- 1TERRA Teaching and Research Centre, Gembloux Agro-Bio Tech, University of Liège, Gembloux, Belgium
- 2UMR Ecosys, INRA AgroParisTech, University Paris Saclay, CNRS, Thiverval Grignon, France
The limitations of conventional agriculture have accelerated the need for a transition to an environmentally and economically sustainable agricultural model. In this regard, the role played by soil organic matter (SOM) is key. Here, we aimed to study the impact of permaculture and biointensive micro-gardening practices, characterized by intensive cultivation, the use of large and localized organic inputs and the non-use of mineral fertilizers and pesticides, on soil physicochemical properties and SOM distribution in aggregate-size fractions. The physicochemical properties of soils in permaculture farming implemented for 7 years were compared with a soil under pasture. A soil experiencing conventional agriculture practices in similar geopedoclimatic conditions was simultaneously studied. Soils were separated into four aggregate-size fractions, into which organic carbon (OC) concentrations have been measured. The major soil physicochemical properties were measured on the bulk soils. The concentrations of total OC and nitrogen (N) in bulk soils were higher under permaculture practices, due to significant inputs of manure and compost, resulting in higher concentrations of the bioavailable nutrients Ca, Mg, K, and P. The permaculture practices sustained a level of macroaggregation similar to that of the pasture, and well above that of conventional practices. OC concentrations increased in the 250–2,000, 50–250 μm and occluded 50–250 μm fractions, but not in the <50 μm fraction compared to pasture. This can be explained by the too short duration of permaculture practices for allowing the decomposition of coarse particulate organic matter (POM) into fine sized SOM that is more associated with mineral particles. The higher OC stocks in permaculture are therefore mainly governed by the coarse POM fraction. Our results show that permaculture/biointensive micro-gardening practices enhance SOM storage and modify the distribution of SOM in aggregate-size fractions, while substantially improving nutrient bioavailability and suggest that these practices strongly affect soil properties. The effects of permaculture practices on soil properties would certainly vary depending on geopedoclimatic context, justifying the need to implement the approach for other soil types. Finally, we recommend to compare biogeochemical budget and nutrients use efficiency of permaculture gardening with conventional horticulture gardening.
Introduction
Agriculture is probably the human activity that has the greatest impact on terrestrial ecosystems, given its global scale (Hathaway, 2016). Increasing awareness about the environmental limits of modern agriculture(Altieri and Nicholls, 2009; Stoate et al., 2009) has led to the need for a transition toward a more resilient and biodiverse agriculture model (Ferguson and Lovell, 2014). In this regard, micro- or mini-farms, inspired by the permaculture model (Mollison and Holmgren, 1990; Holmgren, 2002) and biointensive micro-gardening (Coleman, 1995; Jeavons, 1995), could potentially play a key role in this transition (Morel et al., 2017).
Permaculture and biointensive micro-gardening practices aim to optimize the interactions in the soil-plant system for an efficient use of their ecological functions and ecosystem services (Mollison and Holmgren, 1990), while realizing high yields from a small area and sustaining soil fertility (Jeavons, 2001). The main principles are: (1) to work manually to optimize plant densities (2) to input significant amounts of organic materials and (3) not to use mineral fertilizers or pesticides (Hathaway, 2016). This intensification can be further strengthened by a combination of species that allows the vertical stratification of crops. These practices contrast with conventional agriculture systems that rely mainly on monospecific production, the use of mineral fertilizers and pesticides, and mechanized tillage (Hathaway, 2016). Permaculture systems have largely been overlooked by soil scientists, and have generally been ignored in scientific studies (Ferguson and Lovell, 2014). Indeed, although there has been an increase in the number of publications concerning permaculture since 2008, only 23.1% are about “life sciences” (Ferguson and Lovell, 2014), and are even more rarely about soil science. Nevertheless, these systems have potentially limited impacts on ecosystems compared to conventional agriculture, and have also proven to be economically viable in some cases (Morel et al., 2017).
Practices can differ greatly from one permaculture/biointensive micro-gardening system to another, but are almost always characterized by the use of organic fertilizers (Altieri and Nicholls, 2009; Hathaway, 2016). Soil organic matter (SOM) plays a fundamental role in soil, functioning by maintaining fertility through nutrients recycling (Diacono and Montemurro, 2010; Murphy, 2015), as well as increasing nutrient retention. Furthermore, organic matter (OM) is one of the major contributor to soil aggregation, which is essential for maintaining soil resilience to physical stresses, and enhancing C storage through physical protection (Six et al., 2002). SOM is a continuum of progressively decomposing (by oxidative depolymerization) organic compounds (Lehmann and Kleber, 2015). Progressive decomposition, along with an increasing reactivity of organic molecules with mineral phases, are involved in the soil aggregation processes, leading to the SOM physicochemical protection against enzymatic activity. Therefore, it is crucial to test the extent to which permaculture practices, characterized by significant and localized organic inputs, can sustain nutrient release (Kopittke et al., 2017; Sarker et al., 2018), while modifying OC stocks and distribution in aggregate-size fractions (Chenu et al., 2018).
Based on Tisdall and Oades (1982) conceptual model for soil aggregate formation, Six et al. (1998, 2000) developed fractionation methods in order to obtain different physical units (macroaggregates >250 μm, microaggregates <250 μm and silt and clay <50 μm), and to isolate their components (mineral-associated vs. particulate organic matter, i.e., POM). These methods have allowed the subdivision of SOM according to different stabilization processes, and have been widely used to help understand the impact of land-use and agriculture practices on SOM dynamics and C-protection mechanisms (Denef et al., 2004; Abiven et al., 2009; Devine et al., 2014; Paradelo et al., 2016). Organic input into soil is commonly known to enhance soil aggregation and SOM content (Gulde et al., 2008; Abiven et al., 2009; Diacono and Montemurro, 2010; Virto et al., 2012). Nevertheless, some studies have shown that C-rich soils may not present an increase in OC content after increasing the C input (Campbell et al., 1991; Gulde et al., 2008). Furthermore, Gulde et al. (2008) showed that, as C input increases, the mineral-associated fraction saturates, and additional input accumulates as labile C fractions, mainly through coarse POM, with a relatively high turnover.
Here, we aim to study the impact of permaculture and biointensive micro-gardening practices on soil physicochemical properties, SOM contents and SOM distribution in aggregate-size fractions. In this regard, we compared three types of permaculture practices with the previous land use: a pasture. This is implemented simultaneously with the study of a conventional agricultural field developed under similar geopedoclimatic conditions.
