- 1Centre of Advanced Study in Botany, Banaras Hindu University, Varanasi, India
- 2Centre for Medical Diagnostic and Research, Motilal Nehru National Institute of Technology, Allahabad, India
- 3D. D. Pant Interdisciplinary Research Laboratory, Department of Botany, University of Allahabad, Allahabad, India
- 4Division of Plant Sciences, University of Missouri, Columbia, MO, United States
- 5Government Ramanuj Pratap Singhdev Post Graduate College, Baikunthpur, India
- 6Department of Biotechnology, Motilal Nehru National Institute of Technology Allahabad, Allahabad, India
- 7Amity Institute of Environmental Sciences, Amity University Uttar Pradesh, Noida, India
- 8Ranjan Plant Physiology and Biochemistry Laboratory, Department of Botany, University of Allahabad, Allahabad, India
- 9Department of Biological Sciences, University of the Sciences, Philadelphia, PA, United States
Iron (Fe) is a micronutrient that plays an important role in agriculture worldwide because plants require a small amount of iron for its growth and development. All major functions in a plant's life from chlorophyll biosynthesis to energy transfer are performed by Fe (Brumbarova et al., 2008; Gill and Tuteja, 2011). Iron also acts as a major constituent of many plant proteins and enzymes. The acquisition of Fe in plants occurs through two strategies, i.e., strategy I and strategy II (Marschner and Römheld, 1994). Under various stress conditions, Nramp and the YSL gene families help in translocation of Fe, which further acts as a mineral regulatory element and defends plants against stresses. Iron plays an irreplaceable role in alleviating stress imposed by salinity, drought, and heavy metal stress. This is because it activates plant enzymatic antioxidants like catalase (CAT), peroxidase, and an isoform of superoxide dismutase (SOD) that act as a scavenger of reactive oxygen species (ROS) (Hellin et al., 1995). In addition to this, their deficiency as well as their excess amount can disturb the homeostasis of a plant's cell and result in declining of photosynthetic rate, respiration, and increased accumulation of Na+ and Ca− ions which culminate in an excessive formation of ROS. The short-range order hydrated Fe oxides and organic functional groups show affinities for metal ions. Iron plaque biofilm matrices could sequester a large amount of metals at the soil–root interface. Hence, it has attracted the attention of plant physiologists and agricultural scientists who are discovering more exciting and hidden applications of Fe and its potential in the development of bio-factories. This review looks into the recent progress made in putting forward the role of Fe in plant growth, development, and acclimation under major abiotic stresses, i.e., salinity, drought, and heavy metals.
Introduction
Abiotic stress is a result of several environmental disturbances caused by the continuous encroachment of industrialization, urbanization, and some human interference with the natural ecosystem that influence the quality and quantity of agriculture production per year (Mantri et al., 2012). Abiotic stresses include heat, cold, freezing, drought, salinity, flooding agents, UV, and heavy-metal stresses that have significant impacts on a plant life cycle (Macedo, 2012; Mantri et al., 2012; Singh et al., 2015, 2017; Tripathi et al., 2016c). These stresses not only affect plant biodiversity and productivity but also can interfere with the food web and the ecosystem. Abiotic stresses are worldwide problems as they decline the crop yield (Cramer et al., 2011). Moreover, the presence of heavy metals in food crops causes some severe diseases like cancer and asthma (Brigham et al., 2015). Plants, being the most precious gift of the nature that fulfill several basic requirements of human beings, are severely affected by various abiotic stress factors. Abiotic stress causes significant reduction in growth and yield of plants via inducing oxidative stress through enhanced reactive oxygen species (ROS) production and by lowering the antioxidant activities, level of nutrients, and modification of anatomical structures (Nagajyoti et al., 2010; Nazar et al., 2012; Vaculík et al., 2012; Singh et al., 2015; Tripathi et al., 2016b, 2017a). Hoagland and Arnon (1950) pioneered the most popular and commercial technique for developing plants with their roots in solutions containing mineral nutrients required for the growth of plants.
Various techniques are being used to protect plants from the adverse effects of abiotic stresses, which include exogenous supplementations of silicon, nitric oxide, growth-promoting hormones, enzymes, and nutrient management (Nagajyoti et al., 2010; Nazar et al., 2012; Tripathi et al., 2012, 2016a, 2017a,b,c,d; Vaculík et al., 2012; Saxena and Shekhawat, 2013). Among the remedies for abiotic stress, nutrient regulations or management are considered as the cost effective and eco-friendly techniques (Tripathi et al., 2015; Yadav et al., 2016). It has been reported that plant nutrients (micro and macro) play important roles in growth and development (White and Brown, 2010; Waraich et al., 2011; Tripathi et al., 2014, 2015). Therefore, an adequate and balanced supply of nutrients at the correct time is required for the proper growth and development of plants, maintenance of better soil fertility, and preservation of an intensive cropping system to maintain the global food production, particularly in developing countries (Dordas, 2009; Hansch and Mendel, 2009; Sarwar et al., 2010; Moharana et al., 2012; Waraich et al., 2012). In addition, studies also showed that an exogenous supply of nutrients plays a crucial role in the enhancement of plant tolerance against various abiotic stresses (Pankovic et al., 2000; Hassan et al., 2005; Tlustos et al., 2006; Dheri et al., 2007; Sarwar et al., 2010). Some nutrients such as calcium (Ca), magnesium (Mg), sulfur (S), zinc (Zn), and iron (Fe) have shown significant results when they are examined under salinity, drought, and heavy-metal stresses (Sarwar et al., 2010; Singh et al., 2011; Nazar et al., 2012). Currently, the application of Fe as a nutrient supplement and its role in imparting tolerance to plants against abiotic stresses are gaining attention as an area of research. Some studies suggested that the application of Fe reflects significant and/or potential impacts in alleviating the stress imposed by metal ions (Liu et al., 2007; Yadav et al., 2007; Garnier et al., 2010; Emamverdian et al., 2015).
Iron—the fourth most abundant and essential microelement on the earth's crust—is unavailable to higher plants, largely due to neutral and alkaline soils because of its existence in an insoluble form (Shao et al., 2007). In the plant system, Fe is regarded as an essential element in regulating life-sustaining processes like respiration, photosynthesis, chloroplast development, and chlorophyll biosynthesis where it takes part in electron transportation (Kim and Guerinot, 2007). Inadequate Fe supply in plants causes iron-deficiency, which results in decline in crop yields, interveinal chlorosis in plant leaves, etc. (Kim and Guerinot, 2007; Lan et al., 2011). In addition, excess levels of Fe can be fatal for plant health and productivity (Anjum et al., 2015). Therefore, an appropriate supply of Fe is needed for sustaining plant productivity under stress as well as non-stress conditions. It has been reported that out of the total cultivated areas of the world, one-third has Fe deficiency (Kim and Guerinot, 2007), which is causing significant decline in yield of crops every year. Iron is a chief component of the cell redox systems and also acts as a cofactor of various antioxidant enzymes like catalase (CAT), peroxidase (POD), and ascorbate peroxidase (APX) (Marschner, 1995; Sharma et al., 2004; Kumar et al., 2010). Therefore, Fe homeostasis plays a crucial role in the life cycle of plants under stressed conditions (Figure 1) (Gratao et al., 2005; Meda et al., 2007; Sarwar et al., 2010). In addition to overviewing major Fe acquisition strategies in plants, this review briefly appraises the literatures available on the role of Fe in plants exposed to major abiotic stresses like salinity, drought, and heavy metals. Less discussed topics in the current context have also been highlighted.
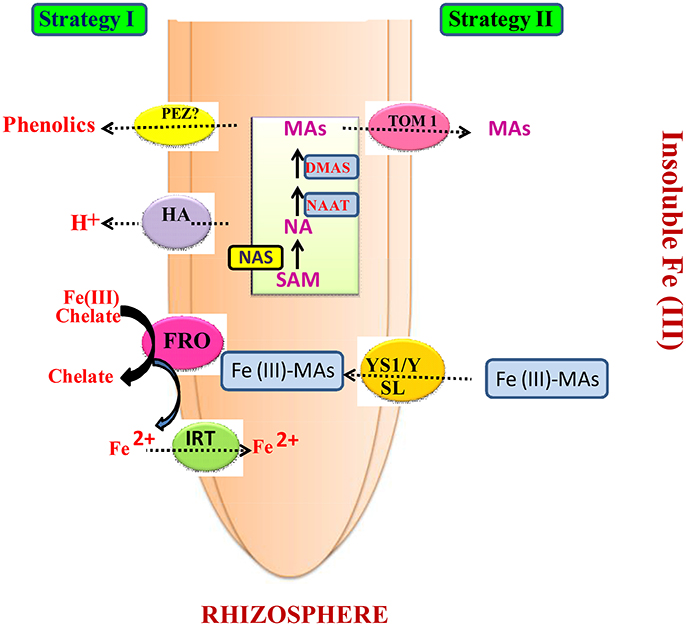
Figure 1. Different Strategies adopted by higher plant for Iron Acquisition. Strategy I occurs in the members of non-graminaceous plants (Left) and Strategy II occurs in the members of graminaceous plants (Right). Ovals corresponds the transporters and enzymes involved that play significant roles in both the strategies. The expression of these genes shown in the present figure occurs in response to Fe deficiency (Modified from; Kobayashi and Nishizawa, 2012).
Iron Acquisition Strategies in Plants
Despite being categorized as the fourth most abundant element of the earth's crust, the availability of Fe to plants is highly heterogeneous as it has low solubility under aerobic conditions, particularly at high pH and in calcareous soil. Plants firmly regulate the homeostasis of Fe and respond to the shortage as well as the surplus level of Fe (Morrissey and Guerinot, 2009). Therefore, in order to maintain proper ion homeostasis, plants stabilize the uptake, transport, and storage (Grotz and Guerinot, 2006). Hence, for the significant acquisition and agglomeration of Fe, plants have developed two different strategies (strategy I and II) under varied soil conditions (Römheld and Marschner, 1986) (Figure 1). Almost all higher plants except the members of family Gramineae follow Strategy I for Fe uptake (Kobayashi and Nishizawa, 2012) (Figure 1). The uptake and accumulation of Fe is frequently enhanced by three reactions: firstly, the secretion of protons through the plasmalemma P-type ATPase in order to acidify the surrounding solution for augmenting the solubility of Fe3+; secondly, by reducing Fe3+ to the more soluble Fe2+ form through Fe3+ chelate reductase; and thirdly, by plasmalemma transport of Fe2+ through Fe transporters (Figure 1). The dominant gene essential for these processes was first cloned from Arabidopsis thaliana and peas such as FRO2, allelic to the frd1 mutation distressing Fe3+-chelate reductase activity (Robinson et al., 1999), and FRO1, respectively (Waters et al., 2002). Furthermore, reduced form of iron in the plants transported from the root via IRT1 (divalent cation transporter) (Eide et al., 1996; Eckhardt et al., 2001), which is a member of the ZIP family (Guerinot, 2000). In addition, IRT1 is also reported as an essential gene because, in the case of excess supplementation of exogenous iron, it reduces the lethality of seedlings and suppresses the chlorosis in irt 1 mutants (Henriques et al., 2002; Varotto et al., 2002; Vert et al., 2002). Moreover, Vert et al. (2002) and Dinneny et al. (2008) observed that the acquisition of Fe starts from the epidermal layers of the root, which is proven by the expression pattern of IRT1 and FRO2. The transporter gene IRT1, which is responsible for Fe transport, has been cloned from Arabidopsis (Eide et al., 1996; Dubeaux et al., 2015) and its ortholog RIT1 has been cloned from pea and tomato as well (Cohen et al., 1998; Eckhardt et al., 2001). Fe3+-chelate reductases are integral membrane-bound proteins and they belong to the family of proteins that are responsible for the transport of electrons from cytosolic NADPH to FAD and, consequently, through heme groups to electron acceptors located on the outer side of the plasma membrane (Figure 1). In response to Fe deficiency, the up-regulation of the FRO gene has been noted in roots (Robinson et al., 1999; Waters et al., 2002). On the basis of the recent studies, IRT1 has been marked as the major transporter gene responsible for the uptake of Fe from soil solutions (Figure 1). Besides this, IRT1 also plays significant role in the transport of Zn, Mn, Co, and Cd (Rogers et al., 2000). Similarly, PEZ1 is a novel effluxer responsible for the xylem loading of phenolics as well as the remobilization of precipitated apoplasmic Fe in the plant cell. This is due to the fact that FRD3, FRDL1, and PEZ1 are efflux Fe-chelating molecules in their Fe liberated forms and Fe efflux occurs in the xylem sap by more than one transporters (Ishimaru et al., 2011). Morrissey et al. (2009) described the role of ferroportin 1/iron regulated 1 (AtFPN1/AtIREG1) metal efflux protein function in Fe homeostasis in Arabidopsis plants, which is similar to the iron efflux transporter in mammals and are responsible for Fe absorption in the intestines and Fe recycling in macrophages. While the active role of AtFPN1 localized on the plasma membrane has not yet been reported, its promoter activity is prominently seen in the stele region of plants. In addition, its mutant species showed the loss of chlorophyll content in both Fe-sufficient and Fe-deficient media, which signify its indispensable role in acquisition of Fe to shoots (Morrissey et al., 2009) as well as mitochondrial Fe metabolism and trafficking progress via the mitochondrial Fe storage protein (mitochondrial ferritin) and transport (mitoferrin-1 and -2) (Richardson et al., 2010).
