The Toxicity of Silver Nanoparticles (AgNPs) to Three Freshwater Invertebrates With Different Life Strategies: Hydra vulgaris, Daphnia carinata, and Paratya australiensis
- 1Ecotoxicology Research Group, Aquatic Pollution Prevention Partnership, School of Science, RMIT University, Melbourne, VIC, Australia
- 2Centre for Environmental Sustainability and Remediation, School of Science, RMIT University, Melbourne, VIC, Australia
- 3Ian Potter NanoBioSensing Facility, NanoBiotechnology Research Laboratory, RMIT University, Melbourne, VIC, Australia
The toxicity of manufactured nanoparticles varies greatly depending on the test species in consideration and estimates of toxicity may also be confounded by test media in which the organisms are cultured. For a more comprehensive toxicity evaluation, species at different trophic levels or with life strategies, tested in different media should be included. In this study, we examined the toxicity of tyrosine-coated silver nanonparticles (tyr-AgNP) to three Australian freshwater invertebrates: Hydra vulgaris, Daphnia carinata, and Paratya australiensis. Tyr-AgNPs were synthesized, characterized and their behavior was examined in different media used for acute toxicity tests. Additionally, the sensitivity of tested organisms to tyr-AgNPs was compared to ionic silver (Ag+). Based on the LC50 values of both tyr-AgNPs and Ag+ ions at different time points, D. carinata was found to be the most sensitive species followed by P. australiensis and H. vulgaris. NP stability studies revealed that tyr-AgNPs were least stable in hydra medium followed by daphnid and shrimp media. This study demonstrates that significant differences in NP toxicity to aquatic organisms exist and the test media and the life strategy of the species play a key role in these differences. Therefore, it is recommended that a multispecies approach is used in predictive risk assessment of NPs and to ensure protection of native species from possible toxic effects from NPs released into aquatic systems. Also recommended is to carefully investigate the fate and behavior of NPs in different media in assessing NP toxicity and emphasize the need to use native species in developing relevant regulatory frameworks.
Introduction
Over the last 30 years, the number of products containing engineered nanomaterials increased across diverse fields mainly due to our growing capacity to synthesize and manipulate such materials (Nowack and Bucheli, 2007; Sun et al., 2017). Among the nanomaterials, silver nanoparticles (AgNPs) are considered as one of the most important due to its exceptional broad spectrum bactericidal properties (Durán et al., 2016; Kumar et al., 2018; Tian et al., 2018), relatively low cost of manufacturing AgNPs (Capek, 2004), unique properties and ability to form diverse nanostructures (Sohn et al., 2015). AgNPs are used in a growing number of applications across a diverse range of commercial consumer products such as food storage containers, coating materials, liquid fabric softeners and detergents, fabrics and clothing, sporting goods, cosmetics, wound dressings, toothbrushes, and antimicrobial coatings (Alarcon et al., 2015; Wei et al., 2015; McGillicuddy et al., 2017).
There are however concerns regarding the extensive application of NPs and rapid proliferation of NP-enabled products which may lead to a high release into the environment and cause deleterious effects on the organisms that are constantly exposed to these materials (Navarro et al., 2008a; Keller et al., 2013; León-Silva et al., 2016; McGillicuddy et al., 2017).
Tons of nanosilver are added into the aquatic environment annually from wastewater plants (Sohn et al., 2015), septic tanks (Benn and Westerhoff, 2008) and agricultural lands (Kaegi et al., 2011) with concentrations expected to range from 0.03 to 0.32 micrograms per liter (Batley et al., 2013; Keller et al., 2013; Keller and Lazareva, 2014). Despite the fact that most AgNPs tend to accumulate in the sludge solids in waste water treatment plants (WWTPs) (Wang et al., 2012), it is estimated that a significant proportion of AgNPs will be released in the effluent (Limbach et al., 2008; Gottschalk et al., 2009). The presence of AgNPs has already been detected in the treated effluent at concentrations of 12 ng L−1 (Siripattanakul-Ratpukdi and Fürhacker, 2014) and 0.1 mg L−1 (Mitrano et al., 2012).
