- 1Environmental and Life Science Graduate Program, Trent University, Peterborough, ON, Canada
- 2Department of Biology, Trent University, Peterborough, ON, Canada
Food quality is highly dynamic within lake ecosystems and varies spatially and temporally over the growing season. Consumers may need to continuously adjust their metabolism in response to this variation in dietary nutrient content. However, the rates of metabolic responses to changes in food nutrient content has received little direct study. Here, we examine responses in two metabolic phosphorus (P) pools, ribonucleic acids (RNA) and adenosine triphosphate (ATP), along with body mass and body P content in Daphnia magna exposed to chronic and acute dietary P-limitation. First, we examined food quality effects on animals consuming different food carbon (C):P quality over a 14 day period. Then, we raised daphnids on one food quality for 4 days, switched them to contrasting dietary treatments, and measured changes in their metabolic responses at shorter time-scales (over 48 h). Animal P, RNA, and ATP content all changed through ontogeny with adults containing relatively less of these pools with increasing body mass. Irrespective of age, Daphnia consuming high C:P diets had lower body %P, %RNA, %ATP, and mass compared to animals eating low C:P diets. Diet switching experiments revealed diet dependent changes in body %P, %RNA, %ATP, and animal mass within 48 h. We found that Daphnia switched from low to high C:P diets had some metabolic buffering capacity with decreases in body %P occurring after 24 h but mass remaining similar to initial diet conditions for 36 h after the diet switch. Switching Daphnia from low to high C:P diets caused a decrease in the RNA:P ratio after 48 h. Daphnia switched from high to low C:P diets increased their body P, RNA, and ATP content within 8–24 h. This switch from high to low C:P diets also led to increased RNA:P ratios in animal bodies. Overall, our study revealed that consumer P metabolism reflects both current and past diet due to more dynamic and rapid changes in P biochemistry than total body mass. This metabolic flexibility is likely linked to resource integration in D. magna, which reduces the negative effects of short-term or variable exposure to nutrient-deficient foods.
Introduction
Consumers frequently face nutritional limitation in aquatic environments due to imbalances between limited supplies of elemental resources and the metabolic demands of consumers (Frost et al., 2005). Within lakes, the nutritional quality of seston (i.e., suspended particulate organic matter) varies across both short (e.g., weekly and possibly daily) and long (e.g., seasonally) timescales (Kreeger et al., 1997; Hessen et al., 2005). This variation in food quality should lead to fluctuating imbalances and elemental constraints on organismal performance and life-histories particularly for high-phosphorus (P) taxa such as Daphnia. Despite this, it remains unclear whether or how animals adjust metabolically to short- vs. long-term nutrient stress or how these adjustments would relate to consumer growth and body stoichiometry.
One well established effect of sustained periods of elemental limitation on consumers is slower growth rates as rates of P acquisition fail to match metabolic requirements to build new body tissues (Frost et al., 2005). However, when faced with heterogeneous dietary P supply, Daphnia appear to employ one of two growth mechanisms: growth or resource integration (Hood and Sterner, 2010). Growth integration is thought to occur in more homeostatic Daphnia species, where their growth rate is a function of the average C:P ratio of their fluctuating diets. This indicates when Daphnia encounter increasing or decreasing dietary nutrients their growth rates will match the supply of dietary P quickly (Hood and Sterner, 2010). Resource integration, on the other hand, may be used by less homeostatic Daphnia species, which possibly retain P when feeding on low C:P ratio diets for use when dietary C:P ratios subsequently increase (Hood and Sterner, 2010). Under this mechanism, growth will remain stable for a period of time after the nutrient quality of food is changed. For example, when switching from a low C:P to a high C:P diet, resource integrators should maintain growth for a period of time using stored nutrient pools to fuel growth. Once these pools are exhausted, growth integrators should decrease their growth to the new nutrient conditions. However, it is unknown how Daphnia carry over P from favorable environments as they are apparently unable to store significant quantities of P in vacuoles as documented for plants, algae, and bacteria (Frost et al., 2005).
One possible location of surplus P retention is within daphnid metabolic P pools, which may be adjustable over both long (days) and short (minutes to hours) time scales. These temporary pools could decouple animal nutrient content from growth requirements and alleviate growth constraints imposed by P deficient diets. Longer-term P retention might be achieved by altering tissue content of larger P pools such as ribosomes. As body ribonucleic acid (RNA) content is highly correlated with ribosome production and growth rate in zooplankton (Elser et al., 2003), decreasing dietary P content could lead to ribosome catabolism and the reallocation of released P toward the transcription of enzyme mRNA (e.g., alkaline phosphatase) needed to increase P-acquisition or for the maintenance of intracellular metabolic pools (Acquisti et al., 2009; Weiße et al., 2015). However, catabolizing ribosomes would not be an efficient response to short-term changes to dietary food quality, due to the high metabolic costs of ribosome synthesis and catabolism (Houseley and Tollervey, 2009). Alternatively, smaller P pools including energy metabolites (e.g., adenosine triphosphate; ATP) are known to rapidly change the energy status of the cell (Hardie, 2003), and changes in this pool may alleviate short-term responses to P-limitation (Wagner et al., 2015) that could be utilized for fine-scale variation (hours) in dietary P. The importance of these metabolite sources as P sources has not been generally considered from a stoichiometric perspective because ATP represents a very small amount of overall body P-content (Elser et al., 1996). However, as ATP has a role in P delivery in cells as the conversion of ATP to ADP yields an available phosphate molecule (Hardie, 2003), ATP thus may provide a mechanism, albeit a short-term one, for consumers to rapidly respond to changes in dietary C:P ratios. Alternatively, as ATP and other energy molecules are tightly regulated within the cells, they may be insensitive to dietary P and fluctuate little with changes in dietary P supply.