Strategy II is exclusively specific to the Gramineae family and is based on the biosynthesis and secretion of mugineic acids (MAs) (Figure 1). The roots of graminaceous plant release phytosiderophores (PSs), which are responsible for the chelation of Fe3+ in the rhizosphere. Specific transporter proteins located on the plasma membrane transport Fe3+ PS complexes in the cytosol (Römheld and Marschner, 1986). In response to Fe deficiency, both processes are enhanced through up-regulation of the underlying genes (Figure 1). Until now, nine different kinds of MAs have been recognized, which have been produced via a conserved pathway from S-adenosyl-L-methionine (Bashir et al., 2006; Ueno et al., 2007; Kobayashi and Nishizawa, 2012). Through sequential enzymatic reaction mediated by the precursors of MAs such as NAS, NAAT, DMAS, and DMA, strategy II enhanced acquisition of Fe in graminaceous plant (Figure 1). Normally, the transportation of Fe in plants may be seen in the anatomical structures of roots when observed under the Phase-contrast microscopy. It can be well observed that the deposition percentage of Fe in root cells is increased by the increased level of Fe concentration.
After the acquisition of Fe inside the root, its translocation occurs across the plasma membrane through several different members of transporter families that are responsible for the transportations of intra- and intercellular ions (Figure 2). Such transporters include Nramp and YSL families (Grotz and Guerinot, 2006). The Nramp family leads the translocation of divalent cations, whereas the members of the YSL family are probably only responsible for the translocation of metal chelates (Conte and Walker, 2011). Members of the NRAMP family are responsible for the transportation of an array of metals across the plasma membranes including Mn2+, Zn2+, Cu2+, Fe2+, Cd2+, Ni2+, and Co2+ in microorganisms, plants, and animals (Nevo and Nelson, 2006). In plants, the expression of Nramp transporters has been located in roots and shoots and is responsible for the transportation of metal ions across plasma membranes and the tonoplast (Krämer et al., 2007). In A. thaliana, NRAMPs facilitate the transport of Fe and Cd. NRAMP1 displays a significant role in the transport and homeostasis of Fe. Besides this, members of the YSL family mediate the acquisition of Fe through the translocation of Fe3+ phytosiderophore complexes (Curie et al., 2001). In addition, CDF transporters have also been recognized recently that lead to the transportation of metal ions (in divalent form) in prokaryotes (Nies, 1992) and also in several eukaryotes (Montanini et al., 2007). The apoplast sometime plays an important role in ion uptake into the cells and also performs some important physiological roles in nutrient transport and storage (Sattelmacher and Horst, 2007).
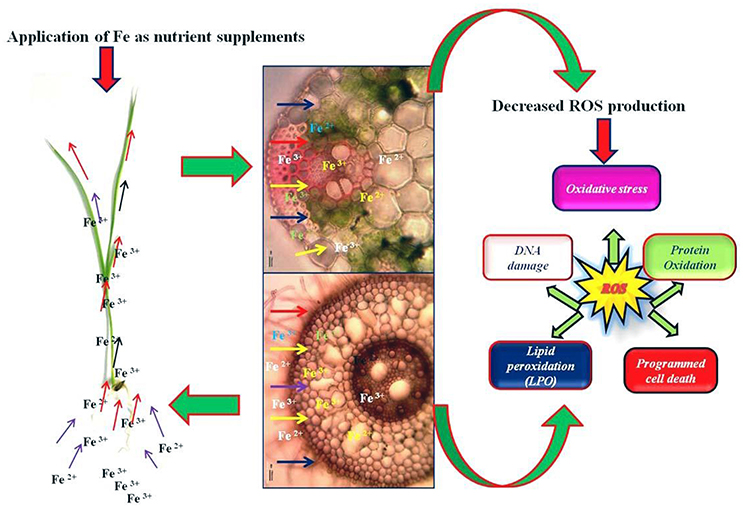
Figure 2. Hypothetical respesentation of exogenous application of Fe and its accumulation from root to shoot, which ultimately decreases the over production of oxidative stress level in plants caused by abiotic stresses.
In addition to this, Deiana et al. (1992) studied homeostasis, kinetics, and the consequences of mechanisms of Fe uptake in plants. Römheld and Marschner (1983) reported that when Fe was found in very high concentrations, caffeic acid plays a very active role in Fe mobilization at the interface of the soil-root. Caffeic acid is the phenolic compound produced from plants (Whitehead et al., 1981, 1983; Olsen et al., 1982; Wei Jin et al., 2008) that functions in the reduction of Fe3+ and takes part in redox reactions near rhizosphere and free spaces (Brown and Ambler, 1973; Olsen et al., 1981; Deiana et al., 1992). Deiana et al. (1992) reported that the concentration of Fe3+ is directly proportional to the yield of Fe2+, i.e., on increasing the Fe3+ concentration. The yield of Fe2+ also increased the value higher than 9 for Fe(III)/CAF molar ratios. Deiana et al. (1992) also found that one molecule of the phenolic plant product (CAF) is capable of reducing the nine electrons of the Fe3+ ion to the Fe2+ ion, and they also demonstrated that the whole reaction mechanism involves two steps. The first one includes the formation of the Fe3+ ion from organic molecules. This reaction is faster and involves the transfer of five electrons, whereas the second one includes the transfer of four electrons with a comparatively slower reaction (Deiana et al., 1992).
Iron and Abiotic Stress Tolerance in Plants
Iron and Salinity Stress
Salinity is one of the most challenging issues of the present time that poses great constraint to agricultural productivity worldwide (Ashraf, 1994; Khan and Gulzar, 2003; Asraf and Harri, 2004; Parihar et al., 2015). Salinity effects are more pronounced in arid and semiarid regions of the world (Bradbury and Ahmad, 1990). It has been estimated that approximately 20% of the total cultivated land is affected by salt stress annually. Likewise, nearly half of the world's irrigated land is being affected by salinity and water-logging conditions (FAO, 2008; Munns and Tester, 2008; Talei et al., 2012). Furthermore, agricultural practices without appropriate water management are another problem that is responsible for extensive salinization in crop lands (Taiz and Zeiger, 2006).
Plant exposure to high salinity induces several detrimental effects on plants as it triggers a wide range of changes at physiological, biochemical, and molecular levels (Tester and Davenport, 2003; Khan et al., 2009). Ion toxicity, nutrient deficiency, and oxidative and osmotic stresses are among the major physiological changes that are primarily being affected by the salt stress in plants (Flowers et al., 1977). Nonetheless, growth stages of plants also get badly hampered by salinity stress (Sairam and Tyagi, 2004; Jithesh et al., 2006). Furthermore, elevated salt concentration in the soil also reduces the osmotic potential that results in disturbed water availability to the root cell. Therefore, it becomes difficult for the plant to acquire both minerals as well as water from the soil (Talei et al., 2011). Rapid alterations in growth, productivity, and the metabolic processes of plants are being observed because of the hormonal signals produced inside root cells (Munns, 2002; Asraf and Harri, 2004).
In addition, salinity reduces the photosynthetic rate, growth, and development of plants, and is also associated with ionic or nutrient imbalance in plant cells (Nazar et al., 2011). Salinity-induced reductions in photosynthesis might involve the excessive accumulation of leaf Na+ and Cl− ions, the stomatal and non-stomatal limitations, and the hindrance of biochemical processes and oxidative damage due to the formation of ROS (Steduto et al., 2000) (Figure 2). Excess accumulation of ROS in plants produces several deleterious effects such as membrane lipid peroxidation, DNA damage, protein oxidation, chloroplast damage, and inhibited biochemical processes (Gunes et al., 2007; Sharma et al., 2012) (Figure 2). Moreover, extreme salinity leads to the ultimate death of the plant cell (Zhu et al., 1997; Xiong and Zhu, 2002). However, a plant's defense mechanisms against salinity comprise osmotic changes, salt separation functions in the cell, and other morphological modifications.
Data analyses based on previous findings showed that salinity also reduces the acquisition of nutrients inside the plant and affects their partitioning (Rabhi et al., 2007; Heidari et al., 2013). Chlorosis might be the unfortunate consequence of the limited Fe supply in plants under salinity (Yousfi et al., 2007). Several studies showed adverse effects of salinity on Fe acquisition in plants.
Hassan et al. (1970), Dahiya and Singh (1976) and Okcu et al. (2005) showed the adverse effect of salinity on the accumulation of Fe content in the shoots of barley, corn, and pea. Furthermore, Heidari and Sarani (2012) reported adverse effects of salinity in the chamomile plant, including stunted growth and a reduction in biochemical components and ion content. Yousfi et al. (2007) have also reported deleterious effect of salt on the physiological processes of barley due to the disturbance in Fe acquisition in plants caused by reducing the flow of phytosiderophore. Now, it is well documented that salinity and Fe interaction in plants result in reduction of salt toxicity.
In the last few decades, extensive studies have been carried out that showed a significant ameliorative effect of Fe against salinity. In reducing the salt stress, Fe plays a unique role by producing antioxidative enzymes (Sharma et al., 2012; Ghasemia et al., 2014). These antioxidative enzymes include catalase (CAT), peroxidases (PODs), and one isoform of superoxide dismutase (SOD) that act as major scavengers of ROS, thereby enhancing cell defense mechanisms against salinity (Scandalios, 1990). However, Manthey et al. (1996) reported decreased activity of both CAT and SOD enzymes under Fe-deficient conditions in onion, which was associated with increased susceptibility against stress. Ghasemia et al. (2014) suggested defending the role of Fe2+ amino acid chelates in tomato plants against salinity. Hence, from the above studies, it can be concluded that Fe plays a significant role in alleviating the adverse effects of salinity.
Iron and Drought Stress
Due to the scarcity of water resources, drought is recognized as one of the single most critical threats to world food security (Abolhasani and Saeidi, 2004; Lambers et al., 2008; Farooq et al., 2009, 2012; Moghadam et al., 2011; Monjezi et al., 2013; Pourgholam et al., 2013). It harms plant growth and development and reduces the growth rates of crop and biomass accumulation. Generally, in crop plants, drought severely affects cell division and expansion, elongation of root, leaf size, proliferation of root, and inhibition of shoot growth (Sharp and Davies, 1989; Spollen et al., 1993; Sharp et al., 2004; Yamaguchi et al., 2010). Furthermore, it hampers all kinds of physiological and biochemical traits such as mineral elements, carbohydrates, ions, hormones, lipids, and nucleic acids (HongBo et al., 2005; Yasar et al., 2006; Moghadam et al., 2011; Pourgholam et al., 2013). The transportation of nutrients from root to shoot are severely affected by drought as the rate of transpiration is reduced. It consequently hampers the active transport of ions and damages the membrane permeability of cells (Viets, 1972; Alam, 1999; Yasar et al., 2006). Simultaneously, due to decrease in soil moisture, a problem occurs with the low distribution of absorbed nutrients in the root of plants in the soil (Alam, 1999; Yasar et al., 2006). More importantly, ROS are generated more frequently due to drought as a result of alterations in electron transport systems (Smirnoff, 1993; Asada, 2006; Waraich et al., 2011). Drought diminishes photosynthetic carbon fixation primarily through restricting the entrance of CO2 into the leaf or by reducing the metabolism (Smirnoff, 1993; Loggini et al., 1999; Apel and Hirt, 2004; Waraich et al., 2011) (Table 1). In addition, stomatal content oscillations and water use efficiency are affected (Farooq et al., 2009, 2012; Li et al., 2009). Stomatal density also deteriorate under severe drought conditions (Xu and Zhou, 2008; De Micco and Aronne, 2012). Photosynthetic pigments and, consequently, photosynthesis are also severely affected by drought (Iturbe-Ormaetxe et al., 1998; Gong et al., 2005). Drought stress reduces chlorophyll a and chlorophyll b contents in marigold (Asrar and Elhindi, 2011; Farooq et al., 2012). It leads to disturbances in water uptake during the imbibition phase of germination, decreases energy supply, and affects enzyme activities, which diminishes the process of germination (Taiz and Zeiger, 2010; Farooq et al., 2012). Drought stress causes generation of numerous signals such as the production of abscisic acid (ABA) in root tissues (Wang et al., 1999; Aroca and Ruiz-Lozano, 2012) or some hydraulic signals passed through the root axis (Aroca and Ruiz-Lozano, 2012; Ionenko et al., 2012).
