, 1763–1773. doi: 10.1080/01904169909365752
Shi, Q., Bao, Z., Zhu, Z., He, Y., Qian, Q., and Yu, J. (2005). Silicon-mediated alleviation of Mn toxicity in Cucumis sativus in relation to activities of superoxide dismutase and ascorbate peroxidase. Phytochemistry 66, 1551–1559. doi: 10.1016/j.phytochem.2005.05.006
Singh, R., Singh, S., Parihar, P., Singh, V. P., and Prasad, S. M. (2015). Arsenic contamination, consequences and remediation techniques: a review. Ecotoxicol. Environ. Saf. 112, 247–270. doi: 10.1016/j.ecoenv.2014.10.009
Singh, V. P., Singh, S., Kumar, J., and Prasad, S. M. (2015a). Hydrogen sulfide alleviates toxic effects of arsenate in pea seedlings through up-regulation of the ascorbate-glutathione cycle: possible involvement of nitric oxide. J. Plant Physiol. 181, 20–29. doi: 10.1016/j.jplph.2015.03.015
Singh, V. P., Singh, S., Kumar, J., and Prasad, S. M. (2015b). Investigating the roles of ascorbate-glutathione cycle and thiol metabolism in arsenate tolerance in ridged Luffa seedlings. Protoplasma 252, 1217–1229. doi: 10.1007/s00709-014-0753-6
Singh, V. P., Srivastava, P. K., and Prasad, S. M. (2013). Nitric oxide alleviates arsenic-induced toxic effects in ridged Luffa seedlings. Plant Physiol. Biochem. 71, 155–163. doi: 10.1016/j.plaphy.2013.07.003
Singh, V. P., Tripathi, D. K., Kumar, D., and Chauhan, D. K. (2011). Influence of exogenous silicon addition on aluminium tolerance in rice seedlings. Biol. Trace Elem. Res. 144, 1260–1274. doi: 10.1007/s12011-011-9118-6
Sooksaen, P., Suttiruengwong, S., Oniem, K., Ngamla-miad, K., and Atireklapwarodom, J. (2008). Fabrication of porous bioactive glass-ceramics via decomposition of natural fibres. J. Metals Mater. Miner. 18, 85–91.
Strasser, R. J., Srivastava, A., and Tsimilli-Michael, M. (2000). The Fluorescence Transient As a Tool to Characterize and Screen Photosynthetic Samples. Probing Photo- synthesis: Mechanisms, Regulation and Adaptation. New York, NY; London: Taylor and Francis.
Suriyaprabha, R., Karunakaran, G., Yuvakkumar, R., Rajendran, V., and Kannan, N. (2012). Silica nanoparticles for increased silica availability in maize (Zea mays L.) seeds under hydroponic conditions. Curr. Nanosci. 8, 902–908. doi: 10.2174/157341312803989033
Tripathi, D. K., Singh, V. P., Kumar, D., and Chauhan, D. K. (2012). Impact of exogenous silicon addition on chromium uptake, growth, mineral elements, oxidative stress, antioxidant capacity, and leaf and root structures in rice seedlings exposed to hexavalent chromium. Acta Physiol. Plant. 34, 279–289. doi: 10.1007/s11738-011-0826-5
Tripathi, D. K., Singh, V. P., Prasad, S. M., Chauhan, D. K., Dubey, N. K., and Rai, A. K. (2015). Silicon-mediated alleviation of Cr(VI) toxicity in wheat seedlings as evidenced by chlorophyll florescence, laser induced breakdown spectroscopy and anatomical changes. Ecotoxicol. Environ. Saf. 113, 133–144. doi: 10.1016/j.ecoenv.2014.09.029
Velikova, V., Yordanov, I., and Edreva, A. (2000). Oxidative stress and some antioxidant system in acid rain-treated bean plants. Plant Sci. 151, 59–66. doi: 10.1016/S0168-9452(99)00197-1
Wang, M., Chen, L., Chen, S., and Ma, Y. (2012). Alleviation of cadmium-induced root growth inhibition in crop seedlings by nanoparticles. Ecotoxicol. Environ. Saf. 79, 48–54. doi: 10.1016/j.ecoenv.2011.11.044
Wang, S., Wang, F., and Gao, S. (2015). Foliar application with nano-silicon alleviates Cd toxicity in rice seedlings. Environ. Sci. Pollut. Res. 22, 2837–2845. doi: 10.1007/s11356-014-3525-0
Wilder, J. W., Venema, L. C., Rinzler, A. G., Smalley, R. E., and Dekker, C. (1998). Electronic structure of atomically resolved carbon nanotubes. Nature 391, 59–62. doi: 10.1038/34139
Keywords: arsenate, maize, photosynthesis, silicon, SiNp
Citation: Tripathi DK, Singh S, Singh VP, Prasad SM, Chauhan DK and Dubey NK (2016) Silicon Nanoparticles More Efficiently Alleviate Arsenate Toxicity than Silicon in Maize Cultiver and Hybrid Differing in Arsenate Tolerance. Front. Environ. Sci. 4:46. doi: 10.3389/fenvs.2016.00046
Received: 17 October 2015; Accepted: 03 June 2016;
Published: 07 July 2016.
Edited by:
Aziz Ullah, Kohat University of Science and Technology, PakistanReviewed by:
Peter Rolf Richter, Friedrich-Alexander University Erlangen-Nürnberg, GermanyHoda Ahmed, King Faisal University, Saudi Arabia
Copyright © 2016 Tripathi, Singh, Singh, Prasad, Chauhan and Dubey. This is an open-access article distributed under the terms of the Creative Commons Attribution License (CC BY). The use, distribution or reproduction in other forums is permitted, provided the original author(s) or licensor are credited and that the original publication in this journal is cited, in accordance with accepted academic practice. No use, distribution or reproduction is permitted which does not comply with these terms.
*Correspondence: Vijay Pratap Singh, dmlqYXlwcmF0YXAuYXVAZ21haWwuY29t
Sheo Mohan Prasad, cHJvZnNtcHJhc2FkQGdtYWlsLmNvbQ==
Devendra Kumar Chauhan, ZGtjaGF1aGFuYXVAeWFob28uY29t
Nawal Kishore Dubey, bmtkdWJleWJodUBnbWFpbC5jb20=