Here we examined responses of body P, RNA, and ATP content over short- (hours to days) and long-term (weeks) exposure to dietary P-limitation in the freshwater zooplankter, Daphnia. We hypothesized that body %P, %RNA, and %ATP are affected by both the duration and intensity of P-limitation due to imbalances between the dietary supply of and metabolic demand for P created by current and past diets. Under chronic limitation, we predicted that body mass, P, RNA, and ATP content would decrease throughout ontogeny in animals consuming high C:P diets. However, under acute dietary changes, we expected resource integration to be the predominant mechanism in D. magna as described by Hood and Sterner (2010). We predicted that animals switched from low to high C:P would continue to maintain their mass for a period of time and utilize their body P stores to fuel biomass production. After these body P pools decrease, daphnid biomass production (weight gain) should also decrease especially if compared to animals maintained in low C:P diets. If this true, we would expected to see body %P and ATP decrease before eventual changes to body RNA content. Whereas, when daphnids are switched from high to low C:P diets, we predicted their mass increase will also have a time lag. This lag between when animals encounter low C:P diets and mass increases is necessary to increase P pools to initiate ribosome production. Specifically, we predicted that body P content would change first, followed by ATP and RNA content, as P uptake is required before the production of these biomolecules can occur. Additionally, we predicted that more extreme dietary P switches would result in faster P pool changes. Alternatively, if growth integration is the main mechanism used by D. magna in heterogeneous dietary conditions we would expect the daphnids mass increase or decrease to the changing dietary P conditions at the same rate as the change in P-pools.
Methods and Analysis
Algae and Daphnia Culturing
We varied Scenedesmus obliquus C:P content (Canadian Phycological Culture Centre strain 10, purchased as S. acutus) by diluting semi-continuous cultures daily with differentially supplied P (Sterner et al., 1993; Wagner et al., 2015), to produce a range of C:P ratios (by mol). Low, intermediate and high C:P cultures contained 70, 30, and 7 μM of P, and was diluted by 55, 20, and 10% day−1, respectively. Harvested algae were centrifuged at 3000 g and resuspended in P-free COMBO media (Kilham et al., 1998). The P-content of concentrated algal suspensions was determined on dry subsamples using the molybdate-blue colorimetric method after persulfate oxidation (APHA, 1992). Subsamples of concentrated algal cultures were also filtered onto ashed GF/C glass fiber filters to determine algal C and nitrogen (N) content using a CN Analyzer (Vario EL III, Elementar Incorporated, Mt Laurel NJ, USA). These data were used to mix nominal concentrations of the prescribed diets, and post-mixing verification was done to calculate the actual C:P ratios of each diet (Wagner and Frost, 2012; Wagner et al., 2015; Prater et al., 2016).
These diets were used to study the chronic and acute responses of animal mass and body %P, %RNA, and %ATP in Daphnia magna. Animals used in these experiments were clonal sisters who were held in groups of 10 animals in 400 ml of P free COMBO media (Kilham et al., 1998) and fed high nutrient quality (~ C:P 80) S. obliquus ad libitum. On the morning of each experiment, we removed neonates (<24 h old) from brood jars ensuring only to use animals born in the second to fourth brood.
Responses to Chronic P-Limitation
Neonates were triple rinsed with P-free COMBO to remove residual nutrients and algal particles, and all animals were allocated to experimental treatments within an hour. Animals were grown in separate vials containing 20 mL of P-free COMBO from days 0–5 and in 40 mL of P-free COMBO from day 6–14. Daphnids were fed 4 mg CL−1 (day 0–5) to 8 mg CL−1 (day 6–14) of their prescribed diet (C:P 100 ± 8, 300 ± 24, 500 ± 45, and 700 ± 12) every other day. After 3, 6, 10, and 14 days of growth, animals were saved for body %P, %RNA, %ATP, and mass analyses to examine changes in the metabolic P-pools with chronic exposure to dietary P-limitation (Table S1).
Acute Changes in P Supply
To determine how short-term changes in dietary P supply affects animal mass, body %P, %RNA, and %ATP we grew Daphnia in separate 20 mL vials of P-free COMBO and fed them 4 mg C L−1 of a low C:P diet (C:P 100 ± 10) on days 0 and 2. On day 4, animals were rinsed, placed in 20 mL of P-free COMBO, and fed the same low C:P diets (C:P 92; nominal C:P 100) or were switched to intermediate or high C:P diets (C:P 289; nominal C:P 300 or C:P 679; nominal C:P 700, respectively). We also performed high to low C:P dietary switches by growing animals at intermediate (C:P 275 ± 20; nominal C:P 300) or high (C:P 710 ± 2; nominal C:P 700) food qualities for 4 days then switching half to low C:P diet (C:P 103 ± 9; nominal C:P 100) with the other half being maintained in the initial C:P 300/C:P 700 diets. Animal mass, body P, RNA, and ATP content were assessed for all contrasts in P-supply after 1, 2, 4, 8, 12, 24, 36, and 48 h (Table S1).
Animal Processing
After D. magna were individually grown, we saved animals for mass (n = 10), body %P, %RNA, and %ATP estimates (n = 5; Table S1). As dry weights could not be obtained for body %ATP and %RNA, we used a dissecting microscope to measure from the top of their eye spot to their tail spine the nearest μm using a digital camera and image software (iSolutions). The corresponding dry mass was calculated using a length-mass regression (R2 = 0.92). Immediately after length measurements were taken, animals were transferred individually to 0.6 mL microfuge tubes and used to estimate body ATP or RNA content. Daphnia for body %RNA measurements were preserved with the addition of 100 μL RNAlater and stored at −80°C until analyzed, while animals used to quantify body %ATP were extracted right away to prevent ATP degradation.
Body P Content
Groups of 3–5 individual Daphnia from each sampling were placed in an aluminum cup and dried for 12 h at 60°C before being weighed on a microbalance to the nearest μg (n = 5). Samples were subsequently oxidized using persulfate and analyzed using the molydbate-blue colorimetric method (APHA, 1992). Animal P content was determined through visible spectrometry at 885 nm by comparing absorbance of samples to P standards.