Materials and Methods
Study Plots
This study was carried out near the village of Le Bec-Hellouin (Normandie, France; 49°13′N, 0°43′E), which experiences an oceanic climate with a mean annual rainfall ranging from 700 to 900 mm. Under these conditions, five different plots were studied, four located at Bec-Hellouin farm (three cultivated under permaculture practices, and one grazed, i.e., pasture) and one located 2 km north of the Bec-Hellouin farm (conventional agriculture plot). General description of the five different plots are provided in Table 1 and the locations are shown in Figure 1.
Bec-Hellouin Farm
The Bec-Hellouin farm was created in 2004. The cultural practices are directly based on the permaculture model (Mollison and Holmgren, 1990; Holmgren, 2002) and biointensive micro-gardening (Coleman, 1995; Jeavons, 1995, 2001). They cultivate intensively and thoroughly on small plots without mechanized assistance, use only organic amendments and no pesticides or mineral fertilizers, and achieve high yields (55 € m−2 on average; Guégan and Leger, 2015). The total cultivated area is about 0.1 ha in a total farm size of about 1 ha, which means that only 10% of the total surface area is cultivated. The farm is composed of different production plots and one grazed pasture. Each is differently managed, and so required individual soil analyses for the present study. We selected the most representative plots of the permaculture farm—mounds, beds, and forest garden (Figure 1). Table 2 presents the specific characteristics of cultivation for the three permaculture plots. As a comparison for organic input (225–330 t ha−1 yr−1 for permaculture mounds and beds), the average for the Basse-Normandie region in organic gardening systems is about 30 t ha−1 yr−1 of fresh manure (Association Bio Normandie, pers. comm.). Thus, at the Bec-Hellouin farm, the OM input is very high and localized in a small area, compared to the total size of the farm. The soil parent material for the four sampled plots located on the Bec-Hellouin farm consists of modern carbonated alluvium, deposited by the river Le Bec. This soil is classified as Eutric Cambisol (Siltic) (IUSS, 2014).
The plot of permaculture mounds lies on an artificial island under intense cultivation. The mounds are 0.8–1.2 m wide, 12 m long, and 0.3–0.5 m high, and are mulched twice a year. Crop association is practiced (cabbage-bean-lettuce, garlic-lettuce, bean-spinach). Calcareous liming was performed once.
The plot of permaculture beds represents a cultivated area of 116 m2 under intense cultivation, with one row every 6 cm. Crop combination is also practiced (radish-carrot-bean, turnip-parsnip-bean, carrot-leek). Calcareous liming was performed once.
The 1,160 m2 plot of forest garden was created based on the concept of vertical layers (Crawford and Aitken, 2013). The tree stratum is relatively open, and comprises apple trees, elder, pear trees, and common dogwood. The shrub stratum is much denser, and is made up of gooseberries, raspberries, blackcurrants, and hazel shrubs. The herbaceous stratum is mostly represented by nettles and comfrey. This plot receives less attention from the farmers, compared to mounds and beds. The permaculture forest garden plot received only one organic amendment 7 years ago as 10 cm thickness of mature compost.
The pasture plot also belongs to the Bec-Hellouin farm. Over an area of 3.4 ha, 20 sheeps, one horse and one donkey graze from March to November. No improvement has been performed on this plot, at least since the launch of the farm in 2004. This plot represents the situation of the three permaculture plots prior to their cultivation.
Conventional Agriculture
No horticulture crops were identified in the area under the same geopedoclimatic conditions, hence another reference was selected as a conventionally managed field crop. The field under conventional practices is managed by another farmer, and is geared toward the production of cereal and protein crops (soft wheat, barley, rape, and peas). The crop rotation is triennial wheat/peas/wheat. The soil is superficially plowed (to 20 cm depth), and fertilization is in mineral form. Pesticides are applied, particularly fungicides on the wheat crops (three times per crop). The soil is developed from a colluvial carbonated deposit, and is also classified as Eutric Cambisol (Siltic) (IUSS, 2014). Given the small textural variation, this plot was only used as a benchmark for conventional agriculture practices in similar geopedoclimatic context but cannot be considered as a land-use evolution from the studied pasture, as it was assumed for the permaculture plots.
Soil Sampling
The soils were sampled at 0–20 cm soil depth with an auger in July 2016. For each of the five plots, soil samples were collected in triplicates. For each of the three replicates, three subsamples were taken as composite samples. In the permaculture mounds and beds, one sample corresponded to one mound or one bed. In pasture and conventional fields, sampling has been carried out in three different zones randomly selected. For the conventional plot, sampling was performed in the third year of the rotation, under wheat cultivation.
Soil Analysis
Physicochemical Characterization
All analyses were performed on the 0–20 cm soil sample. The particle-size distribution of air-dried fine earth was measured by the gravity sedimentation method (NF X11-681). The OM was destroyed using hydrogen peroxide. The sand fraction was separated from the fine earth by ultrasonic dispersion and wet sieving. The clay and silt fractions were then collected by successive sedimentation. The pH of the soil water was determined using distilled water (mass ratio soil:solution 1:5; ISO 10390). The pH of the soil KCl was measured using KCl 1M, employing the same protocol as for the water pH. The bioavailable major nutrients (P, Ca, Mg, K) were extracted using ammonium acetate-EDTA 1M (pH 4.65; Lakanen and Erviö, 1971), and measured by atomic absorption spectroscopy (Ca, Mg, and K) or by spectrophotometry (P). The cation exchange capacity (CEC) was measured by percolating soil columns with 1M ammonium acetate, buffered at pH 7 (Metson, 1956). The excess ammonium was rinsed with ethanol, and the solution was alkalized with 50% sodium hydroxide, then distilled. Finally, the solution was titrated with 0.1M hydrochloric acid. The exchangeable cations were measured by atomic absorption spectroscopy. Base saturation (BS) was calculated as the ratio between the sum of the exchangeable cations and the CEC.
X-Ray Diffraction Analysis
X-ray diffraction analysis was performed using a Bruker D8-Advance Eco diffractometer with a Cu-anode (Univ. Liège) on the pasture, conventional and two permaculture plots (forest garden and beds). The sample analyzed was a mixture of the three composite samples from each plot. Bulk soil was used for the analysis, without orientation. An estimation of the abundance of the different minerals was then assessed with the software TOPAS.
Soil Bulk Density
Soil bulk density measurements were performed on the 0–20 cm soil sample. To assess OC stocks in the studied plots, the soil bulk densities were measured using a modified excavation method (Blake and Hartge, 1986). Soil was extracted from a 20-cm-deep by 15-cm-diameter pit. A plastic bag was placed in the pit and filled with sand (of a known volume). The extracted soil was then dried at 50°C, weighed and sieved at 2,000 μm. The stones remaining on the sieve were dried at 50°C, weighed and their volume measured by immersion in a graduated column. The stone content was calculated as:
where S is the stone content (%), Vs is the volume of the stones (cm3) and Vtot is the total excavated volume (cm3). Then, the soil bulk density can be calculated as:
where δ is soil bulk density (g cm−3), WTOTis the total weight of the excavated soil and VTOT is the total volume of the excavated soil.