To survive under drought condition, the nutrient status of plants plays a significant role in increasing plant tolerance to drought stress (Table 1) (Payne et al., 1992; Marschner, 1995; Rizhsky et al., 2004; Samarah et al., 2004; Yasar et al., 2006; Rotaru and Sinclair, 2009; Rotaru, 2011; Waraich et al., 2011). A study showed that sunflower develops drought resistance with the help of application of micronutrients (Rahimizadeh et al., 2007). It has been demonstrated that the application of Fe nutrition to plants under drought condition can enhance tolerance as it leads to production of assimilates (Sultana et al., 2001; Khan et al., 2003; Rotaru, 2011; Pourgholam et al., 2013) (Table 1). It has also been reported that legumes have positive responses to Fe nutrition (Slatni et al., 2008; Rotaru, 2011). Furthermore, Mahmood et al. (1990) demonstrated that the application of Fe increased the yield of wheat plants. Elanz et al. (2011) recommended Fe foliar application on sunflower under drought stress, which showed that Fe foliar application under normal and stressed conditions plays important roles in seed and oil production (Table 1). Fe foliar application improved soybean yield and also leads to the intensification of seed set in the wheat crop (Arif et al., 2006; Kobraee et al., 2011; Afshar et al., 2013). Akbari et al. (2013) suggested that Fe foliar application with Zn diminishes oxidative stress by reducing H2O2 content and lessening lipid peroxidation by enhancing antioxidant enzymes (CAT, GPX, and SOD) under drought stress (Table 1). It was also proposed that over-expression of Fe-SOD helps in the reduction of secondary injury symptoms and leads to enhancement in drought tolerance (McKersie et al., 1999, 2000; Alscher et al., 2002; Samis et al., 2002). Pirzad and Shokrani (2012) applied Fe with Zn to improve the leaf characters (weight, area, and numbers) of Calendula officinalis under drought stress. Reductions in terms of either vegetative growth or oil contents in sunflower due to drought conditions were found to be alleviated by the foliar applications of Fe (Ebrahimian and Bybordi, 2011). Mostafa et al. (2011) examined the effect of Fe application with surface water stress conditions on growth, yield, and nutrient uptake of Sesamum indicum L. plants (Table 1). Iron spraying plays a significant role in improving the protein quality and resistance under drought stress (Parhamfar, 2006; Afshar et al., 2012). It is important to mention that Fe is applicable in controlling drought effects on root growth (Snyder and Schmidt, 1974; Glinski et al., 1992). Under drought stress and Fe-deficient conditions, the application of Fe over turf grasses leads to color enrichment and growth improvement (Deal and Engel, 1965; Minner and Butler, 1984; Glinski et al., 1992). Moreover, in Fe-sufficient condition, Fe has been applied to give a darker green color for cool-season turf grass (Snyder and Schmidt, 1974; Yust et al., 1984; Carrow et al., 1988; Wehner and Haley, 1990; Glinski et al., 1992). There is still much more investigative work required to understand the role of Fe against drought stress (Table 1).
Iron and Heavy Metal Stress
Rapid technical development in different sectors of agriculture, industries, and anthropogenic activities have resulted in an abundant release of heavy metals (Young et al., 2009; Oliveira, 2012; Kumar et al., 2013). Metals in the form of pollutants pose serious threats to our environment. They exhibit toxicity in the natural soil that harbors vegetation (Foy et al., 1978; Yizong et al., 2009). The excessive use of phosphatic fertilizers, industrial wastes, and sewage sludge applications release several toxic heavy metals/metalloid such as Cd, Cr, Pb, and As into agricultural soil (Bell et al., 2001; Schwartz et al., 2001; Passariello et al., 2002; Yadav, 2010). Later, these heavy metals enter into the ecosystem and food chain through absorption and accumulation processes in plants and animals, affecting them severely (Yamagata and Shigematsu, 1970; Cervantes et al., 2001; Vernay et al., 2007; Yizong et al., 2009; Kumar et al., 2013). For example, the havoc of “Itai-Itai disease” in Japan was due to presence of Cd contamination in rice and soybean (Yamagata and Shigematsu, 1970; Yizong et al., 2009; Kumar et al., 2013; Tchounwou et al., 2013).
Plants, undoubtedly, are being affected by heavy metal contaminations as numerous morphological, physiological, and biochemical changes have been noticed in metal toxicity-challenged plants (Pandey et al., 2005; Oliveira, 2012; Kumar et al., 2013; Singh et al., 2016). The major damaging effects of heavy metal stress in plants are lipid peroxidation (which is an indicator of biomembrane deterioration) and a variation in enzymatic and transport activities (De Vos et al., 1989, 1991b; De Vos and Schat, 1991a; Pandolfini et al., 1992; Somashekariah et al., 1992; Sinha et al., 1997; Yadav, 2010). Several metals such as Hg, Cd, and Cu affect the permeability of the plasma membrane in the plant cell, which consequently results in a loss of K ions (De Vos et al., 1989, 1991b; De Vos and Schat, 1991a; Pandolfini et al., 1992; Reddy and Prasad, 1992; Sinha et al., 1997). Due to high affinity toward cellular sulfhydryl groups, heavy metals also give rise to sulfhydryl reactions (De Vos et al., 1989; Sinha et al., 1997). Nonetheless, heavy metals in plants cause overproduction of ROS, which generate oxidative stress (Wojtaszek, 1997; Mithofer et al., 2004; Yadav, 2010). Consequently, oxidative stress creates membrane damage (Srivastava et al., 2004; Yadav, 2010) due to the unbalanced antioxidant defense system in plants (Demiral and Turkan, 2005; Yadav, 2010). Heavy metal stress in plants also deteriorates the metabolism of essential elements and severely affects the electron transport chain (Table 2) (Qadir et al., 2004; Dong et al., 2006; Yadav, 2010; Singh et al., 2016).
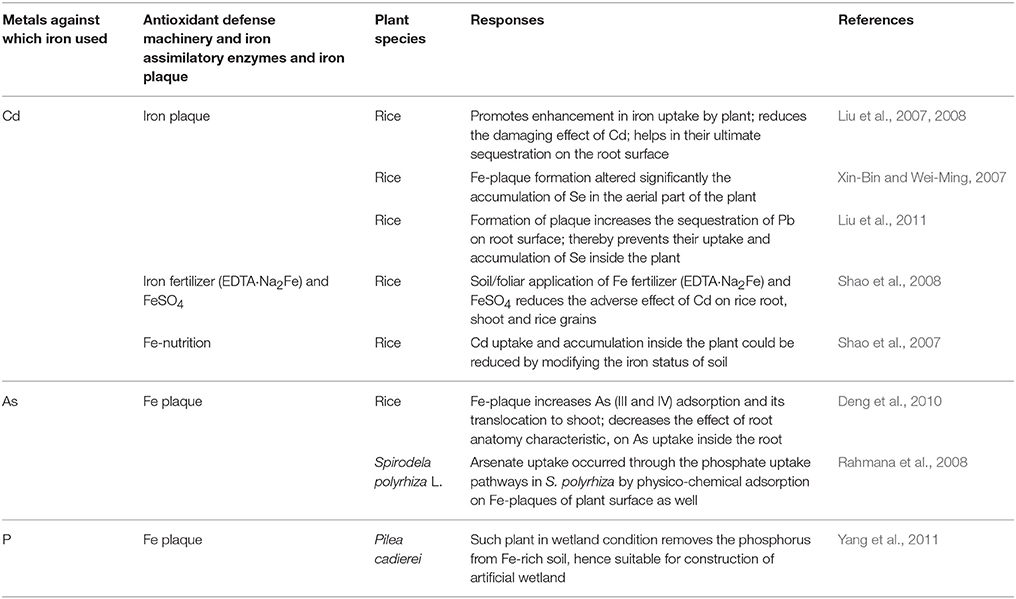
Table 2. Iron-mediated up-regulation of antioxidative enzymes (SOD, APX, and CAT) and heavy-metal stress tolerance.
To cope with heavy metal stress, plants adopt several strategies at various levels (Table 2). Of these, the management of mineral nutrients was found to be the most efficient mechanism (Cakmak, 2005). Among mineral nutrients, Fe is being recognized as the most efficient and potentially essential micronutrient that plays a pivotal role in mitigating metal stress (Table 2). Several reports showed the efficacy of Fe in controlling the damaging effects of metal stress (Emamverdian et al., 2015). Iron is considered as a biologically important micronutrient as it serves as the major constituent of the cell redox systems such as heme proteins, including leg hemoglobin, catalase, cytochromes, peroxidase, and Fe-S clusters such as aconitase, ferredoxin, and superoxide dismutase (SOD) (Marschner, 1995; Emamverdian et al., 2015).
Moreover, the formation of aconitase plaque by ferrous oxidation to ferric iron on the root surface of terrestrial and aquatic plants helps in the sequestration of a large number of metal through adsorption or co-precipitation and thereby inhibits the uptake and accumulation of metal inside the plant cell (Armstrong, 1964, 1967; Bacha and Hossner, 1977; Chen et al., 1980; Mendelssohn and Postek, 1982; Davison and Seed, 1983; Taylor and Crowder, 1983; Taylor et al., 1984; Levan and Riha, 1986; Greipsson and Crowder, 1992; Emerson et al., 1999; Hansel et al., 2001; Weiss et al., 2003; Emamverdian et al., 2015).
Garnier et al. (2010) described the role of Fe plaque in a paddy field irrigated with ground water and suggested the significant role of plaque formation in attenuating the uptake and accumulation of As in rice plants (Table 2). Liu et al. (2007) also presented evidence for the role of Fe plaque in Cd adsorption on the root surface of the rice seedling (Table 2). Xin-Bin and Wei-Ming (2007) demonstrated the effect of Fe plaque on Se translocation and also showed its altered accumulation in the aerial part of the plant. Rahmana et al. (2008) used Fe with phosphate for the regulation of As toxicity in the water fern (Table 2). Yizong et al. (2009) have demonstrated that Fe plaque plays an important role in regulating Cd, Cu, and Pb toxicities in rice plants. Yang et al. (2011) showed significance of Fe in Pilea cadierei against phosphorus (P).
Consequences of Iron Toxicity and Redox Regulation in Plants
Plants may undergo Fe toxicity in two conditions: either excessive amount of Fe or Fe deficiency. Iron plays key roles in plant metabolism like electron transport systems (redox reactions of Fe-S proteins and cytochromes), respiration, photosynthesis, and nitrogen fixation processes (Soyder and Schmidt, 1974; Carrow et al., 1988; Taiz and Zeiger, 1991). Furthermore, the deficiency of Fe can affect about one-third of the cultivated land. Deficiency of Fe also causes the declination of photosynthetic components, especially Fad (Fe-S protein ferredoxin) of the chloroplast (Tognetti et al., 2007). Fe deficiency in plants causes interveinal chlorosis (Bienfait and Van der Mark, 1983), poor root development, growth retardation, and the eventual death of the plant (Kobayashi et al., 2003). In addition, Fe deficiency also leads to the alteration in expression of chlorophyll-binding proteins and the down-regulation of many photosynthetic pigment levels (Thimm et al., 2001; Rout and Sahoo, 2015). In the agricultural soils, Fe deficiency may also occur either at extremely high pH or at extremely low pH levels. López Jiménez et al. (1985) demonstrated that the increased levels of chlorosis in young Avocado leaves was correlated with a decline in the level of Fe in leaf parts, chloroplast numbers, and activity of catalase (Rout and Sahoo, 2015). The Fe deficiency is also characterized by interveinal chlorosis in young leaves rather than main veins, a type of symptom known as “iron deficiency chlorosis” (Rout and Sahoo, 2015). Similarly, excessive amounts of Fe also cause toxicity in plants. Tanaka et al. (1966) demonstrated that upon increasing the level of Fe, roots are affected more than leaves. It shows that in the presence of an excess amount of Fe, plant roots increase their capability and translocate the Fe in younger leaves. High level of Fe disturb the basal level of magnesium and potassium in plants and cause nutritional disturbances (Tanaka et al., 1966).
Although high concentrations of Fe can lead to toxic consequences in plants (Anjum et al., 2015). Excess free Fe ions like Fe2+ and Fe3+ can cause ROS generation by participating in the Fenton reaction (Fenton, 1894; Haber and Weiss, 1934; Kehrer, 2000; Mai and Bauer, 2016) and leading to oxidative stress (Mai and Bauer, 2016). Down regulated detoxifying proteins in Fe-deprived conditions, viz. CAT2 (CATALASE 2; AT4G35090) proteins and PA2 (PEROXIDASE 2; AT5G06720) proteins, were reported in some plants (Donnini et al., 2010; Rodríguez-Celma et al., 2011). These are enzymes that help in the conversion of H2O2 (hydrogen peroxide) to H2O (water) and oxygen. In addition, Fe deficiency in the roots of M. truncatula (Rodríguez-Celma et al., 2011) and P. dulcis × P. persica (Rodriguez-Celma et al., 2013) was characterized by the superoxide dismutase, i.e., ATMSD1 (ARABIDOP-SIS MANGANESE SUPEROXIDE DISMUTASE 1; AT3G10920) (Mai and Bauer, 2016). Furthermore, non-enzymatic ROS generation also occurs due to Fe deficiency. In this process, two enzymes reported in the roots (Mai et al., 2015) and shoots (Zargar et al., 2013) of Arabidopsis thaliana were GST1 (ARABIDOPSIS GLUTATHIONE S-TRANSFERASE 1; AT1G02930) and MDAR1 (MONODEHYDROASCORBATE REDUCTASE 1; AT3G52880) (Rodríguez-Celma et al., 2011; Mai et al., 2015). In the case of Fe deficiency, two heme-binding proteins (CAT2 and PA2) were found to decrease the level of peroxidases. Moreover, ATMSD1 is a ROS-eliminating enzyme formed after the up-regulation of ROS-eliminating enzyme and beneficial in compensation of deprivation of Fe-dependent peroxidases and stimulated the ascorbate-glutathione cycle from GPX3 (GLUTATHIONE PEROXIDASE3; AT2G43350) (Mai et al., 2015). GST1 and MDAR1 were reported to detoxify the ROS generated due to Fe deficiency (Apel and Hirt, 2004).