Body RNA Content
Body %RNA content was determined following Wagner et al. (2015). Individual daphnids (n = 5) were removed from −80°C freezer and rinsed using TE buffer to remove RNAlater®. Daphnia was then transferred to a new 1.5 mL centrifuge tube containing 500 μL of TE buffer and 0.1 mm glass beads filled to the 0.1 mL mark on the centrifuge tube. Centrifuge tubes were placed in mechanical agitator (Bullet Blender 24, Next Advance) set on speed 8 for 3 min to fully homogenize Daphnia. Two subsamples of 50 μL were removed from each sample to determine nucleic acid fractions. To the DNA fraction, 50 μL of RNase (0.1 μg/mL) was added while 50 μL of TE buffer were added to the total nucleic acid fraction. Samples were incubated at 37°C for 15 min, and 75 μL of each sample was added to black 96 well microplate. To each well, 75 μL of RiboGreen was added and incubated for 5 min in the dark. RNA/DNA content was determined by fluorescence through the use of a microplate reader (BioTek SynergyHT, Gen5 software) with the excitation 485/20 nm and emission 528/20 nm. Body RNA content was determined by subtracting the DNA fluorescence fraction from the total nucleic acid fluorescence.
Body ATP Content
After length was determined for ATP animals, individual animals were transferred to a 0.6 mL centrifuge tube and the COMBO was removed with a pipette. We added 100 μL of −20°C methanol to each centrifuge tube to stop all enzymatic activity and preserve ATP. Daphnids were homogenized with a motorized pestle for 1 min to ensure cell lysis. An additional 400 μL of −20°C methanol was added to make the final volume of 500 μL. Body %ATP was analyzed by a stable luminescence assay CellTiter-Glo® (Promega) following manufacturer's protocols. Briefly, samples were vortexed for 30 s, and 50 μL was pipetted in an opaque 96 well microplate with the addition of 50 μL of CellTiter-Glo®. ATP standards (Adenosine 5′-triphosphate disodium salt hydrate <99% purity) were prepared with methanol and were treated the same as samples to determine the ATP concentrations. After the addition of CellTiter-Glo®, the microplate was incubated in the dark for 5 min, placed into a microplate reader (BioTek SynergyHT, Gen5 software), and luminescence was measured with a sensitivity of 53.
Statistics
We analyzed the chronic and acute responses to food quality using 2-way ANOVAs with interaction examining the effects of age/time and diet for all response variables. Post-hoc differences between diets for each day/time were determined after Bonferroni corrections for multiple comparisons with a cumulative α < 0.05 (P < 0.0125 chronic experiments, P < 0.0062 for acute experiments).
Results
Chronic P-Limitation
We found significant interactive effects between age and dietary C:P ratio for animal mass, body %P, %RNA, and %ATP (Table 1). While weight increased throughout the chronic experiment for animals consuming all diets, significant dietary effects emerged after 6 days but only in animals consuming food with C:P ratios of 700 (Figure 1A, Table 1). By day 14, there were significant differences in mass among all dietary food C:P ratios (Table 1). After only 3 days of growth, lower body P content was observed for all P-limited diets with the lowest values found in C:P 500 and C:P 700 animals (Figure 1B). After 6–10 days, we found body P content was significantly different among all diet C:P ratios (Table 1). After 14 days, we found the same pattern in body P content as what emerged on day 3 with no differences between the P content of animals consuming food C:P ratios of 500 and 700. Lower dietary food P content resulted in decreased body RNA content after 3 days of growth in C:P 300 treatments and after 6 days at C:P 500 and 700 (Figure 1C). However, after 10 days body %RNA was similar among all food C:P ratios (Table 1). Relatively small changes were found in body %ATP content after 3 days of growth, but after 6–10 days, we found ATP content in Daphnia was affected by dietary P content (Figure 1D, Table 1). Body %ATP was reduced in animals consuming food C:P ratios 500 and 700 after 6 days and C:P ratios of 300 after 10 days (Table 1). These differences disappeared after 14 days of growth when no differences in ATP were observed among any of the dietary conditions.
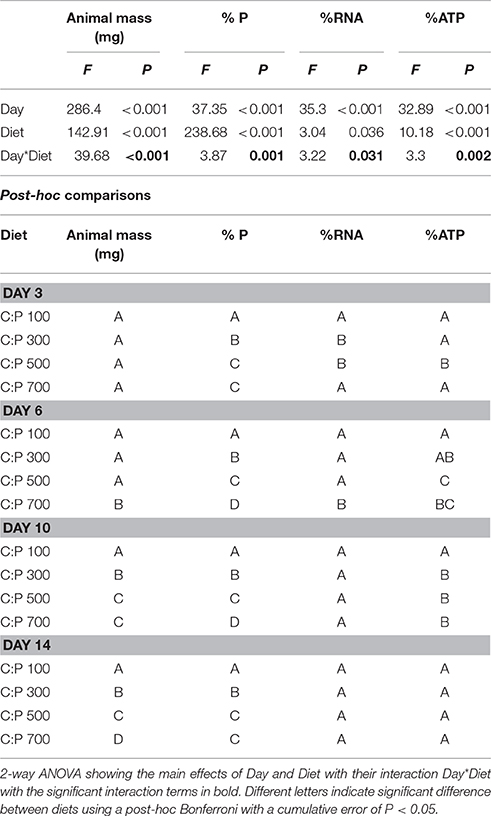
Table 1. Daphnid mass and P, RNA, and ATP content for animals grown on different C:P diets for 3, 6, 10, and 14 days.