C Stock Calculation
Given that cultural practices can modify soil bulk density, it would be inaccurate to compare OC stocks at the same depth for all of the samples; these should be measured on an equivalent soil mass for each sample. Hence, a corrected depth was calculated (Solomon et al., 2002). The pasture plot was chosen for calculation of the corrected depth because it represents the situation prior to cultivation of the three permaculture plots. The corrected depth was calculated as:
where Zcorrected sample is the corrected depth of the sample (cm) and Zpasture is the depth of the pasture sample (cm). Given that the corrected depths of the permaculture soils would be deeper than that of the pasture (lower bulk densities), and that soil analysis was performed on the 0–20 cm sample, we chose to correct only the top 10 cm of the pasture, so as to avoid corrected depths deeper than 20 cm. Hence, the soil mass chosen to calculate OC stocks on for all of the samples came from the bulk density of the pasture to a depth of 10 cm.
The stocks were calculated as:
where StockOC is the stock of OC by unit area (kg m−2) and [OC] is the OC concentration of the sample (g kg−1).
Soil Aggregate-Size Fraction Separation
Soil aggregate-size fraction separations were performed on air-dried soil samples using a wet-sieving method adapted from Elliott (1986) and Six et al. (1998). Briefly, 80 g of bulk soil sieved at 2 mm was placed on a 250 μm sieve submerged in deionized water for 5 min at room temperature. The soil was then sieved by moving the sieve out of the deionized water, and then immersing it again 50 times over 2 min. The same process was repeated again with a 50 μm sieve, in order to obtain three fractions: (1) 250–2,000 μm; (2) 50–250 μm; and (3) <50 μm (Figure 2). All fractions were dried in an oven at 60°C, then weighed in order to determine the mass of each aggregate-size fraction. As sand presents almost no binding potential with OM, it was necessary to remove its weight from the size fractions (Six et al., 2000). The sand content of the 250–2,000 μm fraction was considered to be the coarse sand content (200–2,000 μm) from the particle-size distribution, and the sand content of the 50–250 μm fraction was considered to be the fine sand (50–200 μm) content, based on the particle-size distribution. This approximation slightly overestimated the 250–2,000 μm fraction proportion, and underestimated the 50–250 μm fraction proportion.
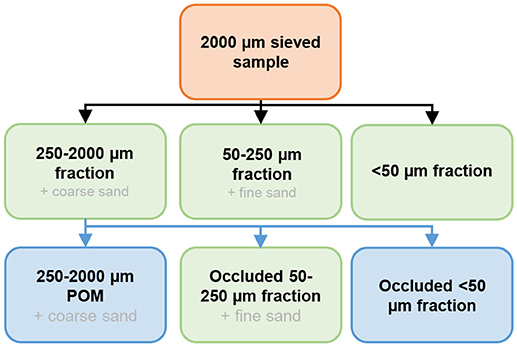
Figure 2. Schematic representation of the soil aggregate-size fraction separation method. Green rectangles represent the pools in which OC concentrations were measured. Black arrows represent the wet sieving method and blue arrows represent 250–2,000 μm fraction separation.
Then, the different components of the 250–2,000 μm fraction (coarse POM + macroaggregates) were mechanically isolated (Six et al., 2000). Briefly, 10 g of 250–2,000 μm fraction were placed on top of a 250 μm screen and shaken with 50 glass beads (4 mm in diameter, 10–15 min) under a continuous flow of water. A 50 μm sieve was placed at the outlet of the system in order to obtain three distinct fractions: (1) 250–2,000 μm coarse POM; (2) occluded 50–250 μm; and (3) occluded <50 μm (Figure 2). The 250–2,000 POM content was corrected for the coarse sand content, and the occluded 50–250 μm fraction for the fine sand content, as discussed above.
The OC concentrations were measured in four fractions (indicated in green in Figure 2)−250–2,000, 50–250, <50 μm and occluded 50–250 μm—by flash-dry combustion. In order to combine the effect of the soil aggregate-size fraction distribution and OC concentrations, we calculated the OC distribution in each fraction per unit mass of bulk soil. This allowed the study of the contribution of the different fractions to the total OC concentrations and stocks in the bulk soils:
The OC distribution of the 250–2,000 μm fraction actually represented the sum of the three distinct OM fractions mentioned above: 250–2,000 POM, occluded 50–250 μm and occluded <50 μm; however, we measured OC concentration only in the occluded 50–250 μm fraction (Figure 2). Therefore, we assumed that the OC concentration in the occluded <50 μm fraction was the same as the one from the <50 μm fraction. Finally, the OC distribution in the 250–2,000 POM fraction per unit mass of bulk soil was measured as follows:
where OC distributionF250−2000 is the OC concentration in the 250–2,000 fraction per unit mass of bulk soil, OC distributionFO50−250 is the OC concentration in the occluded 50–250 μm fraction per unit mass of bulk soil and OC distributionFO <50 is the OC concentration in the occluded <50 μm fraction per unit mass of bulk soil. All of the OC concentrations in the equation are expressed as a function of the bulk soil, in grams of C in the specific fraction per kilogram of bulk soil.
Results
OC Concentrations and Stocks in Bulk Soils
The permaculture soils had significantly higher OC concentrations in the 0–20 cm soil layer (between 60.3 ± 4.3 and 73.0 ± 5.4 g kg−1) than those of the pasture (49.1 ± 3.6 g kg−1) and conventional field (11.0 ± 0.9 g kg−1), as well as higher stocks—between 6.3 ± 0.5 and 7.7 ± 0.6 kg m−2 in the permaculture soils, 5.2 ± 0.4 kg m−2 in the pasture and 1.2 ± 0.1 kg m−2 in the conventional plot (Table 3). The permaculture forest garden plot was characterized by a significantly lower OC concentration and stock than the two other permaculture plots. Total N concentrations were significantly higher in permaculture mounds and beds (6.2 ± 0.1 and 6.3 ± 0.1 g kg−1, respectively) compared to the pasture (5.5 ± 0.4 g kg−1). In the conventional plot, N concentration was much lower, at 1.1 ± 0.1 g kg−1. Finally, the C/N ratios were higher in all the permaculture soils (10.5–11.5) than in the pasture (8.9). The conventional system presented an intermediate C/N value of 9.7.