The environmental impacts of AgNPs including their effects on different organisms within the aquatic environment are still largely unknown (Hu et al., 2016). The toxicity of NPs to organisms depend on the physicochemical characteristics of NPs (Fekete-Kertész et al., 2017). Organic coatings are widely investigated for NPs especially in biomedical research due to their green and ecofriendly characteristics (Daima et al., 2014; Park, 2014). Also, in aquatic systems, NPs undergo physical and chemical transformation (e.g., agglomeration, settling) and therefore, the organisms are not exposed solely to dissolved chemicals, for which test protocols in ecotoxicology were originally devised (Petersen et al., 2015). Therefore, it is necessary to characterize and assess the fate and behavior of NPs in the test medium (Hund-Rinke et al., 2016; Steinhäuser and Sayre, 2017; Rasmussen et al., 2018). The toxicity of Ag+ ions which are possibly released from AgNPs is a cause for concern since Ag+ ions have been traditionally considered as the most toxic form of silver in water prior to the interest in NPs (Ratte, 1999). Uncertainty on what causes their toxicity is one of the major issues when assessing effects of AgNPs (Yue et al., 2017). While the toxicity of AgNPs is partly explained by the release of Ag+ ions, the contribution of AgNPs remains unclear. Some studies presented evidence that toxicity is mainly due to the release of Ag+ ions (Li et al., 2015; Sakamoto et al., 2015; Shen et al., 2015), but others reported a specific nanoparticle effect that could not be simply explained by the dissolution of Ag+ ions (Fabrega et al., 2011; Sørensen et al., 2015). Interestingly, some recent studies have begun to point out that in certain cases metal nanoparticles may in fact be more toxic than the corresponding metal ions (Li et al., 2017; Pakrashi et al., 2017; Abramenko et al., 2018).
Complex and largely unknown properties of nanomaterials (Grieger et al., 2012) accompanied with lack of toxicological and exposure data (USEPA, 2015; Skjolding et al., 2016) are currently among the major barriers for robust risk assessment, which is otherwise critical to formulate sound policies around environmental regulation of nanomaterials (Hjorth et al., 2017). Knowledge gaps remain for many factors including dose-response relationships and differences across species to characterize the risk of NPs to aquatic organisms (Adam et al., 2015). Besides, there is no commensurate investment in environmental safety research while we invest heavily in the development of new nanotechnologies (Miller and Wickson, 2015). There are several known and unknown hurdles in evaluating the toxicity of NPs (Fabrega et al., 2011; Kumar et al., 2017) and the reported recommendation values for the protection of aquatic species in the literature vary greatly (USEPA, 1985, 2012; Fabrega et al., 2011; Gubbins et al., 2011; CCME, 2015; Kwak et al., 2016). Multispecies toxicity studies which include different trophic levels or species with different life strategies are scarce. Previous studies using more than one species showed that the toxicity of both Ag+ ions and AgNPs significantly differed based on the test species (Ribeiro et al., 2014; Kwak et al., 2016). Therefore, it is important to develop a species sensitivity distribution (SSD) which can be used to estimate the potentially affected fraction of species and to set up threshold concentrations (Garner et al., 2015; Belanger et al., 2017). Several studies have focused on deriving hazardous concentration values based on SSD (Gottschalk et al., 2013; Adam et al., 2015; Garner et al., 2015; Nam et al., 2015).