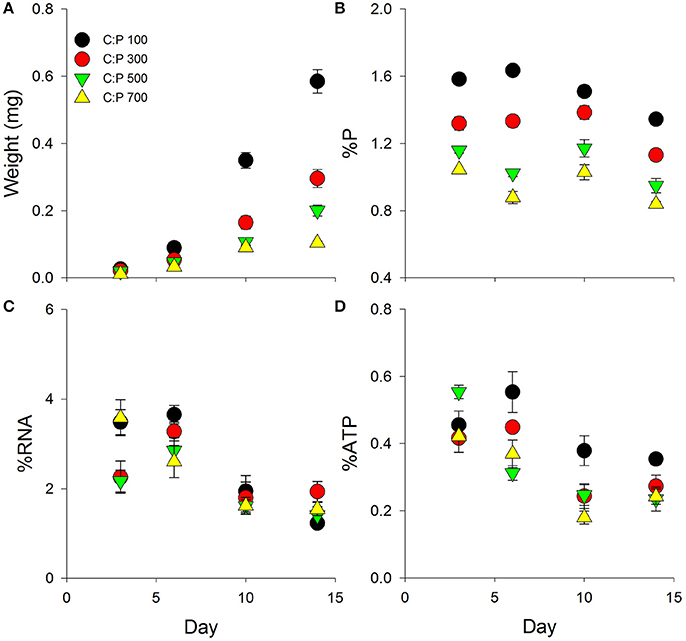
Figure 1. Chronic averages ± standard error in Daphnia mass (A) and metabolic P-pools (B; body %P, C; body %RNA, D; body %ATP) for animals grown on the same C:P diet for 3, 6, 10, and 14 days. See Table 1 for ANOVA results and post-hoc comparisons.
Acute Diet Switches from Low to High C:P Diets
Daphnia switched from C:P 100 to high food C:P ratios exhibited similar changes in body mass as animals maintained in C:P 100 diets for the first 36 h (Figure 2A). However, after 48 h animals maintained in the C:P 100 diets weighed significantly more than animals switched to high food C:P ratios; thus the biomass production (net weight gain) was less in animals switched to high C:P diets. A significant time × diet interaction was also observed for body %P with animals rapidly decreasing their P content after 12 and 24 h when switched to C:P 300 and C:P 700 diets, respectively (Figure 2B). RNA content displayed a significant time × diet interaction, with the mean daphnid RNA content decreased steadily over time when animals were switched from C:P 100 to high C:P diets (Figure 2C). Daphnia switched from C:P 100 to higher C:P diets (C:P 300 and C:P 700) displayed a significant time x diet interaction with the RNA:P ratio decreasing after 48 h compared to animals maintained in C:P 100 diets (Figure 2D). Body %ATP displayed significant main effects for time and diet for animals switched from C:P 100 to C:P 300 and 700 diets (Figure 2E). When animals were switched to C:P 700 diets, their ATP content decreased significantly between 8 and 12 h, whereas animals switched to C:P 300 diets only showed slight decreases in body %ATP through time (Figure 2E).
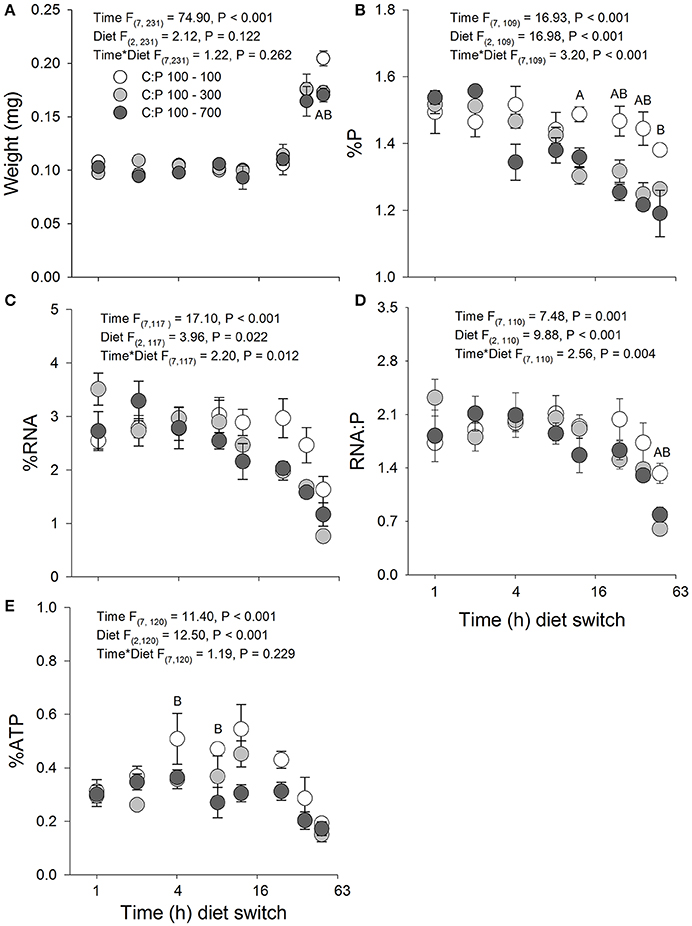
Figure 2. Acute averages ± standard error in Daphnia mass (A) and metabolic P-pools (B; body %P, C; body %RNA, D; RNA:P, E; body %ATP). White circles are animals maintained at C:P 100 while light gray, and dark gray circles represent animals that were switched to C:P 300, and C:P 700, respectively. Post-hoc significant differences between C:P 100–300 represented with (A) and CP:100–700 represented with a (B) (P < 0.006).