Particle-Size Distributions and Soil pH
The soils of the five sampled plots were characterized by a quite homogeneous particle-size distribution (Table 4). According to the USDA's Soil Survey Laboratory Staff (2017), all soils are silt loam. Tukey's test showed no significant differences in the finest particle-sizes (clay and fine silt); however, the conventional plot presented a higher content of coarse silt (+10–13%) and a lower content of coarse sand (−7%) than the four other plots. The soil pHH2O varied between 6.6 (pasture) and 7.4 (permaculture mounds and beds), which were the only two groups significantly differentiated by Tukey's test. The soil pHKCl presented values between 5.6 (pasture) and 7.0 (permaculture mounds), and was significantly lower in the pasture than in the permaculture soils. Finally, the difference between pHH2O and pHKCl was significantly higher for pasture (1.0) compared to the three permaculture soils (0.5). The conventional plot had no differences between pHH2O and pHKCl.
Mineralogical Characterization
The studied soils comprised quartz (70–76%), plagioclase (8–11%), K-feldspar (3–5%), illite (6–8%), and vermiculite (5–6%) (Table 5). In addition, the permaculture plots contained calcite (2–3%), while the pasture and conventional plots contained c. 1% amphibole. Given that no replicates were tested, we cannot conclude that no significant differences exist among the plots; however, we observed homogeneity in the mineralogy of the studied soils, despite difference in the soil parental material between the conventional plot (alluvial origin) and the others (colluvial origin).
Bioavailable Nutrients, CEC, and Exchangeable Cations
The concentrations of bioavailable nutrients extracted with ammonium acetate-EDTA were significantly higher in the three permaculture plots than in the pasture and conventional plots (Table 6). According to Genot et al. (2007), the permaculture plots presented “very high” bioavailable concentrations for all four elements (>364 mg 100 g−1 for Ca, >25.9 mg 100 g−1 for Mg, >41.6 mg 100 g−1 for K, and >13.1 mg 100 g−1 for P); however, in the conventional and pasture systems, the concentrations were “very low” for K (<10.4 mg 100 g−1) and “low” for Mg (6.5–12.9 mg 100 g−1). Nevertheless, they were also classified as “very high” for Ca, probably due to the carbonated nature of the parent materials. The cation exchange capacity (Table 6) and the exchangeable cation concentrations (Ca2+, Mg2+, K+, Na+) were similar for all the permaculture plots and pasture. The CEC and exchangeable Ca2+ and Mg2+ concentrations were significantly lower in the conventional plot. The base saturation percentages were not significantly different among all plots, at nearly 100% and mostly saturated with Ca2+.
Soil Aggregate-Size Fraction Distributions
The permaculture practices led to a higher content of the 250–2,000 POM fraction (between 31.8 and 39.9% of the soil mass) compared to pasture (24.1%), at the expense of the 50–250 μm fraction: between 25.2 and 30.9% in the permaculture plots vs. 39.9% in the pasture (Figure 3). The proportions of <50 μm (18–20%), occluded 50–250 μm (9–12%) and occluded <50 μm fractions were not significantly different, with the exception of the occluded <50 μm proportion of the permaculture mounds (12.3 ± 2.6%) compared to the pasture (6.4 ± 0.4%). As expected, the aggregate-size fraction distribution in the conventional system was very different compared to that of the pasture and permaculture soils, with a much higher percentage of the 50–250 μm fraction (62.6 ± 6.4%), predominantly at the expense of the 250–2,000 fraction.
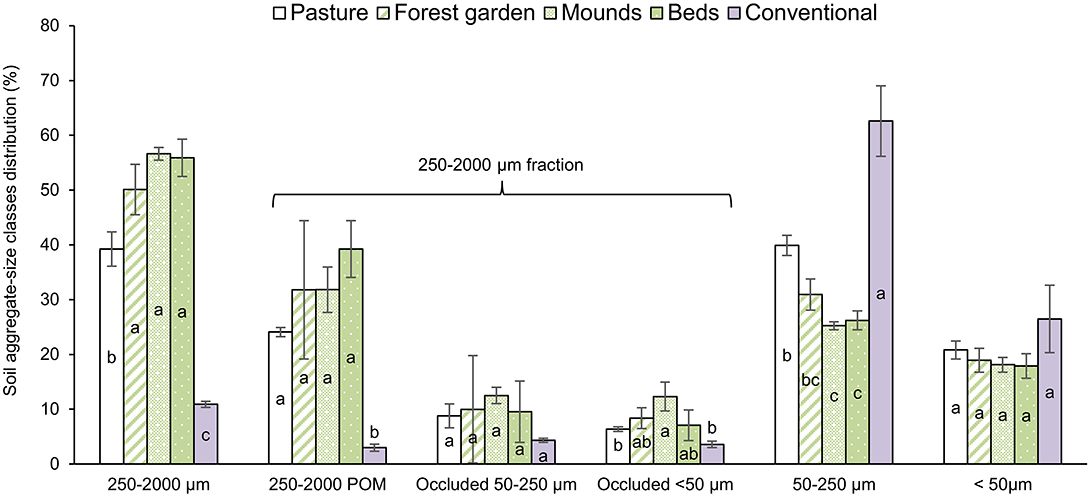
Figure 3. Sand-free distribution of soil aggregate-size fractions in the bulk soils (%). White columns represent the pasture plot, green columns the permaculture plots and purple columns the conventional plot. Various letters represent significant differences at the 95% confidence level (LSD Tukey; n = 3). Bars indicate standard deviation of the mean.
OC Concentrations in Soil Aggregate-Size Fractions
The OC concentrations were higher in three distinct size fractions after the implementation of permaculture practices-−250–2,000, 50–250 μm, and occluded 50–250 μm (Table 7). The difference of OC concentration between pasture and permaculture beds and mounds was c. 60 and 50% for the 250–2,000 μm and occluded 50–250 μm fractions, respectively. The difference was less important in the 50–250 μm fraction, and significant only for the permaculture mounds (+34%). The OC concentrations in the <50 μm fraction were equivalent in all permaculture plots and pasture (between 28.7 ± 10.7 and 37.0 ± 5.8 g kg−1), but three times lower in the conventional plot (10.2 ± 1.5 g kg−1). The permaculture forest garden also presented higher OC concentrations compared to the reference pasture plot, but it was significant only in one aggregate-size fraction—the occluded 50–250 μm fraction (+31%). OC concentrations in the conventional plot were much lower in all aggregate-size fractions (Table 7).
OC Distribution in Soil Aggregate-Size Fractions
The OC distribution in the soil aggregate-size fractions per unit mass of bulk soil are presented in Figure 4. OC concentrations in the permaculture bulk soils (Table 3) were higher compared to those of the pasture, mainly due to the 250–2,000 μm POM. To calculate the OC distributions in the soil aggregate-size fractions per unit mass of bulk soil (Figure 4), we hypothesized similar OC concentrations in free and occluded silt and clay fractions (Nicolás et al., 2014). However, Puget et al. (2000) showed that OC concentrations in the silt and clay fractions can vary depending on aggregate fraction (from 30 to 40 mg C g−1 for clay-size particles and from 4 to 7 mg C g−1 for silt-size fraction). However, despite potential variations, even if the OC concentration in the occluded <50 μm fraction was slightly higher than the one in the free <50 μm fraction, the stock differences between the permaculture plots and the pasture would still primarily be due to the 250–2,000 coarse POM. In the conventional plot, OC is mainly distributed in the 50–250 μm and <50 μm fractions.