Conclusion and Future Outlook
Increased anthropogenic activities and technologies have rendered a polluted environment. Furthermore, the situation is likely to be much worsened by an immense increase in human population that is expected to reach about 9.1 billion by 2050 according to the report of the FAO (2008), which would result in decreased areas of arable land for future agricultural practices. It is well documented that approximately 50% of the world's agricultural land suffers from adverse effects of complex environmental stress factors such as salinity, drought, and heavy metal. Since plants are restricted in their movements, they are inevitably exposed to such stressful conditions that would cause reduction in their yields. To counteract inhibitory/adverse effects of such stresses, plants employ several efficient and sophisticated biochemical defense mechanisms at multiple levels (morphological, anatomical, biochemical, and molecular). Data analyses based on previous findings showed tremendous progress at various levels against stress factors in plants. Of these strategies, management of mineral nutrient status in plants was found to be the most promising. Among mineral nutrients, Fe is regarded as one of the most efficient and essential micronutrients that acts significantly in a number of cellular processes such as metal detoxification, metabolism of secondary metabolites, and maintenance of the cell redox cycle. Previous findings suggested that Fe regulates adverse effects of salinity, drought, and heavy metal by controlling the redox status of the cell and antioxidant defense system. In addition, the formation of Fe plaque also facilitates the sequestration of a large number of metals on the root surface. Although not much is known about the relevancy of Fe nutrition in the mitigation of different stresses, its exogenous application, however, definitely regulates toxicity of several stresses. Despite major progress achieved in the field of Fe nutrition-mediated alleviation of stress, there are several questions still awaiting for the answers. In conclusion, it may be stated that uptake, accumulation, and metabolism of Fe inside the plant still deserve attention and may shed insight into the process of modifying agricultural productivity in stressful environments.
Author Contributions
DKT, ShwetaS, and SwatiS: designed the manuscript; DKT, ShwetaS, SG, and SwatiS: wrote the manuscript; VS, DC, PS, ShiveshS, VY, ND, SL, ShivendraS and SP: critically evaluated the manuscript.
Conflict of Interest Statement
The authors declare that the research was conducted in the absence of any commercial or financial relationships that could be construed as a potential conflict of interest.
Acknowledgments
The authors are grateful to the University Grants Commission, New Delhi, for financial assistance. DKT and SG is also grateful to the UGC for providing the Dr. D. S. Kothari Fellowship and National Fellowship for Higher Education (NFHE).
Abbreviations
DMAS, deoxymugineic acid synthase; FRO, ferric-chelate reductase oxidase; HA, H+-ATPase; IRT, iron-regulated transporter; MAs, mugineic acid family phytosiderophores; NA, nicotianamine; NAAT, nicotianamine aminotransferase; NAS, nicotianamine synthase; PEZ, PHENOLICS EFFLUX ZERO; SAM, S-adenosyl-L-methionine; TOM1, transporter of mugineic acid family phytosiderophores 1; YS1/YSL, YELLOW STRIPE 1/YELLOW STRIPE 1–like.
References
Abolhasani, K., and Saeidi, G. (2004). Relationships between agronomic characteristic of safflower under water stress and control. Iran. J. Field Cops Res. 127–138.
Afshar, R. M., Hadi, H., and Pirzad, A. (2012). Effect of Nano-iron foliar application on qualitative and quantitative characteristics of cowpea, under end season drought stress. Int. Res. J. Appl. Basic Sci. 3, 1709–1717.
Afshar, R. M., Hadi, H., and Pirzad, A. (2013). Effect of nano-iron on the yield and yield component of cowpea (Vigna unguiculata) under end season water deficit. Int. J. Agric. Res. Rev. 3, 27–34.
Akbari, G. A., Amirinejad, M., Baghizadeh, A., Allahdadi, I., and Shahbazi, M. (2013). Effect of Zn and Fe foliar application on yield, yield components and some physiological traits of cumin (Cuminum cyminum) in dry farming. Int. J. Agron. Plant Prod. 4, 3231–3237.
Alam, S. M. (1999). “Nutrient uptake by plants under stress conditions,” in Handbook of Plant and Crop Stress, ed M. Pessarakli (New York, NY: Marcel Dekker), 285–314.
Alscher, R. G., Erturk, N., and Heath, L. S. (2002). Role of superoxide dismutases (SODs) in controlling oxidative stress in plants. J. Exp. Bot. 53, 1331–1341. doi: 10.1093/jexbot/53.372.1331
Anjum, N. A., Singh, H. P., Khan, M. I. R., Mason, A., Per, T. S., Negi, A., et al. (2015). Too much is bad—an appraisal of phytotoxicity of elevated plant-beneficial heavy metal ions. Environ. Sci. Pollut. Res. 22, 3361–3382. doi: 10.1007/s11356-014-3849-9
Apel, K., and Hirt, H. (2004). Reactive oxygen species: metabolism, oxidative stress, and signal transduction. Annu. Rev. Plant Biol. 55, 373–399. doi: 10.1146/annurev.arplant.55.031903.141701
Arif, M., Chohan, M. A., Ali, S., Gul, R., and Khan, S. (2006). Response of wheat to foliar application of nutrients. J. Agric. Biol. Sci. 1, 30–34.
Armstrong, W. (1964). Oxygen diffusion from the roots of some British bog plants. Nature 204, 801–802. doi: 10.1038/204801b0
Armstrong, W. (1967). The oxidizing activity of roots in water-logged soils. Physiol. Plant. 20, 920–926. doi: 10.1111/j.1399-3054.1967.tb08379.x
Aroca, R. J., and Ruiz-Lozano, M. R. (2012). Plant Responses to Drought Stress, Edited by Aroca. Berlin; Heidelberg: Springer-Verlag.
Asada, K. (2006). Production and scavenging of reactive oxygen species in chloroplasts and their functions. Plant Physiol. 141, 391–396. doi: 10.1104/pp.106.082040
Ashraf, M. (1994). Breeding for salinity tolerance in plants. Crit. Rev. Plant Sci. 13, 17–42. doi: 10.1080/07352689409701906
Asraf, M., and Harri, P. J. C. (2004). Potential biochemical indicators of salinity tolerance in plants. Plant Sci. 166, 3–16. doi: 10.1016/j.plantsci.2003.10.024
Asrar, A. W. A., and Elhindi, K. M. (2011). Alleviation of drought stress of marigold (Tagetes erecta) plants by using arbuscular mycorrhizal fungi. Saudi J. Biol. Sci. 18, 93–98. doi: 10.1016/j.sjbs.2010.06.007
Bacha, R. E., and Hossner, L. R. (1977). Characteristics of coatings formed on rice roots as affected by iron and manganese additions. Soil Sci. Soc. Am. J. 41, 931–935. doi: 10.2136/sssaj1977.03615995004100050051x
Bashir, K., Inoue, H., Nagasaka, S., Takahashi, M., Nakanishi, H., Mori, S., et al. (2006). Cloning and characterization of deoxymugineic acid synthase genes from graminaceous plants. J. Biol. Chem. 43, 32395–32402. doi: 10.1074/jbc.M604133200
Bell, F. G., Bullock, S. E. T., Halbich, T. F. J., and Lindsay, P. (2001). Environmental impacts associated with an abandoned mine in the Witbank Coalfield, South Africa. Int. J. Coal Geol. 45, 195–216. doi: 10.1016/S0166-5162(00)00033-1
Bienfait, H. F., and Van der Mark, F. (1983). “Phytoferritin and its role in iron metabolism,” in Metals and Micronutrients. Uptake and Utilization by Plants, ed D. A. Robb (New York, NY: Academic Press), 111–123. doi: 10.1016/B978-0-12-589580-4.50014-6
Bradbury, M., and Ahmad, R. (1990). The effect of silicon on the growth of Prosopis juliflora growing in saline soil. Plant Soil 125, 71–74. doi: 10.1007/BF00010745
Brigham, E. P., McCormack, M. C., Takemoto, C. M., and Matsui, E. C. (2015). Iron status is associated with asthma and lung function in US women. PLoS ONE 10:e0117545. doi: 10.1371/journal.pone.0117545
Brown, J. C., and Ambler, J. E. (1973). “Reductants” released by roots of Fe-deficient soybeans. Agron. J. 65, 311–314. doi: 10.2134/agronj1973.00021962006500020037x
Brumbarova, T., Matros, A., Mock, H. P., and Bauer, P. (2008). A proteomic study showing differential regulation of stress, redox regulation and peroxidase proteins by iron supply and the transcription factor FER. Plant J. 54, 321–334. doi: 10.1111/j.1365-313X.2008.03421.x
Cakmak, I. (2005). The role of potassium in alleviating detrimental effects of abiotic stresses in plants. J. Plant Nutr. Soil Sci. 168, 521–530. doi: 10.1002/jpln.200420485
Carrow, R. N., Johnson, B. J., and Landry, G. W. Jr. (1988). Centipede response to foliar applications of Fe and N. Agron. J. 80, 746–750. doi: 10.2134/agronj1988.00021962008000050010x
Cervantes, C., Campos-Garcia, J., Devars, S., Guatierrez-Corrora, F., Loza-Tavera, M., Torres-Guzman, J. C., et al. (2001). Interactions of chromium with microorganisms and plants. FEMS Microbiol. Rev. 25, 335–347. doi: 10.1111/j.1574-6976.2001.tb00581.x
Chen, C. C., Dixon, J. B., and Turner, F. T. (1980). Iron plaque on rice roots, morphology and models of development. Soil Sci. Soc. Am. J. 44, 1113–1119. doi: 10.2136/sssaj1980.03615995004400050046x
Cohen, C. K., Fox, T. C., Garvin, D. F., and Kochian, L. V. (1998). The role of iron-deficiency stress responses in stimulating heavy metal transport in plants. Plant Physiol. 116, 1063–1072. doi: 10.1104/pp.116.3.1063
Conte, S. S., and Walker, E. L. (2011). Transporters contributing to iron trafficking in plants. Mol. Plant 4, 464–476. doi: 10.1093/mp/ssr015
Cramer, G. R., Urano, K., Delrot, S., Pezzotti, M., and Shinozaki, K. (2011). Effects of abiotic stress on plants: a systems biology perspective. BMC Plant Biol. 11:163. doi: 10.1186/1471-2229-11-163
Curie, C., Panaviene, Z., Loulergue, C., Dellaporta, S. L., Briat, J. F., and Walker, E. L. (2001). Maize yellow stripe1 encodes a membrane protein directly involved in Fe (III) uptake. Nature 409, 346–349. doi: 10.1038/35053080
Dahiya, S. S., and Singh, M. (1976). Effect of salinity, alkalinity and iron application on the availability of iron, manganese, phosphorus and sodium in pea (Pisum sativum L.) crop. Plant Soil 44, 697–702. doi: 10.1007/BF00011387
Davison, W., and Seed, G. (1983). The kinetics of the oxidation of ferrous iron in synthetic and natural-waters. Geochim. Cosmochim. Acta 47, 67–79. doi: 10.1016/0016-7037(83)90091-1
De Micco, V., and Aronne, G. R. (2012). Plant Responses to Drought Stress, Edited by Aroca. Berlin; Heidelberg: Springer-Verlag.
De Vos, C. H. R., and Schat, H. (1991a). “Free radicals and heavy metal tolerance,” in Ecological Responses to Environmental Stress, eds J. Rozema and J. A. C. Verkleij (Dordrecht: Kluwer Academic), 22–30. doi: 10.1007/978-94-009-0599-3_3
De Vos, C. H. R., Schat, H., Waal, M. A. M., Vooijs, R., and Ernst, W. H. O. (1991b). Increased resistance to copper induced damage of the root cell plasmalemma in copper tolerant Silene cucubalus. Physiol. Plant. 82, 523–528. doi: 10.1034/j.1399-3054.1991.820407.x
De Vos, C. H. R., Vooijs, R., Schat, H., and Ernst, W. H. O. (1989). Copper induced damage to permeability barrier in roots of Silene cucubalus. Plant Physiol. 135, 165–169. doi: 10.1016/S0176-1617(89)80171-3
Deal, E. E., and Engel, R. E. (1965). Iron, manganese, boron and zinc: effects on growth of ‘Merion’ Kentucky bluegrass. Agron. J. 57, 553–555. doi: 10.2134/agronj1965.00021962005700060011x
Deiana, S., Gessa, C., Manunza, B., Marchetti, M., and Usai, M. (1992). Mechanism and stoichiometry of the redox reaction between iron (III) and caffeic acid. Plant Soil 145, 287–294. doi: 10.1007/BF00010357
Demiral, T., and Turkan, I. (2005). Comparative lipid peroxidation, antioxidant systems and proline content in roots of two rice cultivars differing in salt tolerance. Environ. Exp. Bot. 53, 247–257. doi: 10.1016/j.envexpbot.2004.03.017
Deng, D., Wu, S. C., Wu, F. Y., Deng, H., and Wong, M. H. (2010). Effects of root anatomy and Fe plaque on arsenic uptake by rice seedlings grown in solution culture. Environ. Pollut. 158, 2589–2595. doi: 10.1016/j.envpol.2010.05.015
Dheri, G. S., Brar, M. S., and Malhi, S. S. (2007). Influence of phosphorus application on growth and cadmium uptake of spinach in two Cd contaminated soils. J. Plant Nutr. Soil Sci. 170, 495–499. doi: 10.1002/jpln.200625051
Dinneny, J. R., Long, T. A., Wang, J. Y., Jung, J. W., Mace, D., Pointer, S., et al. (2008). Cell identity mediates the response of Arabidopsis roots to abiotic stress. Science 320, 942–945. doi: 10.1126/science.1153795
Dong, J., Wu, F. B., and Zhang, G. P. (2006). Influence of cadmium on antioxidant capacity and four microelement concentrations in tomato seedlings (Lycopersicon esculentum). Chemosphere 64, 1659–1666. doi: 10.1016/j.chemosphere.2006.01.030
Donnini, S., Prinsi, B., Negri, A. S., Vigani, G., Espen, L., and Zocchi, G. (2010). Proteomic characterization of iron deficiency responses in Cucumis sativus L. roots. BMC Plant Biol. 10:268. doi: 10.1186/1471-2229-10-268
Dordas, C. (2009). “Role of nutrients in controlling plant diseases in sustainable agriculture: a review,” in Sustainable Agriculture, eds E. Lichtfouse, M. Navarrete, P. Debaeke, V. Souchere, and C. Alberola (Springer), 443–460. doi: 10.1007/978-90-481-2666-8_28
Dubeaux, G., Zelazny, E. and Vert, G. (2015). Getting to the root of plant iron uptake and cell-cell transport: polarity matters. Commun. Integr. Biol. 8:e1038441. doi: 10.1080/19420889.2015.1038441
Ebrahimian, E., and Bybordi, A. (2011). Effect of iron foliar fertilization on growth, seed and oil yield of sunflower grown under different irrigation regimes. Middle-East J. Sci. 9, 621–627.