Acute Diet Switch from High to Low C:P Diets
Daphnia mass displayed a significant time × diet interaction with increases in biomass production in animals switched to C:P 100 diets (Figures 3A, 4A). Daphnia body mass increased more rapidly (24 h) when switched from C:P 700 than from C:P 300 (48 h) to C:P 100 diets. Changes in body P content also differed depending on the magnitude of the dietary imbalance with animals switched from C:P 700 diets increasing after 12 h compared to animals switched from C:P 300 diets, which did not change until after 24 h (Figures 3B, 4B). Daphnia %RNA, RNA:P ratios, and %ATP all displayed diet dependent changes. Body RNA content in animals switched from C:P 700 to C:P 100 diets increased significantly after 36 h (Figure 4C) but did not differ in animals switched from C:P 300 diets (Figure 3C). Daphnia switched from C:P 300 to C:P 100 diets showed no significant diet effects on their RNA:P ratios (Figure 3D). In contrast, the RNA:P ratio in animals switched from C:P 700 to C:P 100 diets displayed a significant time x diet interaction with ratios increasing after 48 h on low C:P food (Figure 4D). Animal body %ATP had significant diet and time effects with animals switched from C:P 300 diets displayed slightly higher average ATP content over time (Figure 3E). However, animals switched from C:P 700 diets showed much stronger changes as they differed significantly after 12 h and %ATP steadily increased over 48 h when we found the highest levels observed across all experiments (Figure 4E).
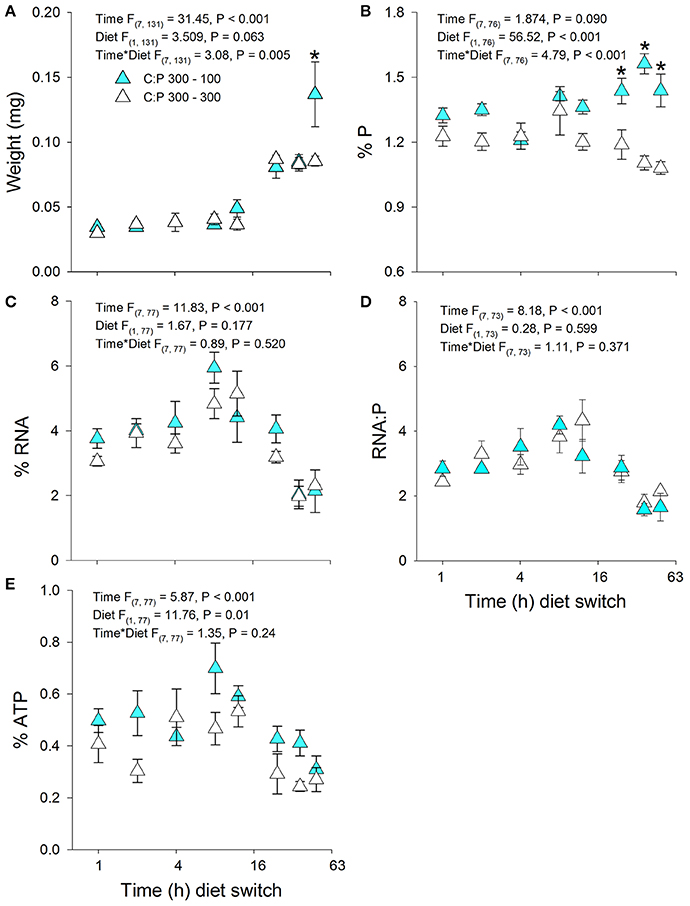
Figure 3. Acute averages ± standard error in Daphnia mass (A) and metabolic P-pools (B; body %P, C; body %RNA, D; RNA:P, E; body %ATP). White triangles are animals maintained at C:P 300 while cyan triangles representing animals that were switched to C:P 100 * indicates significant changes between switched diets and non-switched diets (P < 0.006).
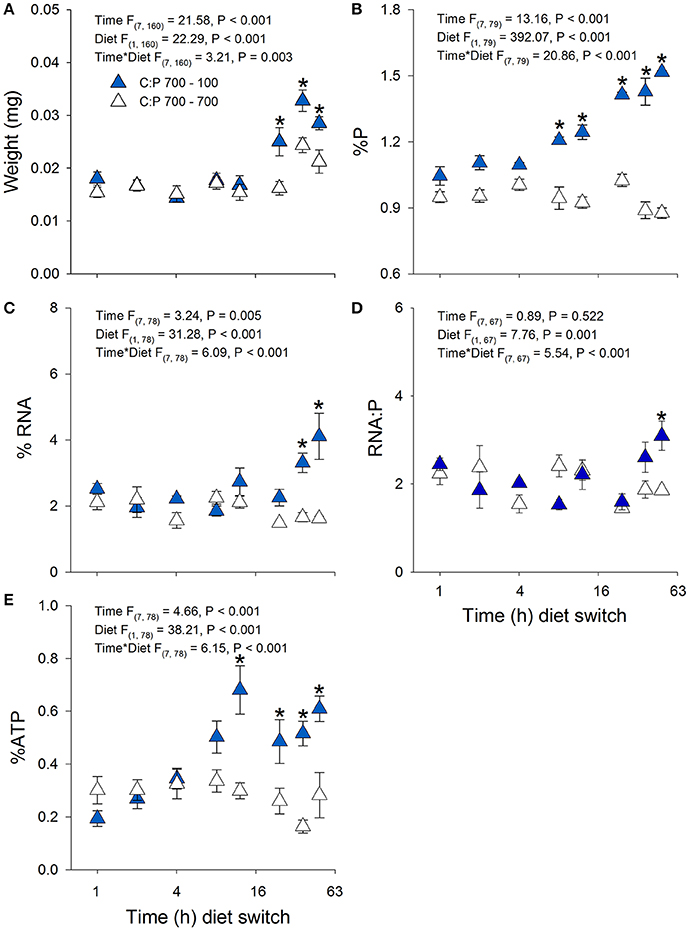
Figure 4. Acute averages ± standard error in Daphnia mass (A) and metabolic P-pools (B; body %P, C; body %RNA, D; RNA:P, E; body %ATP). White triangles are animals maintained at C:P 700 while dark blue triangles representing animals that were switched to C:P 100 * indicates significant changes between switched diets and non-switched diets (P < 0.006).