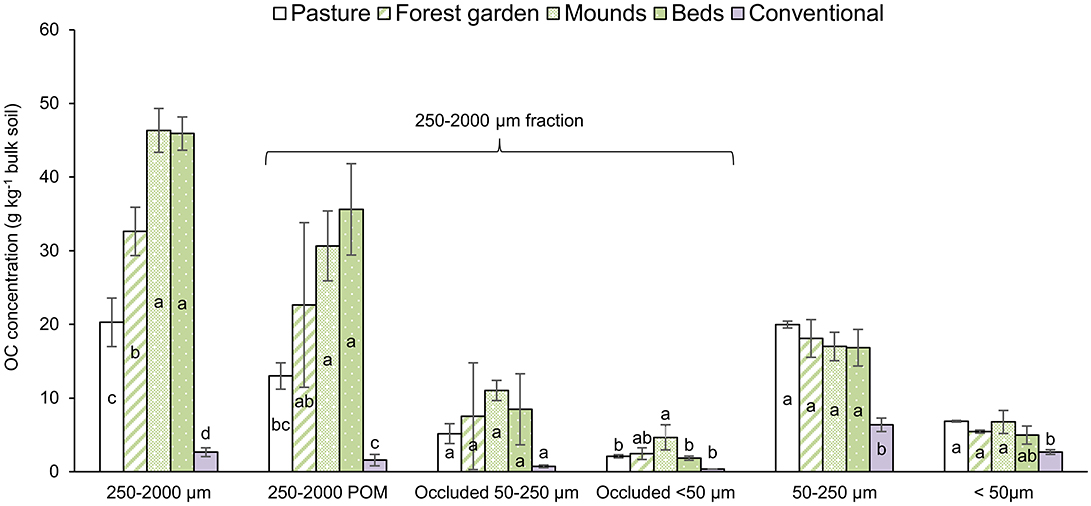
Figure 4. OC distributions in the soil aggregate-size fractions (in grams OC per kilo bulk soil). Various letters represent significant differences at the 95% confidence level (LSD Tukey; n = 3). Bars indicate standard deviation of the mean.
Discussion
Pedological Context of the Studied Plots
The higher content of coarse silt and lower content of coarse sand in the conventional plot compared to the others (Table 4) is probably due to the difference in parent material (colluvial vs. alluvial deposits). In addition, the conventional plot was located near a plateau (Figure 1), and may have received aeolian silty material. Nevertheless, X-ray diffraction analysis showed that the soil mineralogy is similar among all the studied plots (Table 5). The only difference is the presence of calcite in the permaculture plots, but not in the conventional and pasture plots. The permaculture plots can therefore be compared to pasture in terms of the evolution of soil properties. The conventional agriculture plot is used to compare the effect of conventional practices with alternative cropping practices given the similarity in particle-size distribution and mineralogy, but with the limit that horticulture plots are compared to cereal crop.
Increase in OC Storage After Implementation of Permaculture Practices
The permaculture soils have much higher OC concentrations and stocks compared to the pasture soil (between 1.2 and 1.5 times higher; Table 3). The initial input (a 10-cm layer of compost added during the first year), and the high annual exogenous organic input (225–330 t ha−1 yr−1) in the form of manure, explain such a large difference in OC concentrations and stocks. Application rates are usually lower (e.g., 3.2 t OC ha−1 yr−1 in Poulton et al., 2018 and 2 t OC ha−1 yr−1 in Peltre et al., 2012). The present very high inputs explain that SOC stocks in permaculture are higher that the one in the pasture after only 7 years (+ 17.3 t ha−1), compared to a 32 (Poulton et al., 2018) and 20-year-long experiment (Peltre et al., 2012). The absence of exogenous OM input explains the much lower OC stock in the conventional agriculture plot as ofen observed (e.g., Autret et al., 2016; Dignac et al., 2017). In the permaculture plots, the lower OC concentration and stock in the forest garden plot is also probably due to the absence of exogenous organic input. Indeed, higher organic inputs, as plant biomass or organic amendments, often explain increased SOC concentrations and stocks (Peltre et al., 2012; Virto et al., 2012; Chenu et al., 2018). Despite the absence of anthropogenic inputs in the forest garden plot, OC concentrations and stocks remained higher compared to the reference pasture plot, probably due the initial organic input (Table 2), the vertical stratification of the crops and the return of biomass through litterfall that is characteristic of this type of land use.
Effect of Permaculture Practices on Soil Physicochemical Properties
Soil pH (Table 4) in the pasture is generally lower than in the permaculture plots. Moreover, the significantly higher difference between pHH2O and pHKCl in the pasture plot compared to the permaculture plots reveals a greater amount of acid cations sorbed onto the exchange complex; while no significant differences were revealed among the base saturation percentages between the plots (c. 100%; Table 6).
The three times lower CEC in the conventional system compared to the others (Table 6) can be directly associated with the lower OC content (Table 3). Indeed, the clay content and mineralogy are similar among all the soils (Tables 4, 5). However, despite much higher concentrations of OC in the permaculture soils compared to the pasture, and similar clay contents and type, the CEC was the same—that is, c. 32 cmolc kg−1. This suggests than the difference in OC concentrations between permaculture and pasture soils can be attributed to POM, with a low CEC compared to finer size fractions in the soil, i.e., silt and clay (Oorts et al., 2003). Knowing the clay content and mineralogy, the bulk soil CEC and the OM content, we estimated the CEC of the soil organic matter in the different plots. It was high for the permaculture plots soil (c. 150–190 cmolc kg−1) as well as the pasture soil (~230 cmolc kg−1); indicating a contribution of fine sized SOM. Finally, the composition of the exchange complex had not changed among the permaculture soils and pasture. This is certainly due to the high content of Ca2+saturating the exchangeable complex given the carbonated nature of the parent material.