Eckhardt, U., Marques, A. M., and Buckhout, T. J. (2001). Two iron-regulated cation transporters from tomato complement metal uptake-deficient yeast mutants. Plant Mol. Biol. 45, 437–448. doi: 10.1023/A:1010620012803
Eide, D., Broderius, M., Fett, J., and Guerinot, M. L. (1996). A novel iron-regulated metal transporter from plants identified by functional expression in yeast. Proc. Natl. Acad. Sci. U.S.A. 93, 5624–5628. doi: 10.1073/pnas.93.11.5624
Elanz, E., Mohsen, R., and Ahmed, B. (2011). Influence of salt stress on cations concentration quality and quantity of sunflower cultivars. J. Food Agric. Environ. 2, 469–476.
Emamverdian, A., Ding, Y., Mokhberdoran, F., and Xie, Y. (2015). Heavy metal stress and some mechanisms of plant defense response. ScientificWorldJournal 2015:756120. doi: 10.1155/2015/756120
Emerson, D., Weiss, J. V., and Megonigal, J. P. (1999). Iron-oxidizing bacteria are associated with ferric hydroxide precipitates (Fe-plaque) on the roots of wetland plants. Appl. Environ. Microb. 65, 2758–2761.
FAO (2008). Land Resources, Management, Planning and Use. Available online at: http://www.fao.org/ag/agl/agll/spush/ (Accessed April 25).
Farooq, M., Basra, S. M. A., Wahid, A., Ahmad, N., and Saleem, B. A. (2009). Improving the drought tolerance in rice (Oryza sativa L.) by exogenous application of salicylic acid. J. Agron. Crop Sci. 195, 237–246. doi: 10.1111/j.1439-037X.2009.00365.x
Farooq, M., Hussain, M., Wahid, A. K., and Siddique, H. M. (2012). Plant Responses to Drought Stress, Edited by Aroca. Berlin; Heidelberg: Springer-Verlag.
Fenton, H. J. H. (1894). LXXIII.—Oxidation of tartaric acid in presence of iron. J. Chem. Soc. Trans. 65, 899–910. doi: 10.1039/CT8946500899
Flowers, T. J., Troke, P. F., and Yeo, A. R. (1977). The mechanism of salt tolerance in halophytes. Annu. Rev. Plant Physiol. 28, 89–121. doi: 10.1146/annurev.pp.28.060177.000513
Foy, C. D., Chaney, R., and White, M. C. (1978). The physiology of metal toxicity in plants. Annu. Rev. Plant Physiol. 29, 511–566. doi: 10.1146/annurev.pp.29.060178.002455
Garnier, J. M., Travassac, F., Lenoble, V., Rose, J., Zheng, Y., Hossain, M. S., et al. (2010). Temporal variations in arsenic uptake by rice plants in Bangladesh: the role of iron plaque in paddy fields irrigated with ground water. Sci. Total Environ. 408, 4185–4193. doi: 10.1016/j.scitotenv.2010.05.019
Ghasemia, S., Khoshgoftarmanesha, A. H., Afyunia, M., and Hadadzadehb, H. (2014). Iron (II)–amino acid chelates alleviate salt-stress induced oxidative damages on tomato grown in nutrient solution culture. Sci. Hortic. 165, 91–98. doi: 10.1016/j.scienta.2013.10.037
Gill, S. S., and Tuteja, N. (2011). Cadmium stress tolerance in crop plants probing the role of sulfur. Plant Signal. Behav. 6, 215–222. doi: 10.4161/psb.6.2.14880
Glinski, D. S., Carrow, R. N., and Karnok, K. J. (1992). Iron fertilization effects on shoot/root growth, water use, and drought stress of creeping bentgrass. Agron. J. 84, 496–503. doi: 10.2134/agronj1992.00021962008400030027x
Gong, Q., Li, P., Ma, S., Indu Rupassara, S., and Bohnert, H. J. (2005). Salinity stress adaptation competence in the extremophile Thellungiella halophila in comparison with its relative Arabidopsis thaliana. Plant J. 44, 826–839. doi: 10.1111/j.1365-313X.2005.02587.x
Gratao, P. L., Polle, A., Lea, P. J., and Azevedo, R. A. (2005). Making the life of heavy metal stressed plants a little easier. Funct. Plant Biol. 32, 32481–32494. doi: 10.1071/FP05016
Greipsson, S., and Crowder, A. A. (1992). Amelioration of copper and nickel toxicity by iron plaque on roots of rice (Oryza sativa L.). Can. J. Bot. 70, 824–830. doi: 10.1139/b92-105
Grotz, N., and Guerinot, M. L. (2006). Molecular aspects of Cu, Fe and Zn homeostasis in plants. Biochim. Biophys. Acta 1763, 595–608. doi: 10.1016/j.bbamcr.2006.05.014
Guerinot, M. L. (2000). The ZIP family of metal transporters. Biochim. Biophys. Acta 1465, 190–198. doi: 10.1016/S0005-2736(00)00138-3
Gunes, A., Inal, A., Alpaslan, M., Eraslan, F., Bagci, E. G., and Cicek, N. (2007). Salicylic acid induced changes on some physiological parameters symptomatic for oxidative stress and mineral nutrition in maize (Zea mays L.) grown under salinity. J. Plant Physiol. 164, 728–736. doi: 10.1016/j.jplph.2005.12.009
Haber, F., and Weiss, J. (1934). The catalytic decomposition of hydrogen peroxide by iron salts. Proc. R. Soc. A 147, 332–351. doi: 10.1098/rspa.1934.0221
Hansch, R., and Mendel, R. R. (2009). Physiological functions of mineral micronutrients (Cu, Zn, Mn, Fe, Ni, Mo, B, Cl). Curr. Opin. Plant Biol. 12, 259–266. doi: 10.1016/j.pbi.2009.05.006
Hansel, C. M., Fendorf, S., Sutton, S., and Newville, M. (2001). Characterization of Fe plaque and associated metals on the roots of mine-waste impacted aquatic plants. Environ. Sci. Technol. 35, 3863–3868. doi: 10.1021/es0105459
Hassan, M. J., Zhang, G., Wu, F., Wei, K., and Chen, Z. (2005). Zinc alleviates growth inhibition and oxidative stress caused by cadmium in rice. J. Plant Nutr. Soil Sci. 168, 255–261. doi: 10.1002/jpln.200420403
Hassan, N. A. K., Drew, J. V., Knudsen, D., and Olson, R. A. (1970). Influence of soil salinity on production of dry matter and uptake and distribution of nutrients in barley and corn. 2. Corn (Zea mays L.). Agron. J. 62, 46–51. doi: 10.2134/agronj1970.00021962006200010015x
Heidari, M., Galavi, M., and Hassani, M. (2013). Effect of sulfur and iron fertilizers on yield, yield components and nutrient uptake in sesame (Sesamum indicum L.) under water stress. Afr. J. Biotechnol. 10, 8816–8822. doi: 10.5897/AJB11.854
Heidari, M., and Sarani, S. (2012). Growth, biochemical components and ion content of Chamomile (Matricaria chamomilla L.) under salinity stress and iron deficiency. J. Saudi Soc. Agric. Sci. 11, 37–42. doi: 10.1016/j.jssas.2011.05.002
Hellin, E., Hernandez-Cortez, J. A., Piqueras, A., Olmos, E., and Sevilla, F. (1995). The influence of the iron content on the superoxide dismutase activity and chloroplast ultra structure of Citrus limon in iron nutrition in soil and plants. J. Plant Nutr. 5, 211–229.
Henriques, R., Jásik, J., Klein, M., Martinoia, E., Feller, U., Schell, J., et al. (2002). Knock-out of Arabidopsis metal transporter gene IRT1 results in iron deficiency accompanied by cell differentiation defects. Plant Mol. Biol. 50, 587–597. doi: 10.1023/A:1019942200164
Hoagland, D. R., and Arnon, D. I. (1950). The Water-Culture Method for Growing Plants Without Soil, 2nd Edn. Circular. California Agricultural Experiment Station, 347.
HongBo, S., ZongSuo, L., and MingAn, S. (2005). Changes of anti-oxidative enzymes and MDA content under soil water deficits among 10 wheat (Triticum aestivum L.) genotypes at maturation stage. Colloid Surf. B 45, 7–13. doi: 10.1016/j.colsurfb.2005.06.016
Ionenko, I. F., Dautova, N. R., and Anisimov, A. V. (2012). Early changes of water diffusional transfer inmaize roots under the influence of water stress. Environ. Exp. Bot. 76, 16–23. doi: 10.1016/j.envexpbot.2011.09.012
Ishimaru, Y., Kakei, Y., Shimo, H., Bashir, K., Sato, Y., Sato, Y., et al. (2011). A rice phenolic efflux transporter is essential for solubilizing precipitated apoplasmic iron in the plant stele. J. Biol. Chem. 286, 24649–24655. doi: 10.1074/jbc.M111.221168
Iturbe-Ormaetxe, I., Escuredo, P. R., Arrese-Igor, C., and Becana, M. (1998). Oxidative damage in pea plants exposed to water deficit or paraquat. Plant Physiol. 116, 173–181. doi: 10.1104/pp.116.1.173
Jithesh, M. N., Prashanth, S. R., Sivaprakash, K. R., and Parid, A. K. (2006). Antioxidative response mechanisms in halophytes: their role in stress defense. J. Genet. 85, 237–254. doi: 10.1007/BF02935340
Kehrer, J. P. (2000). The Haber–Weiss reaction and mechanisms of toxicity. Toxicology 149, 43–50. doi: 10.1016/S0300-483X(00)00231-6
Khan, H. R., McDonald, G. K., and Rengel, Z. (2003).Zn fertilization improves water use efficiency, grain yield and seed Zn content in chickpea. Plant Soil 249, 389–400. doi: 10.1023/A:1022808323744
Khan, M. A., Ansari, R., Ali, H., Gul, B., and Nielsen, B. L. (2009). Panicum turgidum: a sustainable feed alternative for cattle in saline areas. Agric. Ecosyst. Environ. 129, 542–546. doi: 10.1016/j.agee.2008.10.014
Khan, M. A., and Gulzar, S. (2003). Germination responses of Sporobolus ioclados: a saline desert grass. J. Arid Environ. 53, 387–394. doi: 10.1006/jare.2002.1045
Kim, S. A., and Guerinot, M. L. (2007). Mining iron: iron uptake and transport in plants. FEBS Lett. 581, 2273–2280. doi: 10.1016/j.febslet.2007.04.043
Kobayashi, T., and Nishizawa, N. K. (2012). Iron uptake, translocation, and regulation in higher plants. Annu. Rev. Plant Biol. 63, 131–152. doi: 10.1146/annurev-arplant-042811-105522
Kobayashi, T., Nakayama, Y., Itai, R. N., Nakanishi, H., Yoshihara, T., Mori, S., et al. (2003). Identification of novel cis-acting elements, IDE1 and IDE2, of the barley IDS2 gene promoter conferring iron-deficiency-inducible, root-specific expression in heterogeneous tobacco plants. Plant J. 36, 780–793. doi: 10.1046/j.1365-313X.2003.01920.x
Kobraee, S., Shamsi, K., and Rasekhi, B. (2011). Effect of micronutrients application on yield and yield components of soybean. Annu. Biol. Res. 2, 476–482.
Krämer, U., Talke, I. N., and Hanikenne, M. (2007). Transition metal transport. FEBS Lett. 581, 2263–2272. doi: 10.1016/j.febslet.2007.04.010
Kumar, P., Tewari, R. K., and Sharma, P. N. (2010). Sodium nitroprusside-mediated alleviation of iron deficiency and modulation of antioxidant responses in maize plants. AoB Plants 2, 1–11. doi: 10.1093/aobpla/plq002
Kumar, S., Asif, M. H., Chakrabarty, D., Tripathi, R. D., Dubey, R. S., and Trivedi, P. K. (2013). Expression of a rice Lambda class of glutathione S-transferase, OsGSTL2, in Arabidopsis provides tolerance to heavy metal and other abiotic stresses. J. Hazard. Mater. 248, 228–237. doi: 10.1016/j.jhazmat.2013.01.004
Lambers, H., Chapin, F. S., and Pons, T. L. (2008). Plant Physiological Ecology, 2nd Edn. New York, NY: Springer.