Discussion
We found evidence to support our hypothesis that body %P, %RNA, and %ATP are affected by the duration and intensity of dietary P-limitation. As predicted, chronically P-limited animals were smaller and had lower body %RNA, %P, and %ATP. These metabolic P pools also decreased in aging animals with lower content found in larger animal bodies. Our results from short-term diet changes support findings of Hood and Sterner (2010) that resource integration mechanisms occur in D. magna in a heterogeneous dietary P-supply environment. For example, daphnids were able to maintain their biomass production (net weight gain) for 36 h when switched to higher C:P diets compared to animals maintained in C:P 100 diets. Additionally, when daphnids were switched to C:P 100 they incorporated P into their tissues before body %RNA and mass increases were observed. Overall, our results indicate the P pools are extremely sensitive and flexible under variable dietary P supply. These plastic metabolic responses are likely to be highly beneficial in animals living in natural environments where they can frequently encounter large differences in food quality.
Consistent with previous results, Daphnia decreased their body %P and %RNA with high C:P diets during juvenile growth (Elser et al., 2003; Acharya et al., 2004; Wagner et al., 2015). We also found that ATP content decreased in animals provided high food C:P ratios during chronic P-limitation (Wagner et al., 2015). As predicted by the growth rate hypothesis, we saw that mass production decreased between neonate, juvenile, and adult stages concomitant with decreases in RNA and P content throughout ontogeny (Vrede et al., 1999; Elser et al., 2003). Body ATP content also decreased with age, but these changes were not linked to large declines in P content as ATP represented only 0.2–0.7% of daphnid dry mass. These dynamics are likely related to changes in life-history investment, as reduction in growth decreases the need for P-rich biomolecules (Vrede et al., 1999) and adult reproduction requires higher amounts of C-rich lipids (Tessier et al., 1983). Lipid accumulation causes the dilution of other elements and biomolecules leading to a decrease in overall P and RNA content, thus altering daphnid stoichiometry under chronic P-limitation.
When Daphnia encounter high food C:P ratios in their diets, we found that their body P content declined within 12 h while their RNA content only started to decrease between 12 and 24 h. Gut contents can be largely excluded as a cause of body %P declines, because gut residence times are generally under 1 h (Wojewodzic et al., 2011). Some of this decrease in body %P can be attributed to the slight declines in body %RNA, as the RNA:P ratio remained constant for the first 36 h. However, animals switched to high C:P diets were able to maintain biomass production (net weigh gain) equivalent to low C:P diet animals for 36 h. Thus growth can be maintained even though there are slight decreases in RNA over the first 36 h. This result is further evidence for resource integration (Hood and Sterner, 2010). After 36 h, RNA:P ratios declined indicating there is more P per RNA and suggests that RNA catabolism is occurring. The P released from the RNA catabolism could be stored in organic P metabolites (e.g., α-glycerophosphate), which have been documented to be a significant P pools found in the hemolymph of an insect (Woods et al., 2002). If cladocerans can store organic P within their hemolymph, it could provide these animals with greater abilities to increase RNA synthesis if dietary C:P ratios increase again. However, it is unknown if these animals can initiate growth faster than animals switched from a high to low C:P diets.
Switching Daphnia from high to low C:P diets caused body %P to increase 24–40 h before RNA content increased, with faster changes in body P content seen in more severely P-limited animals. This result is especially surprising as ribosomal RNA accounts for up to 80% of the total body P content (Vrede et al., 1999; Sterner and Elser, 2002) and we would expect to see changes body %P coincide with changes in RNA content. However, it is possible physiological mechanisms to deal with low P and excess C in high C:P diets remain active for hours after animals are transferred to low C:P diets. When P-limited, Daphnia increase their P-acquisition enzymes (e.g., alkaline phosphatase; McCarthy et al., 2010; Elser et al., 2011; Wagner and Frost, 2012) and display increased feeding rates (Darchambeau et al., 2003). If these mechanisms remain active for hours after animals are transferred to low C:P diets, they may contribute to the accumulation of P without direct changes in growth or RNA content. Dietary P enters through the gut lining as phosphate where it can be converted into phosphosugars (e.g., α-glycerophosphate) or metabolites such as ATP. We found evidence that P is incorporated into energy molecules as both P and ATP, increased after 8 and 12 h, respectively. These rapid changes in ATP could be priming molecular pathways with energy and P to restore P pool sizes needed to support the high material and energetic demands of ribosome and protein synthesis (Houseley and Tollervey, 2009). Altogether, these potential molecular mechanisms could allow for growth to initiate and animals to become significantly larger within 24 h when they are switched from C:P 700 to C:P 100 diets.
We found that animal body RNA:P ratios were fairly stable for the first 36 h after switching to contrasting diets. However, after 48 h the animals switched to high from low C:P diets decreased their RNA:P, while animals switched to low from high C:P diets had increased RNA:P ratios in their bodies. We suspect these changes in RNA:P ratios are caused by RNA being catabolized and contributing P into other body P pools when Daphnia are switched to high C:P diets. Whereas, increased RNA:P ratios when Daphnia are switched to low C:P diets may be due to the rapid synthesis of ribosomes that outstrips overall P accumulation by animals. Furthermore, these drastic changes in body %RNA could be caused by a change in the metabolic phenotype switching between fast and slow growth (Weiße et al., 2015). It may be important for these two distinct phenotypes to be resilient and avoid changing at the first signs of dietary heterogeneity. Delaying phenotype switches for short-time periods (i.e., up to 48 h) may prevent unnecessary energetic and resource costs of RNA anabolism and catabolism. Synthesizing large amounts of RNA when animals are switched from high to low C:P diets may have high costs associated if this process is initiated prematurely. These costs would include the resource and energetic demands of RNA synthesis and, if low C:P diets only remain for a few hours, the resource and energetic costs of breaking down RNA (Houseley and Tollervey, 2009). Premature RNA catabolism during short periods of high C:P diets could lead to slower growth and development and delayed maturity, which would have strong fitness consequences.