The mineralization of OM inputs in permaculture mounds and beds releases a high amount of nutrients into the soil. This phenomenon explains the much higher concentrations of bioavailable Ca, Mg, K, P in the permaculture systems compared to the pasture and conventional agriculture systems (Tables 3, 6). The lower concentration of bioavailable Ca in the conventional plot compared to the others is probably due to differences in parent material and soil depth. Indeed, the soils are slightly thinner in the valley (permaculture soils and pasture), and thus closer to the carbonated parent material, resulting in a higher bioavailable Ca concentration. It is worth noting that the forest garden plot has received no organic amendments but does show concentrations of bioavailable nutrients as high as the others (Table 6). This highlights the lower nutrient loss through biomass exportation and a more developed root system due to the vertical strata system, stimulating the weathering of parent material and the return of nutrients with litterfall (Jobbagy and Jackson, 2001; Lucas, 2001). In contrast, the low bioavailable nutrients concentrations in the conventional system are more surprising, given the soil fertilization. However, the soil-plant cycling of nutrients, added by mineral fertilizers, is probably not optimized given the losses from biomass export and leaching (N, P, and K in soluble form) compared to the slow release of nutrients from organic input by amendments (Diacono and Montemurro, 2010).
Effect of Organic Input on Soil Aggregation
Permaculture practices have enhanced the coarse POM content in the soils at the expense of the 50–250 μm fraction (so-called microaggregates and free fine POM). This observation is coherent, given that the exogenous organic input reached the soil in the form of free coarse POM. It is likely that such an increase in POM enhanced the 250–2,000 μm fraction proportion—so-called macroaggregates—(Abiven et al., 2009; Tejada et al., 2009; Annabi et al., 2011) even if we cannot affirm it in the framework of this work. On the other hand, the much larger 50–250 μm fraction proportion (the so-called microaggregates), at the expense of the 250–2,000 μm fraction proportion (the so-called macroaggregates) in the soil of the conventional system, could be explained by: (1) the absence of OC input, resulting in low OC concentrations in the bulk soil; and (2) the rapid turnover of macroaggregates due to tillage (Six et al., 2002), and exposure of the bare soil to rain events increasing disaggregation processes.
Increase in OC Stocks Ascribed to Coarse POM
While OC concentrations are higher in the 250–2,000 μm and occluded 50–250 μm fractions of the permaculture soils compared to the pasture, OC concentrations in the free silt & clay” (<50 μm) fraction are equivalent (Table 7). This can be explained by the fact that the duration of permaculture cultivation is certainly too short to allow a breakdown of the coarse manure and compost particles into finer ones that can interact with minerals. We may also note that the OC concentration in this fraction—that is, 29–30 g OC kg−1 (Table 7)—is quite high compared to literature: 12–15 g OC kg−1 silt + clay in a Haplic Calcisol (Nicolás et al., 2014), 20–40 g OC kg−1 clay and 3–7 g OC kg−1 silt in cultivated Eutric Cambisols (Puget et al., 2000), 15–20 g OC kg−1 silt + clay in a Petrocalcic Calcisol (Garcia-Franco et al., 2015), 18–22 g OC kg−1 silt + clay in a Lixisol (siltic) (Devine et al., 2014) and 15, 26, and 49 g OC kg−1 silt + clay in a Vermic Umbrisol cultivated for 55, 7, and 0 years respectively (Balesdent et al., 1998). Moreover, Gulde et al. (2008) clearly showed that OC concentrations in the silt + clay fraction was becoming constant, even with increasing manure input over 32 years. Such observations were made on a typical Chernozem (clayic loamic), and OC concentration in the silt + clay fraction was around 40 g kg−1. These observations could support the hypothesis of a C-saturation of the silt + clay fraction implying no additional accumulation of OM in this fine fraction (Hassink, 1997; Gulde et al., 2008). However, we obviously cannot demonstrate this assumption here and it deserves to be specifically studied.
In the permaculture plots, the relative increase in coarse POM (250–2,000 μm size; Figure 4) is the primary explanation for the higher SOC stocks, if we compare with pasture SOC stocks and OC distribution in fractions (Table 3). In addition, the CEC did not evolve in the permaculture plots compared to the pasture, consistent with a change in SOC mostly due to POM. Here, the permaculture practices have increased the SOM stocks through coarse POM, which is a labile SOC fraction, with a 3.1 ± 0.3 year residence time under temperate conditions (Balesdent, 1996), and thus is sensitive to mineralization in cases of temperature increases or land-use changes (e.g., Cheng et al., 2011; Poeplau and Don, 2013). Given the relatively very short time since the implementation of these practices, however, the OM in the fine fractions may progressively increase with time, even if this process is largely dependent on a hypothetical C-saturation of this fraction (Gulde et al., 2008), as discussed above.
Conclusions
The permaculture practices largely increase nutrient bioavailability, as well as SOC stocks, in the surface layer of the soil (≈20 cm). On average, SOC stocks were 1.73 kg m−2 (17.3 t ha−1) higher than those in the reference pasture plot. Aggregate-size distributions were somewhat modified by permaculture practices, but there was a very large increase in coarse POM, presumably deriving from manure application, which would explain most of the SOC stock difference. Thus, permaculture practices enable the storage of additional C in soils. The type of additional SOM is likely characterized by rapid turnover rates and is associated with large benefits in terms of soil physicochemical properties (essentially nutrient bioavailability and aggregation). The higher SOM content mainly as coarse POM suggests that OC does not really react with fine mineral fraction to form organo-mineral associations. This should be further investigated by studying the effect of permaculture practices implemented for a longer time. In the present study case, the available organic resources are concentrated over a very small surface area. An assessment of SOC stocks over the entire farm thus remains to be performed, to also account for transfers of crop residues from certain plots to produce the manure concentrated on the permaculture plots. This would contribute to establish the biogeochemical budget of the permaculture farm, to be compared to that of conventional horticulture gardening farms.
Author Contributions
J-TC designed the research plan. VS, FdT, and J-TC collected soil samples. VS and FdT performed the analyses. FdT, VS, CC, GC, and J-TC contributed to the analyses and the interpretation of the data. FdT prepared the discussion and figures. FdT wrote the first version of the manuscript, and all of the authors participated in editing this version.
Conflict of Interest Statement
The authors declare that the research was conducted in the absence of any commercial or financial relationships that could be construed as a potential conflict of interest.
Acknowledgments
We would like to thank Charles and Perrine Hervé-Gruyer and Louise Gehin for access to their farm and for their open-mindedness toward, among other things, this scientific study. We also thank all the people working at La Ferme du Bec Hellouin for letting us sample, and for the warm welcome made to Valentin. Finally, we want to thank Antoine Marquet from Assocation Bio Normandie for his time and the valuable information he imparted about organic gardening, and also François Fontaine for the XRD analysis, Aurore Degré for her help in implementing the aggregate separation procedure and Sebastien Ligot and Jean-Charles Bergen for their help in fractionating the soil aggregates. Finally, we also would like to thank ULiege for the Welcome Grant Research fund accorded to J-TC.