Lan, P., Li, W., Wen, T. N., Shiau, J. Y., Wu, Y. C., Lin, W., et al. (2011). iTRAQ protein profile analysis of Arabidopsis roots reveals new aspects critical for iron homeostasis. Plant Physiol. 155, 821–834. doi: 10.1104/pp.110.169508
Levan, M. A., and Riha, S. J. (1986). The precipitation of black oxide coatings on flooded conifer roots of low internal porosity. Plant Soil 95, 33–42. doi: 10.1007/BF02378850
Li, Y. P., Ye, W., Wang, M., and Yan, X. D. (2009). Climate change and drought: a risk assessment of crop yield impacts. Clim. Res. 39, 31–46. doi: 10.3354/cr00797
Liu, H., Zhang, J., Christie, P., and Zhang, F. (2008). Influence of iron plaque on uptake and accumulation of Cd by rice (Oryza sativa L.) seedlings grown in soil. Sci. Total Environ. 394, 361–368. doi: 10.1016/j.scitotenv.2008.02.004
Liu, H. J., Zhang, J. L., and Zhang, F. S. (2007). Role of iron plaque in Cd uptake by and translocation within rice (Oryza sativa L.) seedlings grown in solution culture. Environ. Exp. Bot. 59, 314–320. doi: 10.1016/j.envexpbot.2006.04.001
Liu, J., Zhang, X. H., Tran, H., Wang, D. Q., and Zhu, Y. N. (2011). Heavy metal contamination and risk assessment in water, paddy soil, and rice around an electroplating plant. Environ. Sci. Pollut. Res. 18, 1623–1632. doi: 10.1007/s11356-011-0523-3
Loggini, B., Scartazza, A., Brugnoli, E., and Navari-Izzo, F. (1999). Antioxidative defence system, pigment composition, photosynthetic efficiency in two wheat cultivars subjected to drought. Plant Physiol. 119, 1091–1099. doi: 10.1104/pp.119.3.1091
López Jiménez, A., Alcalde Blanco, S., Barrera Guerra, J. L., and Cortez Flores, J. I. (1985). Evaluación Fisiológico Nutrimental de Tres Razas de Aguacate en Suelos con Diferentes Niveles de CaCO3 (No. TESIS.). Colegio de Postgraduados, Campus Montecillo, Centro de Fruticultura.
Macedo, A. F. (2012). “Abiotic stress responses in plants: metabolism to productivity,” in Abiotic Stress Responses in Plants, eds P. Ahmad and M. Prasad (New York, NY: Springer). doi: 10.1007/978-1-4614-0634-1_3
Mahmood, T., Qureshi, R. H., Aslam, M., Qadir, M., and Ashraf, M. (1990). “Effect of salinity on germination, growth and chemical composition of some members of gramineae,” in 3rd National Congress of Soil Sci, March 20-22 (Lahore).
Mai, H. J., and Bauer, P. (2016). From the proteomic point of view: integration of adaptive changes to iron deficiency in plants. Curr. Plant Biol. 5, 45–56. doi: 10.1016/j.cpb.2016.02.001
Mai, H. J., Lindermayr, C., Toerne, C., Fink-Straube, C., Durner, J., and Bauer, P. (2015). Iron and fer-like iron deficiency-induced transcription factor-dependent regulation of proteins and genes in Arabidopsis thaliana roots. Proteomics 15, 3030–3047. doi: 10.1002/pmic.201400351
Manthey, J. A., Tisserat, B., and Crowley, D. E. (1996). Root responses of sterile grown onion plants to iron deficiency. J. Plant Nutr. 19, 145–161. doi: 10.1080/01904169609365113
Mantri, N., Patade, V., Penna, S., Ford, R., and Pang, E. (2012). “Abiotic stress responses in plants: present and future,” in Abiotic Stress Responses in Plants, eds P. Ahmad and M. Prasad (New York, NY: Springer). doi: 10.1007/978-1-4614-0634-1_1
Marschner, H., and Römheld, V. (1994). Strategies of plants for acquisition of iron. Plant Soil 165, 375–388. doi: 10.1007/BF00008069
McKersie, B. D., Bowley, S. R., and Jones, K. S. (1999). Winter survival of transgenic alfalfa overexpressing superoxide dismustase. Plant Physiol. 119, 839–847. doi: 10.1104/pp.119.3.839
McKersie, B. D., Murnaghan, J., Jones, K. S., and Bowley, S. R. (2000). Iron-superoxide dismutase expression in transgenic alfalfa increases winter survival without a detectable increase in photosynthetic oxidative stress tolerance. Plant Physiol. 122, 1427–1438. doi: 10.1104/pp.122.4.1427
Meda, A. R., Scheuermann, E. B., Prechsl, U. E., Erenoglu, B., Schaaf, G., et al. (2007). Iron acquisition by phytosiderophores contributes to cadmium tolerance. Plant Physiol. 143, 1761–1773. doi: 10.1104/pp.106.094474
Mendelssohn, J. A., and Postek, M. T. (1982). Elemental analysis of deposits on the roots of Spartina alterniflora Loisel. Am. J. Bot. 69, 904–912. doi: 10.2307/2442887
Minner, D. D., and Butler, J. D. (1984). Correcting iron deficiency of Kentucky bluegrass. HortScience 19, 109–110.
Mithofer, A., Schulze, B., and Boland, W. (2004). Biotic and heavy metal stress response in plants: evidence for common signals. FEBS Lett. 566, 1–5. doi: 10.1016/j.febslet.2004.04.011
Moghadam, H. R. T., Zahedi, H., and Ghooshchi, F. (2011). Oil quality of canola cultivars in response to water stress and super absorbent polymer application. Pesq. Agropec. Trop. 41, 579–586. doi: 10.5216/pat.v41i4.13366
Moharana, P. C., Sharma, B. M., Biswas, D. R., Dwivedi, B. S., and Singh, R. V. (2012). Long-term effect of nutrient management on soil fertility and soil organic carbon pools under a 6-year-old pearl millet-wheat cropping system in an Inceptisol of subtropical India. Field Crop Res. 136, 32–41. doi: 10.1016/j.fcr.2012.07.002
Monjezi, F., Vazin, F., and Hassanzadehdelouei, M. (2013). Effects of iron and zinc spray on yield and yield components of wheat (Triticum aestivum L.) in drought stress. Cercet. Agron. Mold. 46, 23–32. doi: 10.2478/v10298-012-0072-z
Montanini, B., Blaudez, D., Jeandroz, S., Sanders, D., and Chalot, M. (2007). Phylogenetic and functional analysis of the Cation Diffusion Facilitator (CDF) family: improved signature and prediction of substrate specificity. BMC Genomics 8:107. doi: 10.1186/1471-2164-8-107
Morrissey, J., and Guerinot, M. L. (2009). Iron uptake and transport in plants: the good, the bad, and the ionome. Chem. Rev. 109, 4553–4567. doi: 10.1021/cr900112r
Morrissey, J., Baxter, I. R., Lee, J., Li, L., Lahner, B., Grotz, N., et al. (2009). The ferroportin metal efflux proteins function in iron and cobalt homeostasis in Arabidopsis. Plant Cell 21, 3326–3338. doi: 10.1105/tpc.109.069401
Mostafa, M. G., Chen, Y. H., Jean, J. S., Liu, C. C., and Lee, Y. C. (2011). Kinetics and mechanism of arsenate removal by nanosized iron oxide-coated perlite. J. Hazard. Mater. 187, 89–95. doi: 10.1016/j.jhazmat.2010.12.117
Munns, R. (2002). Comparative physiology of salt and water stress. Plant Cell Environ. 25, 239–250 doi: 10.1046/j.0016-8025.2001.00808.x
Munns, R., and Tester, M. (2008). Mechanisms of salinity tolerance. Annu. Rev. Plant Biol. 59, 651–681. doi: 10.1146/annurev.arplant.59.032607.092911
Nagajyoti, P. C., Lee, K. D., and Sreekanth, T. V. M. (2010). Heavy metals, occurrence and toxicity for plants: a review. Environ. Chem. Lett. 8, 199–216. doi: 10.1007/s10311-010-0297-8
Nazar, R., Iqbal, N., Masood, A., Khan, M. I. R., Syeed, S., and Khan, N. A. (2012). Cadmium toxicity in plants and role of mineral nutrients in its alleviation. Am. J. Plant Sci. 3, 1476–1489. doi: 10.4236/ajps.2012.310178
Nazar, R., Iqbal, N., Masood, A., Syeed, S., and Khan, N. A. (2011). Understanding the significance of sulfur in improving salinity tolerance in plants. Environ. Exp. Bot. 70, 80–87. doi: 10.1016/j.envexpbot.2010.09.011
Nevo, Y., and Nelson, N. (2006). The NRAMP family of metal-ion transporters. Biochim. Biophys. Acta 1763, 609–620. doi: 10.1016/j.bbamcr.2006.05.007
Nies, D. H. (1992). Resistance to cadmium, cobalt, zinc, and nickel in microbes. Plasmid 27, 17–28. doi: 10.1016/0147-619X(92)90003-S
Okcu, G., Kaya, M. D., and Atak, M. (2005). Effects of salt and drought stresses on germination and seedling growth of pea (Pisum sativum L.). Turk. J. Agric. For. 29, 237–242.
Oliveira, H. (2012). Chromium as an environmental pollutant: insights on induced plant toxicity. J. Bot. 2012:375843. doi: 10.1155/2012/375843
Olsen, R. A., Bennett, J. H., Blume, D., and Brown, J. C. (1981). Chemical aspects of the Fe stress response mechanism in tomatoes. J. Plant Nutr. 3, 905–921. doi: 10.1080/01904168109362887
Olsen, R. A., Brown, J. C., Bennett, J. H., and Blume, D. (1982). Reduction of Fe3+ as it relates to Fe chlorosis. J. Plant Nutr. 5, 433–445. doi: 10.1080/01904168209362971
Pandey, M., Dixit, V. K., Katiyar, G. P., Nath, G., Sundram, S. M., Chandra, N., et al. (2005). Ganga water pollution and occurrence of enteric diseases in Varanasi city. Ind. J. Commun. Med. 30, 115–120.
Pandolfini, T., Gabbrielli, R. R., and Comparini, C. (1992). Nickel toxicity and peroxidase activity in seedlings of Triticum aestivum L. Plant Cell Environ. 15, 719–725. doi: 10.1111/j.1365-3040.1992.tb01014.x
Pankovic, D., Plesnicar, M., Maksimoviic, I. A., Petrovic, N., Sakac, Z., and Kastori, R. (2000). Effect of nitrogen nutrition on photosynthesis in Cd treated sunflower plants. Ann. Bot. 86, 841–847. doi: 10.1006/anbo.2000.1250
Parhamfar, T. (2006). The Effect Fertilizers Macro, Micro and Setaria Italica Millet Harvest Time on Yield And Forage Quality. Master's thesis of Agriculture, Zabol University, 105.
Parihar, P., Singh, S., Singh, R., Singh, V. P., and Prasad, S. M. (2015). Effect of salinity stress on plants and its tolerance strategies: a review. Environ. Sci. Pollut. Res. 22, 4056–4075. doi: 10.1007/s11356-014-3739-1
Passariello, B., Giuliano, V., Quaresima, S., Barbaro, M., Caroli, S., Forte, G., et al. (2002). Evaluation of the environmental contamination at an abandoned mining site. Microchem. J. 73, 245–250. doi: 10.1016/S0026-265X(02)00069-3
Payne, W. A., Drew, M. C., Hossner, L. R., Lascano, R. J., Onken, A. B., and Wendt, C. W. (1992). Soil phosphorus availability and pearl millet water-use efficiency. Crop Sci. 32, 1010–1015. doi: 10.2135/cropsci1992.0011183X003200040035x
Pirzad, A., and Shokrani, F. (2012). Effects of iron application on growth characters and flower yield of Calendula officinalis L. under water stress. World Appl. Sci. J. 18, 1203–1208. doi: 10.5829/idosi.wasj.2012.18.09.538
Pourgholam, M., Nemati, N., and Oveysi, M. (2013). Effect of zinc and iron under the influence of drought on yield and yield components of rapeseed (Brassica napus). Ann. Biol. Res. 4, 186–189.