Overall, Daphnia responses to food quality differed with exposure duration and with diet. This variation in consumer metabolic responses has important implications for understanding consumer responses to nutrient limitation in nature. Consumers may frequently encounter spatio-temporal variation dietary food quality in nature (Kreeger et al., 1997; Interlandi et al., 1999), which would produce variable metabolic responses that are a function of the degree and direction of dietary switches. Despite this, most theory behind consumer-resource interactions has been built from studying consumer responses to chronic dietary P-limitation (but see Hood and Sterner, 2010). Thus, we lack a basic understanding of why differences in growth strategies (growth vs. resource integration) evolve and how these differences at the organismal level would alter higher order ecological processes at population and ecosystem-levels. Greater effort toward incorporating natural variation in food quality into laboratory experiments (Hood and Sterner, 2010, 2014) and in linking measurements of fine-scale resource variation and consumer metabolic and life-history responses (DeMott et al., 2004) would appear to be fertile areas of future work. In addition, our results highlight the fact that relationships between consumer growth, biochemistry, and elemental composition depend on both current and past dietary exposures. This reinforces the idea that consumer elemental composition should not be used as a proxy for demand (Frost and Elser, 2002; Hood and Sterner, 2014; Prater et al., 2016) and that calculations of consumer resource imbalances may not be sufficient to predict consumer responses to elemental limitation. Progress in understanding the ecological effects of consumer nutrient limitation therefore still hinges on the development of tools for directly assessing consumer nutrient status in natural ecosystems (Wagner et al., 2013; Frost et al., 2014).
We found the metabolic P pools and P content were plastic due to both chronic and acute exposure to in P-poor food. During chronic exposure to one dietary condition, animals in high C:P diets had lower body %P and RNA. While, over short-term exposure to contrasting C:P diets causes rapid changes in body %P, %RNA, and %ATP. Animal P content generally responded more rapidly compared to RNA and ATP content, with changes in biomass not occurring until 24–48 h after dietary switches which supports resource integration growth mechanisms. We offer a potential bottom-up molecular explanation for resource integration, where elements (i.e., P) must be present to make the biomolecules before altering growth rate. Additionally, delaying switching between slow and fast growing phenotypes is an efficient strategy for dealing with dietary elemental variation. In conclusion, we found D. magna has highly plastic metabolic P pools, which would provide growth advantages in heterogeneous food environments
Ethics Statement
This study is exempt from the animal care protocols as only invertebrates were used.
Author Contributions
NW and PF designed the project, NW and CP performed the experiments and analyzed the data, all authors contributed to writing the manuscript.
Conflict of Interest Statement
The authors declare that the research was conducted in the absence of any commercial or financial relationships that could be construed as a potential conflict of interest.
The reviewer SD and handling Editor declared their shared affiliation, and the handling Editor states that the process nevertheless met the standards of a fair and objective review.
Acknowledgments
We would like to thank Andrea Conine, Hamaza Khattak, and Charlotte Narr for their assistance maintaining algae and Daphnia cultures. Both NW and CP would like to thank Ontario Graduate Scholarship for funding assistance.
Supplementary Material
The Supplementary Material for this article can be found online at: http://journal.frontiersin.org/article/10.3389/fenvs.2017.00036/full#supplementary-material
References
Acharya, K., Kyle, M., and Elser, J. J. (2004). Effects of stoichiometric dietary mixing on Daphnia growth and reproduction. Oecologia 138, 333–340. doi: 10.1007/s00442-003-1444-8
Acquisti, C., Kumar, S., and Elser, J. J. (2009). Signatures of nitrogen limitation in the elemental composition of the proteins involved in the metabolic apparatus. Proc. R. Soc. B. Biol. Sci. 276, 2605–2610. doi: 10.1098/rspb.2008.1960
Darchambeau, F., Faerøvig, P. J., and Hessen, D. O. (2003). How Daphnia copes with excess carbon in its food. Oecologia 136, 336–346. doi: 10.1007/s00442-003-1283-7
DeMott, W. R., Pape, B. J., and Tessier, A. J. (2004). Patterns and sources of variation in Daphnia phosphorus content in nature. Aquat. Ecol. 38, 433–440. doi: 10.1023/B:AECO.0000035183.53389.66
Elser, J. J., Acharya, K., Kyle, M., Cotner, J., Makino, W., Markow, T., et al. (2003). Growth rate-stoichiometry couplings in diverse biota. Ecol. Lett. 6, 936–943. doi: 10.1046/j.1461-0248.2003.00518.x
Elser, J. J., Dobberfuhl, D. R., Mackay, N., and Schampel, J. H. (1996). Organism size life history and N:P stoichiometry. Bioscience 46, 674–684. doi: 10.2307/1312897
Elser, J. J., Hayakawa, K., and Urabe, J. (2011). Nutrient limitation reduces food quality for zooplankton: Daphnia response to seston phosphorus enrichment. Ecology 82, 898–903. doi: 10.1890/0012-9658(2001)082[0898:NLRFQF]2.0.CO;2