References
Abiven, S., Menasseri, S., and Chenu, C. (2009). The effects of organic inputs over time on soil aggregate stability–a literature analysis. Soil Biol. Biochem. 41, 1–12. doi: 10.1016/j.soilbio.2008.09.015
Altieri, M. A., and Nicholls, C. I. (2009). Agroecology, small farms, and food sovereignty. Sustain. Agric. Rev. 61, 102–113. doi: 10.1007/978-94-007-5449-2
Annabi, M., Le Bissonnais, Y., Le Villio-Poitrenaud, M., and Houot, S. (2011). Improvement of soil aggregate stability by repeated applications of organic amendments to a cultivated silty loam soil. Agric. Ecosyst. Environ. 144, 382–389. doi: 10.1016/j.agee.2011.07.005
Autret, B., Mary, B., Chenu, C., Balabane, M., Girardin, C., Bertrand, M., et al. (2016). Alternative arable cropping systems: a key to increase soil organic carbon storage? Results from a 16 year field experiment. Agric. Ecosyst. Environ. 232, 150–164. doi: 10.1016/j.agee.2016.07.008
Balesdent, J. (1996). The significance of organic seperates to carbon dynamics and its modelling in some cultivated soils. Eur. J. Soil Sci. 47, 485–493. doi: 10.1111/j.1365-2389.1996.tb01848.x
Balesdent, J., Besnard, E., Arrouays, D., and CHenu, C. (1998). The dynamics of carbon in particle size fractions of soil in a forest cultivated sequence. Plant Soil 201, 49–57. doi: 10.1023/A:1004337314970
Blake, G. R., and Hartge, K. H. (1986). “Bulk density,” in Methods of Soil Analysis, Part 1: Physical and Mineralogical Methods, ed A. Klute (Madison, WI: American Society of Agronomy), 365–375.
Campbell, C. A., Bowren, K. E., Schnitzer, M., Zentner, R. P., and Townley-Smith, L. (1991). Effect of crop rotations and fertilization on soil organic matter and some biochemical properties of a thick Black Chernozem. Can. J. Soil Sci. 387, 377–387. doi: 10.4141/cjss91-036
Cheng, X., Luo, Y., Xu, X., Sherry, R., and Zhang, Q. (2011). Soil organic matter dynamics in a North America tallgrass prairie after 9 yr of experimental warming. Biogeosciences 8, 1487–1498. doi: 10.5194/bg-8-1487-2011
Chenu, C., Angers, D. A., Barré, P., Derrien, D., and Arrouays, D. (2018). Increasing organic stocks in agricultural soils: knowledge gaps and potential innovations. Soil Tillage Res. doi: 10.1016/j.still.2018.04.011. [Epub ahead of print].
Coleman, E. (1995). The New Organic Grower: A Master's Manual of Tools and Techniques for the Home and Market Gardener, 2nd Edn. White River Junction, VT: Chelsea Green Publishing.
Denef, K., Six, J., Merckx, R., and Paustian, K. (2004). Carbon sequestration in microaggregates of no-tillage soils with different clay mineralogy. Soil Sci. Soc. Am. J. 68:1935. doi: 10.2136/sssaj2004.1935
Devine, S., Markewitz, D., Hendrix, P., and Coleman, D. (2014). Soil aggregates and associated organic matter under conventional tillage, no-tillage, and forest succession after three decades. PLoS ONE 9:e84988. doi: 10.1371/journal.pone.0084988
Diacono, M., and Montemurro, F. (2010). Long-term effects of organic amendments on soil fertility. A review. Agron. Sustain. Dev. 30, 401–422. doi: 10.1051/agro/2009040
Dignac, M.-F., Derrien, D., Barré, P., Barot, S., Cécillon, L., Chenu, C., et al. (2017). Increasing soil carbon storage: mechanisms, effects of agricultural practices and proxies. A review. Agron. Sustain. Dev. 37:14. doi: 10.1007/s13593-017-0421-2
Elliott, E. T. (1986). Aggregate structure and carbon, nitrogen, and phosphorus in native and cultivated soils. Soil Sci. Soc. Am. J. 50, 627–633. doi: 10.2136/sssaj1986.03615995005000030017x
Ferguson, R. S., and Lovell, S. T. (2014). Permaculture for agroecology: design, movement, practice, and worldview. A review. Agron. Sustain. Dev. 34, 251–274. doi: 10.1007/s13593-013-0181-6
Garcia-Franco, N., Albaladejo, J., Almagro, M., and Martínez-Mena, M. (2015). Beneficial effects of reduced tillage and green manure on soil aggregation and stabilization of organic carbon in a Mediterranean agroecosystem. Soil Tillage Res. 153, 66–75. doi: 10.1016/j.still.2015.05.010
Genot, V., Colinet, G., and Bock, L. (2007). La Fertilité des Sols en Région Wallonne–Dossier Scientifique Réalisé Dans le cadre de L'élaboration du Rapport Analytique 2006 sur l'Etat de l'Environnement Wallon, 1–77.
Guégan, S., and Leger, F. (2015). Maraîchage Biologique Permaculturel et Performance Économique. INRA.
Gulde, S., Chung, H., Amelung, W., Chang, C., and Six, J. (2008). Soil carbon saturation controls labile and stable carbon pool dynamics. Soil Sci. Soc. Am. J. 72, 605–612. doi: 10.2136/sssaj2007.0251
Hassink, J. (1997). The capacity of soils to preserve organic C and N by their association with silt and clay particles. Soil Sci. Soc. Am. J. 61, 131–139. doi: 10.2136/sssaj1997.03615995006100010020x
Hathaway, M. D. (2016). Agroecology and permaculture: addressing key ecological problems by rethinking and redesigning agricultural systems. J. Environ. Stud. Sci. 6, 239–250. doi: 10.1007/s13412-015-0254-8
Holmgren, D. (2002). Permaculture: Principles and Pathways Beyond Sustainability. Hepburn, VIC: Holmgren Design Services.
IUSS (2014). World Reference Base for Soil Classification 2014. Rome: Food Agriculture Organization of United Nations.
Jeavons, J. (2001). Biointensive mini-farming. J. Sustain. Agric. 19, 81–83. doi: 10.1300/J064v19n02_06
Jobbagy, E. G., and Jackson, R. B. (2001). The distribution of soil nutriments with depth: global patterns of the imprint of plants. Biogeochemistry 53, 51–77. doi: 10.1023/A:1010760720215
Kopittke, P. M., Dalal, R. C., Finn, D., and Menzies, N. W. (2017). Global changes in soil stocks of carbon, nitrogen, phosphorus, and sulphur as influenced by long-term agricultural production. Glob. Chang. Biol. 23, 2509–2519. doi: 10.1111/gcb.13513
Lakanen, E., and Erviö, R. (1971). A comparison of eight extractans for the determination of plant available micronutrients in soils. Acta Agral. Fenn. 123, 223–232.