Qadir, S., Qureshi, M. I., Javed, S., and Abdin, M. Z. (2004). Genotypic variation in phytoremediation potential of Brassica juncea cultivars exposed to Cd stress. Plant Sci. 167, 1171–1181. doi: 10.1016/j.plantsci.2004.06.018
Rabhi, M., Barhoumi, Z., Ksouri, R., Abdelly, C., and Gharsalli, M. (2007). Interactive effects of salinity and iron deficiency in Medicago ciliaris. R. Biol. 330, 779–788. doi: 10.1016/j.crvi.2007.08.007
Rahimizadeh, M., Habibi, D., Madani, H., Mohammadi, G. N., Mehraban, A., and Sabet, A. M. (2007). The effect of micronutrients on antioxidant enzymes metabolism in sunflower (Helianthus annuus L.) under drought stress. Helia 30, 167–174. doi: 10.2298/HEL0747167R
Rahmana, M. A., Hasegawaa, H., Uedaa, K., Makia, T., and Rahman, M. M. (2008). Arsenic uptake by aquatic macrophyte Spirodela polyrhiza L., Interactions with phosphate and iron. J. Hazard. Mater. 160, 356–361. doi: 10.1016/j.jhazmat.2008.03.022
Reddy, G. N., and Prasad, M. N. V. (1992). Characterization of cadmium binding protein from Scenedesmus quadricauda and Cd toxicity reversal by phytochelatin constituting amino acids and citrate. J. Plant Physiol. 140, 156–162. doi: 10.1016/S0176-1617(11)80927-2
Richardson, D. R., Lane, D. J., Becker, E. M., Huang, M. L. H., Whitnall, M., Rahmanto, Y. S., et al. (2010). Mitochondrial iron trafficking and the integration of iron metabolism between the mitochondrion and cytosol. Proc. Natl. Acad. Sci. U.S.A. 107, 10775–10782. doi: 10.1073/pnas.0912925107
Rizhsky, L., Liang, H. J., Shuman, J., Shulaev, V., Davletova, S., and Mittler, R. (2004). The response of Arabidopsis to a combination of drought and heat stress. Plant Physiol. 134, 1683–1696. doi: 10.1104/pp.103.033431
Römheld, V., and Marschner, H. (1986). Evidence for a specific uptake system for iron phytosiderophores in roots of grasses. Plant Physiol. 80, 175–180. doi: 10.1104/pp.80.1.175
Robinson, N. J., Procter, C. M., Connolly, E. L., and Guerinot, M. L. (1999). A ferric-chelate reductase for iron uptake from soils. Nature 397, 694–697. doi: 10.1038/17800
Rodríguez-Celma, J., Lattanzio, G., Grusak, M. A., Abadía, A., Abadía, J., and López-Millán, A. F. (2011). Root responses of Medicago truncatula plants grown in two different iron deficiency conditions: changes in root protein profile and riboflavin biosynthesis. J. Proteome Res. 10, 2590–2601. doi: 10.1021/pr2000623
Rodríguez-Celma, J., Lattanzio, G., Jiménez, S., Briat, J. F., Abadía, J., Abadía, A., et al. (2013). Changes induced by Fe deficiency and Fe resupply in the root protein profile of a peach-almond hybrid rootstock. J. Proteome Res. 12, 1162–1172. doi: 10.1021/pr300763c
Rogers, E. E., Eide, D. J., and Guerinot, M. L. (2000). Altered selectivity in an Arabidopsis metal transporter. Proc. Natl. Acad. Sci. U.S.A. 97, 12356–12360. doi: 10.1073/pnas.210214197
Römheld, V., and Marschner, H. (1983). Mechanism of iron uptake by peanut plants I. FeIII reduction, chelate splitting, and release of phenolics. Plant Physiol. 71, 949–954. doi: 10.1104/pp.71.4.949
Rotaru, V. (2011). The effect of phosphorus and iron on plant growth and Nutrient status of two soybean (Glycine max. L.) cultivars Under suboptimal water regime of soil. Lucrări Stiintifice Suplimentseria Agronomie 54, 11–16.
Rotaru, V., and Sinclair, T. (2009). Influence of plant phosphorus and iron concentrations on growth of soybean. J. Plant Nutr. 32, 1513–1526. doi: 10.1080/01904160903093828
Rout, G. R., and Sahoo, S. (2015). Role of iron in plant growth and metabolism. Rev. Agric. Sci. 3, 1–24. doi: 10.7831/ras.3.1
Sairam, R. K., and Tyagi, A. (2004). Physiology and molecular biology of salinity stress tolerance in plants. Curr. Sci. 86, 407–421.
Samarah, N., Mullen, R., and Cianzio, S. (2004). Size distribution and mineral nutrients of soybean seeds in response to drought stress. J. Plant Nutr. 27, 815–835. doi: 10.1081/PLN-120030673
Samis, K., Bowley, S., and McKersie, B. (2002). Pyramiding Mn-superoxide dismutase transgenes to improve persistence and biomass production in alfalfa. J. Exp. Bot. 53, 1343–1350.
Sarwar, N., Malhi, S. S., Zia, M. H., Naeem, A., Bibi, S., and Farid, G. (2010). Role of mineral nutrition in minimizing cadmium accumulation by plants. J. Sci. Food Agric. 90, 925–937. doi: 10.1002/jsfa.3916
Sattelmacher, B., and Horst, W. J. (2007). The Apoplast of Higher Plants: Compartment of Storage, Transport and Reactions—The Significance of the Apoplast for the Mineral Nutrition of Higher Plants. Dordrecht: Springer.
Saxena, I., and Shekhawat, G. S. (2013). Nitric oxide (NO) in alleviation of heavy metal induced phytotoxicity and its role in protein nitration. Nitric Oxide 32, 13–20. doi: 10.1016/j.niox.2013.03.004
Scandalios, J. G. (1990). Response of plant antioxidant defense genes to environmental stress. Adv. Gen. 28, 1–41. doi: 10.1016/S0065-2660(08)60522-2
Schmidt, R. E., and Snyder, V. (1984). Effect of N, temperature,and moisture stress on the growth and physiology of bent grass and response to chelated iron. Agron. J. 76, 590–594. doi: 10.2134/agronj1984.00021962007600040020x
Schwartz, C., Gerard, E., Perronnet, K., and Morel, J. L. (2001). Measurement of in situ phytoextraction of zinc by spontaneous metallophytes growing on a former smelter site. Sci. Total Environ. 279, 215–221. doi: 10.1016/S0048-9697(01)00784-7
Shao, G., Chen, M., Wang, D., Xu, C., Mou, R., Cao, Z., et al. (2008). Using iron fertilizer to control Cd accumulation in rice plants: a new promising technology. Sci. China C Life Sci. 51, 245–253. doi: 10.1007/s11427-008-0031-y
Shao, G., Chen, M., Wang, W., Mou, R., and Zhang, G. (2007). Iron nutrition affects cadmium accumulation and toxicity in rice plants. Plant Growth Regul. 53, 33–42. doi: 10.1007/s10725-007-9201-3
Sharma, P., Jha, A. B., Dubey, R. S., and Pessarakli, M. (2012). Reactive oxygen species, oxidative damage, and antioxidative defense mechanism in plants under stressful conditions. J. Bot. 2012, 1–26. doi: 10.1155/2012/217037
Sharma, S. S., Kaul, S., Metwally, A., Goyal, K. C., Finkemeier, I., and Dietz, K. J. (2004). Cadmium toxicity to barley (Hordeumvulgare) as affected by varying Fe nutritional status. Plant Sci. 166, 1287–1295. doi: 10.1016/j.plantsci.2004.01.006
Sharp, R. E., and Davies, W. J. (1989). “Regulation of growth anddevelopment of plants growing with a restricted supply of water,” in Plants Under Stress, eds H. G. Jones, T. L. Flowers, and M. B. Jones (London: Cambridge University Press), 71–93.
Sharp, R. E., Poroyko, V., Hejlek, L. G., Spollen, W. G., Springer, G. K., Bohnert, H. J., et al. (2004). Root growth maintenance during water deficits: physiology to functional genomics. J. Exp. Bot. 55, 2343–2351. doi: 10.1093/jxb/erh276
Singh, R., Gautam, N., Mishra, A., and Gupta, R. (2011). Heavy metal and living systems: an overview. Ind. J. Pharmacol. 43, 246–253. doi: 10.4103/0253-7613.81505
Singh, S., Parihar, P., Singh, R., Singh, V. P., and Prasad, S. M. (2016). Heavy metal tolerance in plants: role of transcriptomics, proteomics, metabolomics, and ionomics. Front. Plant Sci. 6:1143. doi: 10.3389/fpls.2015.01143
Singh, S., Srivastava, P. K., Kumar, D., Tripathi, D. K., Chauhan, D. K., and Prasad, S. M. (2015). Morpho-anatomical and biochemical adapting strategies of maize (Zea mays L.) seedlings against lead and chromium stresses. Biocatal. Agric. Biotechnol. 4, 286–295. doi: 10.1016/j.bcab.2015.03.004
Singh, S., Tripathi, D. K., Singh, S., Sharma, S., Dubey, N. K., Chauhan, D. K., et al. (2017). Toxicity of aluminium on various levels of plant cells and organism: a review. Environ. Experi. Bot. 137, 177–193. doi: 10.1016/j.envexpbot.2017.01.005
Sinha, S., Guptha, M., and Chandra, P. (1997). Oxidative stress induced by iron in Hydrilla verticillata (i.f) royle: response of antioxidants. Ecotoxicol. Environ. Safe. 38, 286–291. doi: 10.1006/eesa.1997.1598
Slatni, T., Kroma, A., Aydi, S., Gouia, C., and Abdelly, C. H. (2008). Growth nitrogen fixation and ammonium assimilation in common been subjected to iron deficiency. Plant Soil 312, 49–57. doi: 10.1007/s11104-007-9481-4
Smirnoff, N. (1993). The role of active oxygen in the response of plants to water deficit and desiccation. New Phytol. 125, 27–58. doi: 10.1111/j.1469-8137.1993.tb03863.x
Snyder, V., and Schmidt, R. E. (1974). “Nitrogen and iron fertilization of bentgrass,” in Proceedings of the Second International Turfgrass Research Conference (No. proceedingsofth2) (American Society of Agronomy, Crop Science Society of America), 176–185.
Somashekariah, B. V., Padmaja, K., and Prasad, A. R. K. (1992). Phytotoxicity of cadmium ions on germinating seedlings of mung bean (Phaseolus vulgaris): involvement of lipid peroxides in chlorophyll degradation. Physiol. Plant. 85, 85–89. doi: 10.1034/j.1399-3054.1992.850113.x
Soyder, V., and Schmidt, R. E. (1974). “Nitrogen and iron fertilization on bent grass,” in Proceedings 2nd International Turfgrass Research Conference VPI, Blacksburg, VA 15–20 June 1973, ed E. C. Roberts (Madison, WI, ASA), 176–185.
Spollen, W. G., Sharp, R. E., Saab, I. N., and Wu, Y. (1993). “Regulation of cell expansion in roots and shoots at low water potentials,” in Water Deficits: Plant Responses from Cell to Community, eds J. A. C. Smith, and H. Griffiths (Oxford: BIOS Scientific Publishers), 37–52.
Srivastava, S., Tripathi, R. D., and Dwivedi, U. N. (2004). Synthesis of phytochelatins and modulation of antioxidants in response to cadmium stress in Cuscuta reflexa—an angiospermic parasite. J. Plant Physiol. 161, 665–674. doi: 10.1078/0176-1617-01274
Steduto, P., Albrizio, R., Giorio, P., and Sorrentino, G. (2000). Gas exchange response and stomatal and non-stomatal limitations to carbon assimilation of sunflower under salinity. Environ. Exp. Bot. 44, 243–255. doi: 10.1016/S0098-8472(00)00071-X
Sultana, N., Ikeda, T., and Kashem, M. A. (2001). Effect of foliar spray of nutrient solutions on photosynthesis, dry matter accumulation and yield in seawater-stressed rice. Environ. Exp. Bot. 46, 129–140. doi: 10.1016/S0098-8472(01)00090-9
Taiz, L., and Zeiger, E. (1991). Plant Physiology. Redwood City, CA: The Benjamin/Cummings Publ. Co. Inc.
Taiz, L., and Zeiger, E. (2010). Plant Physiology, 5th Edn. Sunderland, MA: Sinauer Associates Inc. Publishers.
Talei, D., Kadir, M. A., Yusop, M. K., Valdiani, A., and Abdullah, M. P. (2012). Salinity effects on macro and micronutrients uptake in medicinal plant king of bitters (Andrographis paniculata Nees.). Plant Omics J. 5, 271–278.
Talei, D., Mihdzar, A. K., Khanif, M. Y., Saad, M. S., and Valdiani, A. (2011). Effects of different surface sterilizers on seed germination and contamination of king of bitters (Andrographis paniculata Nees.). Am. Eurasian J. Agric. Environ. Sci. 10, 639–643.
Tanaka, A., Loe, R., and Navasero, S. A. (1966). Some mechanisms involved in the development of iron toxicity symptoms in the rice plant. Soil Sci. Plant Nutr. 12, 32–38. doi: 10.1080/00380768.1966.10431951
Taylor, G. J., and Crowder, A. A. (1983). Use of the DCB technique for extraction of hydrous iron oxides from roots of wetland plants. Am. J. Bot. 70, 1254–1257. doi: 10.2307/2443295
Taylor, G. J., Crowder, A. A., and Rodden, R. (1984). Formation and morphology of an iron plaque on the roots of Typha Latifolia L. grown in solution culture. Am. J. Bot. 71, 666–675. doi: 10.2307/2443363
Tchounwou, P. B., Yedjou, C. G., Patlolla, A. K., and Sutton, D. J. (2013). Heavy metal toxicity and the environment. EXS 101, 133–164. doi: 10.1007/978-3-7643-8340-4_6
Tester, M., and Davenport, R. (2003). Na+ tolerance and Na+ transport in higher plants. Ann. Bot. 91, 503–527. doi: 10.1093/aob/mcg058
Thimm, O., Essigmann, B., Kloska, S., Altmann, T., and Buckhout, T. J. (2001). Response of Arabidopsis to iron deficiency stress as revealed by microarray analysis. Plant Physiol. 127, 1030–1043. doi: 10.1104/pp.010191
Tlustos, P., Szakova, J., Korinek, K., Pavlikova, D., Hanc, A., and Balik, J. (2006). The effect of liming on cadmium, lead and zinc uptake reduction by spring wheat grown in contaminated soil. Plant Soil Environ. 52, 16–24.
Tognetti, V. B., Zurbriggen, M. D., Morandi, E. N., Fillat, M. F., Valle, E. M., Hajirezaei, M. R., et al. (2007). Enhanced plant tolerance to iron starvation by functional substitution of chloroplast ferredoxin with a bacterial flavodoxin. Proc. Natl. Acad. Sci. U.S.A. 104, 11495–11500. doi: 10.1073/pnas.0704553104
Tripathi, D. K., Bashri, G., Shweta, Singh, S., Ahmad, P., Singh, V. P., et al. (2017a). “Efficacy of silicon against aluminum toxicity in plants,” in Silicon in Plants: Present and Future Prospects, eds D. K. Tripathi, V. P. Singh, P. Ahmad, D. K. Chauhan, and S. M. Prasad (CRC Press; Taylor and Francis), 355–366.