Frost, P. C., and Elser, J. J. (2002). Growth responses of littoral mayflies to the phosphorus content of their food. Ecol. Lett. 5, 232–240. doi: 10.1046/j.1461-0248.2002.00307.x
Frost, P. C., Evans-white, M. A., Finkel, Z. V., Jensen, T. C., and Matzek, V. (2005). Are you what you eat? Physiological constraints on organismal stoichiometry in an elementally imbalanced world. Okios 109, 18–28. doi: 10.1111/j.0030-1299.2005.14049.x
Frost, P. C., Song, K., and Wagner, N. D. (2014). A beginner's guide to nutritional profiling in physiology and ecology. Integr. Comp. Biol. 54, 873–879. doi: 10.1093/icb/icu054
Hardie, D. G. (2003). Minireview: the AMP-activated protein kinase cascade: the key sensor of cellular energy status. Endocrinology 144, 5179–5183. doi: 10.1210/en.2003-0982
Hessen, D. O., Van Donk, E., and Gulati, R. (2005). Seasonal seston stoichiometry: effects on zooplankton in cyanobacteria-dominated lakes. J. Plankton Res. 27, 449–460. doi: 10.1093/plankt/fbi018
Hood, J. M., and Sterner, R. W. (2010). Diet mixing: do animals integrate growth or resources across temporal heterogeneity? Am. Nat. 176, 651–663. doi: 10.1086/656489
Hood, J. M., and Sterner, R. W. (2014). Carbon and phosphorus linkages in Daphnia growth are determined by growth rate, not species or diet. Funct. Ecol. 28, 1156–1165. doi: 10.1111/1365-2435.12243
Houseley, J., and Tollervey, D. (2009). The many pathways of RNA degradation. Cell 136, 763–776. doi: 10.1016/j.cell.2009.01.019
Interlandi, S. J., Kilham, S. S., and Theriot, E. C. (1999). Responses of phytoplankton to varied resource availability in large lakes of the Greater Yellowstone Ecosystem. Limnol. Oceanogr. 44, 668–682. doi: 10.4319/lo.1999.44.3.0668
Kilham, S. S., Kreeger, D., Lynn, S. G., Goulden, C. E., and Herrera, L. (1998). COMBO: a defined freshwater culture medium for algae and zooplankton. Hydrobiologia 377, 147–159. doi: 10.1023/A:1003231628456
Kreeger, D., Goulden, C., Kilham, S. S., Lynn, S. G., Datta, S., Sebastian, J., et al. (1997). Seasonal changes in the biochemistry of lake seston. Freshw. Biol. 38, 539–554. doi: 10.1046/j.1365-2427.1997.00233.x
McCarthy, S. D., Rafferty, S. P., and Frost, P. C. (2010). Responses of alkaline phosphatase activity to phosphorus stress in Daphnia magna. J. Exp. Biol. 213, 256–261. doi: 10.1242/jeb.037788
Prater, C., Wagner, N. D., and Frost, P. C. (2016). Effects of calcium and phosphorus limitation on the nutritional ecophysiology of Daphnia. Limnol. Oceanogr. 61, 268–278. doi: 10.1002/lno.10208
Sterner, R. W., and Elser, J. J. (2002). Ecological Stoichiometry: The Biology of Elements from Molecules to the Biosphere. Princeton, NJ: Princeton University Press.
Sterner, R. W., Hagemeier, D. D., Smith, W. L., and Smith, R. F. (1993). Phytoplankton nutrient limitation and food quality for Daphnia. Limnol. Oceanogr. 38, 857–871. doi: 10.4319/lo.1993.38.4.0857
Tessier, A. J., Henry, L. L., Goulden, C. E., and Durand, M. W. (1983). Starvation in Daphnia: energy reserves and reproductive allocation. Limnol. Oceanogr. 28, 667–676. doi: 10.4319/lo.1983.28.4.0667
Vrede, T., Andersen, T., and Hessen, D. O. (1999). Phosphorus distribution in three crustacean zooplankton species. Limnol. Oceanogr. 44, 225–229. doi: 10.4319/lo.1999.44.1.0225
Wagner, N. D., and Frost, P. C. (2012). Responses of alkaline phosphatase activity in Daphnia to poor nutrition. Oecologia 170, 1–10. doi: 10.1007/s00442-012-2277-0
Wagner, N. D., Hillebrand, H., Wacker, A., and Frost, P. C. (2013). Nutritional indicators and their uses in ecology. Ecol. Lett. 16, 535–544. doi: 10.1111/ele.12067
Wagner, N. D., Lankadurai, B. P., Simpson, M. J., Simpson, A. J., and Frost, P. C. (2015). Metabolomic differentiation of nutritional stress in an aquatic invertebrate. Physiol. Biochem. Zool. 88, 43–52. doi: 10.1086/679637
Weiße, A. Y., Oyarzún, D. A., Danos, V., and Swain, P. S. (2015). Mechanistic links between cellular trade-offs, gene expression, and growth. Proc. Natl. Acad. Sci. U.S.A. 112, E1038–E1047. doi: 10.1073/pnas.1416533112
Wojewodzic, M. W., Kyle, M., Elser, J. J., Hessen, D. O., and Andersen, T. (2011). Joint effect of phosphorus limitation and temperature on alkaline phosphatase activity and somatic growth in Daphnia magna. Oecologia 165, 837–846. doi: 10.1007/s00442-010-1863-2
Keywords: Daphnia physiology, ecological stoichiometry, diet heterogeneity, P metabolite pools
Citation: Wagner ND, Prater C and Frost PC (2017) Dynamic Responses of Phosphorus Metabolism to Acute and Chronic Dietary Phosphorus-Limitation in Daphnia. Front. Environ. Sci. 5:36. doi: 10.3389/fenvs.2017.00036
Received: 01 February 2017; Accepted: 12 June 2017;
Published: 29 June 2017.
Edited by:
Dedmer B. Van de Waal, Netherlands Institute of Ecology (NIOO-KNAW), NetherlandsReviewed by:
Cedric Leo Meunier, Alfred Wegener Institute, GermanySteven Declerck, Netherlands Institute of Ecology (NIOO-KNAW), Netherlands
Copyright © 2017 Wagner, Prater and Frost. This is an open-access article distributed under the terms of the Creative Commons Attribution License (CC BY). The use, distribution or reproduction in other forums is permitted, provided the original author(s) or licensor are credited and that the original publication in this journal is cited, in accordance with accepted academic practice. No use, distribution or reproduction is permitted which does not comply with these terms.
*Correspondence: Nicole D. Wagner, bmljb2xlZ291bGRpbmd3YWduZXJAZ21haWwuY29t
†Present Address: Nicole D. Wagner, Department of Environmental and Physical Science, University of Toronto, Toronto, ON, Canada