Lehmann, J., and Kleber, M. (2015). The contentious nature of soil organic matter. Nature 528, 60–68. doi: 10.1038/nature16069
Lucas, Y. (2001). The role of plants in controlling rates and products of weathering: importance of biological pumping. Annu. Rev. Earth Planet. Sci. 29, 135–163. doi: 10.1146/annurev.earth.29.1.135
Mollison, B., and Holmgren, D. (1990). Permaculture One: A Perennial Agriculture for Human Settlements. Tagari.
Morel, K., San Cristobal, M., and Léger, F. G. (2017). Small can be beautiful for organic market gardens: an exploration of the economic viability of French microfarms using MERLIN. Agric. Syst. 158, 39–49. doi: 10.1016/j.agsy.2017.08.008
Murphy, B. W. (2015). Impact of soil organic matter on soil properties—a review with emphasis on Australian soils. Soil Res. 53, 605–635. doi: 10.1071/SR14246
Nicolás, C., Kennedy, J. N., Hernández, T., García, C., and Six, J. (2014). Soil aggregation in a semiarid soil amended with composted and non-composted sewage sludge-A field experiment. Geoderma 219–220, 24–31. doi: 10.1016/j.geoderma.2013.12.017
Oorts, K., Vanlauwe, B., and Merckx, R. (2003). Cation exchange capacities of soil organic matter fractions in a Ferric Lixisol with different organic matter inputs. Agric. Ecosyst. Environ. 100, 161–171. doi: 10.1016/S0167-8809(03)00190-7
Paradelo, R., van Oort, F., Barré, P., Billiou, D., and Chenu, C. (2016). Soil organic matter stabilization at the pluri-decadal scale: insight from bare fallow soils with contrasting physicochemical properties and macrostructures. Geoderma 275, 48–54. doi: 10.1016/j.geoderma.2016.04.009
Peltre, C., Christensen, B. T., Dragon, S., Icard, C., Kätterer, T., and Houot, S. (2012). RothC simulation of carbon accumulation in soil after repeated application of widely different organic amendments. Soil Biol. Biochem. 52, 49–60. doi: 10.1016/j.soilbio.2012.03.023
Poeplau, C., and Don, A. (2013). Sensitivity of soil organic carbon stocks and fractions to different land-use changes across Europe. Geoderma 192, 189–201. doi: 10.1016/j.geoderma.2012.08.003
Poulton, P., Johnston, J., MacDonald, A., White, R., and Powlson, D. (2018). Major limitations to achieving “4 per 1000” increases in soil organic carbon stock in temperate regions: evidence from long-term experiments at Rothamsted Research, U. K. Glob. Chang. Biol. 12, 3218–3221. doi: 10.1111/gcb.14066
Puget, P., Chenu, C., and Balesdent, J. (2000). Dynamics of soil organic matter associated with particle-size fractions of water-stable aggregates. Eur. J. Soil Sci. 51, 595–605. doi: 10.1111/j.1365-2389.2000.00353.x
Sarker, J. R., Singh, B. P., Dougherty, W. J., Fang, Y., Badgery, W., Hoyle, F. C., et al. (2018). Impact of agricultural management practices on the nutrient supply potential of soil organic matter under long-term farming systems. Soil Tillage Res. 175, 71–81. doi: 10.1016/j.still.2017.08.005
Six, J., Conant, R. T., Paul, E. A., and Paustian, K. (2002). Stabilization mechanisms of soil organic matter: implications for C-saturation of soils. Plant Soil 241, 155–176. doi: 10.1023/A:1016125726789
Six, J., Elliott, E. T., and Paustian, K. (2000). Soil macroaggregate turnover and microaggregate formation: a mechanism for C sequestration under no-tillage agriculture. Soil Biol. Biochem. 32, 2099–2103. doi: 10.1016/S0038-0717(00)00179-6
Six, J., Elliott, E. T., Paustian, K., and Doran, J. W. (1998). Aggregation and soil organic matter accumulation in cultivated and native grassland soils. Soil Sci. Soc. Am. J. 62, 1367–1377. doi: 10.2136/sssaj1998.03615995006200050032x
Solomon, D., Fritzsche, F., Lehmann, J., Tekalign, M., and Zech, W. (2002). Soil organic matter dynamics in the subhumid agroecosystems of the ethiopian highlands: evidence from natural 13 C abundance and particle-size fractionation. Soil Sci. Soc. Am. J. 66, 969–978. doi: 10.2136/sssaj2002.9690
Stoate, C., Báldi, A., Beja, P., Boatman, N. D., Herzon, I., van Doorn, A., et al. (2009). Ecological impacts of early 21st century agricultural change in Europe–a review. J. Environ. Manage. 91, 22–46. doi: 10.1016/j.jenvman.2009.07.005
Tejada, M., Hernandez, M. T., and Garcia, C. (2009). Soil restoration using composted plant residues: effects on soil properties. Soil Tillage Res. 102, 109–117. doi: 10.1016/j.still.2008.08.004
Tisdall, J. M., and Oades, J. M. (1982). Organic matter and water-stable aggregates in soils. Eur. J. Soil Sci. 33, 141–163. doi: 10.1111/j.1365-2389.1982.tb01755.x
USDA's Soil Survey Laboratory Staff (2017). Soil Survey Manual. USDA Handbook 18. Washinton, DC: Government Printing Office.
Keywords: agricultural transition, agroecology, soil fertility management, organic carbon stocks, soil organic matter management, aggregate-size fractions, soil carbon saturation, soil carbon stabilization
Citation: de Tombeur F, Sohy V, Chenu C, Colinet G and Cornelis J-T (2018) Effects of Permaculture Practices on Soil Physicochemical Properties and Organic Matter Distribution in Aggregates: A Case Study of the Bec-Hellouin Farm (France). Front. Environ. Sci. 6:116. doi: 10.3389/fenvs.2018.00116
Received: 27 May 2018; Accepted: 20 September 2018;
Published: 30 October 2018.
Edited by:
David Montagne, AgroParisTech Institut des Sciences et Industries du Vivant et de L'environnement, FranceReviewed by:
Jerome Balesdent, Institut National de la Recherche Agronomique (INRA), FranceCarsten W. Mueller, Technische Universität München, Germany
Copyright © 2018 de Tombeur, Sohy, Chenu, Colinet and Cornelis. This is an open-access article distributed under the terms of the Creative Commons Attribution License (CC BY). The use, distribution or reproduction in other forums is permitted, provided the original author(s) and the copyright owner(s) are credited and that the original publication in this journal is cited, in accordance with accepted academic practice. No use, distribution or reproduction is permitted which does not comply with these terms.
*Correspondence: Felix de Tombeur, ZmVsaXguZGV0b21iZXVyQHVsaWVnZS5iZQ==