Tripathi, D. K., Shweta, Singh, S., Yadav, V., Arif, N., Singh, S., et al. (2017b). “Silicon: a potential element to combat adverse impact of UV-B in plants,” in UV-B Radiation: From Environmental Stressor to Regulator of Plant Growth, eds V. P. Singh, S. Singh, S. M. Prasad, and P. Parihar (Hoboken, NJ: John Wiley & Sons), 175–195.
Tripathi, D. K., Singh, S., Singh, S., Chauhan, D. K., Dubey, N. K., and Prasad, R. (2016a). “Silicon as a beneficial element to combat the adverse effect of drought in agricultural crops,” in Water Stress and Crop Plants: A Sustainable Approach, ed P. Ahmad (Chichester: John Wiley & Sons), 682–694.
Tripathi, D. K., Singh, S., Singh, S., Mishra, S., Chauhan, D. K., and Dubey, N. K. (2015). Micronutrients and their diverse role in agricultural crops: advances and future prospective. Acta Physiol. Plantar. 37, 1–14. doi: 10.1007/s11738-015-1870-3
Tripathi, D. K., Singh, S., Singh, V. P., Prasad, S. M., Chauhan, D. K., and Dubey, N. K. (2016b). Silicon nanoparticles more efficiently alleviate arsenate toxicity than silicon in maize cultiver and hybrid differing in arsenate tolerance. Front. Environ. Sci. 4:46. doi: 10.3389/fenvs.2016.00046
Tripathi, D. K., Singh, S., Singh, V. P., Prasad, S. M., Dubey, N. K., and Chauhan, D. K. (2017c). Silicon nanoparticles more effectively alleviated UV-B stress than silicon in wheat (Triticum aestivum) seedlings. Plant Physiol. Biochem. 110, 70–81. doi: 10.1016/j.plaphy.2016.06.026
Tripathi, D. K., Singh, V. P., Chauhan, D. K., Prasad, S. M., and Dubey, N. K. (2014). “Role of macronutrients in plant growth and acclimation: recent advances and future prospective,” in Improvement of Crops in the Era of Climatic Changes, eds P. Ahmad, M. R. Wani, M. M. Azooz, and L.-S. P. Tran (New York, NY: Springer), 197–216.
Tripathi, D. K., Singh, V. P., Kumar, D., and Chauhan, D. K. (2012). Impact of exogenous silicon addition on chromium uptake, growth, mineral elements, oxidative stress, antioxidant capacity, and leaf and root structures in rice seedlings exposed to hexavalent chromium. Acta Physiol. Plantar. 34, 279–289. doi: 10.1007/s11738-011-0826-5
Tripathi, D. K., Singh, V. P., Prasad, S. M., Dubey, N. K., Chauhan, D. K., and Rai, A. K. (2016c). LIB spectroscopic and biochemical analysis to characterize lead toxicity alleviative nature of silicon in wheat (Triticum aestivum L.) seedlings. J. Photochem. Photobiol. B: Biol. 154, 89–98. doi: 10.1016/j.jphotobiol.2015.11.008
Tripathi, D. K., Tripathi, A., Gaur, S., Singh, S., Singh, Y., Vishwakarma, K., et al. (2017d). Uptake, accumulation and toxicity of silver nanoparticle in autotrophic plants, and heterotrophic microbes: a concentric review. Front. Microbiol. 8:7. doi: 10.3389/fmicb.2017.00007
Ueno, D., Rombola, A. D., Iwashita, T., Nomoto, K., and Ma, J. F. (2007). Identification of two new phytosiderophores secreted by perennial grasses. New Phytol. 174, 304–310. doi: 10.1111/j.1469-8137.2007.02056.x
Vaculík, M., Landberg, T., Greger, M., Luxová, M., Stoláriková, M., and Lux, A. (2012). Silicon modifies root anatomy, and uptake and subcellular distribution of cadmium in young maize plants. Ann. Bot. 110, 433–443. doi: 10.1093/aob/mcs039
Varotto, C., Maiwald, D., Pesaresi, P., Jahns, P., Salamini, F., and Leister, D. (2002). The metal ion transporter IRT1 is necessary for iron homeostasis and efficient photosynthesis in Arabidopsis thaliana. Plant J. 31, 589–599. doi: 10.1046/j.1365-313X.2002.01381.x
Vernay, P., Gauthier-Moussard, C., and Hitmi, A. (2007). Interaction of bioaccumulation of heavy metal chromium with water relation, mineral nutrition and photosynthesis in developed leaves of Lolium perenne L. Chemosphere 68, 1563–1575. doi: 10.1016/j.chemosphere.2007.02.052
Vert, G., Grotz, N., Dédaldéchamp, F., Gaymard, F., Guerinot, M. L., Briat, J. F., et al. (2002). IRT1, an Arabidopsis transporter essential for iron uptake from the soil and for plant growth. Plant Cell 14, 1223–1233. doi: 10.1105/tpc.001388
Viets, F. G. Jr. (1972). “Water deficits and nutrient availability,” in Water Deficits and Plant Growth, Vol. III: Plant Responses and Control of Water Balance, ed T. T. Kozlowski (New York, NY: Academic Press), 217–240.
Wang, H. F., Zhang, J. H., Liang, J. H., and Yin, W. L. (1999). Root and xylem ABA changes in response to soil drying in two woody plants. Chin. Sci. Bull. 44, 2236–2241. doi: 10.1007/BF02885929
Waraich, E. A., Ahmad, R., and Ashraf, M. Y. (2011). Role of mineral nutrition in alleviation of drought stress in plants. Aus. J. Crop Sci. 5, 764–777.
Waraich, E. A., Ahmad, R., Halim, A., and Aziz, T. (2012). Alleviation of temperature stress by nutrient management in crop plants: a review. J. Soil Sci. Plant Nutr. 12, 221–244. doi: 10.4067/S0718-95162012000200003
Waters, B. M., Blevins, D. G., and Eide, D. J. (2002). Characterization of FRO1, a pea ferric-chelate reductase involved in root iron acquisition. Plant Physiol. 129, 85–94. doi: 10.1104/pp.010829
Wehner, D. J., and Haley, J. E. (1990). Iron fertilization of Kentucky bluegrass. Commun. Soil Sci. Plant Anal. 21, 629–637. doi: 10.1080/00103629009368258
Wei Jin, C., You, G. Y., and Zheng, S. J. (2008). The iron deficiency-induced phenolics secretion plays multiple important roles in plant iron acquisition underground. Plant Signal Behav. 3, 60–61. doi: 10.4161/psb.3.1.4902
Weiss, J. V., Emerson, D., Backer, S. M., and Megonigal, J. P. (2003). Enumeration of Fe (II)-oxidizing and Fe (III)-reducing bacteria in the root zone of wetland plants, implications for a rhizosphere iron cycle. Biogeochemistry 64, 77–96. doi: 10.1023/A:1024953027726
White, P. J., and Brown, P. H. (2010). Plant nutrition for sustainable development and global health. Ann. Bot. 105, 1073–1080. doi: 10.1093/aob/mcq085
Whitehead, D. C., Dibb, H., and Hartley, R. D. (1981). Extractant pH and the release of phenolic compounds from soils, plant roots and leaf litter. Soil Biol. Biochem. 13, 343–348. doi: 10.1016/0038-0717(81)90074-2
Whitehead, D. C., Dibb, H., and Hartley, R. D. (1983). Bound phenolic compounds in water extracts of soils, plant roots and leaf litter. Soil Biol. Biochem. 15, 133–136. doi: 10.1016/0038-0717(83)90092-5
Wojtaszek, P. (1997). Oxidative burst: an early plant response to pathogen infection. Biochem. J. 322, 681–692. doi: 10.1042/bj3220681
Xin-Bin, Z., and Wei-Ming, S. (2007). Effect of root surface iron plaque on Se translocation and uptake by Fe-deficient rice. Pedosphere 17, 580–587. doi: 10.1016/S1002-0160(07)60068-X
Xiong, L., and Zhu, J. K. (2002). Molecular and genetic aspects of plant responses to osmotic stress. Plant Cell Environ. 25, 131–139. doi: 10.1046/j.1365-3040.2002.00782.x
Xu, Z., and Zhou, G. (2008). Responses of leaf stomatal density to water status and its relationship with photosynthesis in a grass. J. Exp. Bot. 59, 3317–3325. doi: 10.1093/jxb/ern185
Yadav, S. K. (2010). Heavy metals toxicity in plants: an overview on the role of glutathione and phytochelatins in heavy metal stress tolerance of plants. South Afr. J. Bot. 76, 167–179. doi: 10.1016/j.sajb.2009.10.007
Yadav, S. S., Verma, A., Sani, S., and Sharma, Y. K. (2007). Likely amelioration of chromium toxicity by Fe and Zn in maize. J. Ecophysiol. Occup. Health 7, 111–117.
Yadav, V., Arif, N., Singh, S., Srivastava, P. K., Sharma, S., Tripathi, D. K., et al. (2016). Exogenous mineral regulation under heavy metal stress: advances and prospects. Biochem. Pharmacol. 5:220. doi: 10.4172/2167-0501.1000220
Yamagata, N., and Shigematsu, I. (1970). Cadmium pollution in perspective. Bull. Inst. Public Health 19, 1–27.
Yamaguchi, M., Valliyodan, B., Zhang, J., Lenoble, M. E., Yu, O., Rogers, E. E., et al. (2010). Regulation of growth response to water stress in the soybean primary root. I. Proteomic analysis reveals region-specific regulation of phenylpropanoid metabolism and control of free iron in the elongation zone. Plant Cell Env. 33, 223–243. doi: 10.1111/j.1365-3040.2009.02073.x
Yang, L., Li, Y., Yang, X., Xiao, H., Peng, H., and Deng, S. (2011). Effects of iron plaque on phosphorus uptake by Pilea cadierei cultured in constructed wetland. Proce. Env. Sci. 11, 1508–1512. doi: 10.1016/j.proenv.2011.12.227
Yasar, F., Turkmen, S., and Ellialtioglu, S. (2006). Determination of antioxidant activities in some melon (Cucumis melo L.) varieties and cultivars under salt stress. J. Hortic. Sci. Biotec. 81, 627–630. doi: 10.1080/14620316.2006.11512115
Yizong, H., Ying, H., and Yunxia, L. (2009). Heavy metal accumulation in iron plaque and growth of rice plants upon exposure to single and combined contamination by copper, cadmium and lead. Acta Ecol. Sin. 29, 320–326. doi: 10.1016/j.chnaes.2009.09.011
Young, M. F., Glahn, R. P., Ariza-Nieto, M., Inglis, J., Olbina, G., Westerman, M., et al. (2009). Serum hepcidin is significantly associated with iron absorption from food and supplemental sources in healthy young women. Am. J. Clin. Nutr. 89, 533–538. doi: 10.3945/ajcn.2008.26589
Yousfi, S., Wissal, M., Mahmoudi, H., Abdelly, C., and Gharsalli, M. (2007). Effect of salt on physiological responses of barley to iron deficiency. Plant Physiol. Bioch. 45, 309–314. doi: 10.1016/j.plaphy.2007.03.013
Yust, A. K., Wehner, D. J., and Fermaaian, T. W. (1984). Foliar applications of N and Fe to Kentucky bluegrass. Agron. J. 76, 936–938. doi: 10.2134/agronj1984.00021962007600060017x
Zargar, S. M., Kurata, R., Inaba, S., and Fukao, Y. (2013). Unraveling the iron deficiency responsive proteome in Arabidopsis shoot by iTRAQ-OFFGEL approach. Plant Signal. Behav. 8:e26892. doi: 10.4161/psb.26892
Keywords: Trace elements, iron (Fe), abiotic stress, plants, reactive oxygen species (ROS), enzymatic antioxidants, proteins, gene families
Citation: Tripathi DK, Singh S, Gaur S, Singh S, Yadav V, Liu S, Singh VP, Sharma S, Srivastava P, Prasad SM, Dubey NK, Chauhan DK and Sahi S (2018) Acquisition and Homeostasis of Iron in Higher Plants and Their Probable Role in Abiotic Stress Tolerance. Front. Environ. Sci. 5:86. doi: 10.3389/fenvs.2017.00086
Received: 29 June 2016; Accepted: 24 November 2017;
Published: 09 February 2018.
Edited by:
Naser A. Anjum, University of Aveiro, PortugalReviewed by:
Vijay Shridhar, Doon University, IndiaCatherine Santaella, UMR7265 Biologie végétale et microbiologie environnementales (BVME), France
Copyright © 2018 Tripathi, Singh, Gaur, Singh, Yadav, Liu, Singh, Sharma, Srivastava, Prasad, Dubey, Chauhan and Sahi. This is an open-access article distributed under the terms of the Creative Commons Attribution License (CC BY). The use, distribution or reproduction in other forums is permitted, provided the original author(s) and the copyright owner are credited and that the original publication in this journal is cited, in accordance with accepted academic practice. No use, distribution or reproduction is permitted which does not comply with these terms.
*Correspondence: Durgesh K. Tripathi, ZGt0cmlwYXRoaWF1QGdtYWlsLmNvbQ==
Vijay P. Singh, dmlqYXlwcmF0YXAuYXVAZ21haWwuY29t
Devendra K. Chauhan, ZGtjaGF1aGFuYXVAeWFob28uY29t