Kwak et al. (2016) and Chen et al. (2018) have studied SSDs of AgNPs and suggested hazardous concentration values (HC5) in the range of 1.1–31.73 μg L−1 at which 95% of species are not harmed in the aquatic environments. The objectives of the present study were to examine the toxicity of organic (tyrosine)-coated AgNPs (tyr-AgNP) and Ag+ ions to three freshwater invertebrates at different trophic levels: the cnidarian Hydra vulgaris, the daphnid Daphnia carinata, and the freshwater shrimp Paratya australiensis and to compare species sensitivity and the influence of culture media on the toxicity of AgNPs. Hydra (Medusozoa: Anthomedusae: Hydridae) is a freshwater coelenterate native to tropical and temperate regions. Their diploblastic body nature, recognizable morphological changes, ubiquitous presence in freshwater environments, easy maintenance in the laboratory, cost effectiveness, and the ability to reproduce asexually resulting in large number of genetically similar individuals make them an ideal organism for acute toxicity tests (Trottier et al., 1997; Beach and Pascoe, 1998). Daphnia sp. (Crustacea: Cladocera: Daphniidae) is widely used as a model organism in aquatic toxicology tests since they are one of the most sensitive organisms and their ecology, phylogeny, toxicology, and physiology are relatively well-understood and a great number of toxicants have been evaluated using daphnids (Von der Ohe and Liess, 2004; Altshuler et al., 2011; Edwards and Pascoe, 2018). The freshwater atyid shrimp, P. australiensis (Crustacea: Decapoda: Atyidae) inhabits streams and other freshwater habitats along the east coast of Australia (Hughes et al., 1995). There are no studies conducted to date on toxicity of NPs to P. australiensis though it has been used in toxicity studies as a bioindicator for other contaminants in the Australian environments (Phyu et al., 2005; Kumar et al., 2010a,b; Lanctôt et al., 2016).
Materials and Methods
Test Materials
Silver nitrate, L-tyrosine, and KOH were obtained from Sigma-Aldrich. Tyr-AgNPs were produced in-house as described previously by Daima et al. (2014). Briefly, 10 mL of 10−3 M of aqueous silver nitrate (AgNO3) solution, 10 mL of 10−3 M aqueous tyrosine solution and 1 mL of 10−1 M solution of KOH were mixed, diluted to 100 mL with deionized water and allowed to boil until the colorless solution turned yellow, indicating the formation of silver nanoparticles. The NPs were prepared in the required concentrations through boiling the aqueous dispersions, followed by dialysis using a dialysis membrane (MWCO: 12.4 kDa) to remove potentially unreduced Ag+ ions and other reactants. For dialysis of NPs, the membrane containing NPs was submerged in 3 L of MilliQ water with stirring over 48 h. The water was replaced twice with fresh MilliQ water after 6 and 24 h. The dialysed NP stock solution was kept in the dark. 0.1 mL of NP was acid digested in 0.2 mL of ultra-pure grade 70% HNO3 on a heating block at 105°C for 12 h. The digested sample was diluted with MilliQ water and the silver (Ag+) concentration in solution was measured with ICP-MS (7700X, Agilent Technologies).
Preparation and Characterization of Test Materials
The NPs were characterized in the MilliQ water as follows. The surface plasmon resonance (SPR) analysis of tyr-AgNPs was performed using a Varian Cary 50 UV-visible spectrophotometer in a quartz cuvette with a path length of 1 cm. NP size was determined by drop casting the NP solution on carbon-coated copper grids and images were obtained by transmission electron microscopy (TEM) operated at 100 kV (JEOL 1010). The zeta potential and hydrodynamic diameter (HDD) of the nanoparticles were measured using a folded capillary cell and glass cuvette, respectively, on a Zetasizer (Dynamic light scattering; Malvern Zetasizer Nano series, NanoZS). Nominal concentrations of test solutions for toxicity tests were prepared based on the ICP-MS results. Required amounts of tyr-AgNP stock solution and AgNO3 were pipetted into relevant test media to obtain the different exposure concentrations.
Test Organisms and Culture Conditions
The original animal stock of pink hydra (H. vulgaris) was obtained from Southern Biological Pty Ltd, Australia and cultured in 1 L glass bowls. The hydra culture medium (CaCl2.2H2O: 147 mg L−1, TES buffer: 110 mg L−1, EDTA: 4 mg L−1) was prepared based on the protocol described previously by Trottier et al. (1997). The pH was adjusted to 7.0 ± 0.1 with 1 M NaOH. The culture was kept at 25°C with a photoperiod of 16:8 h light: dark cycle. Animals were fed daily with live Artemia salina nauplii (brine shrimp) for 1 h. After feeding, the bowls were washed, and the medium was replaced. All glassware used were washed with 5% nitric acid and rinsed with de-ionized water prior to use.
D. carinata was selectively cultured and cultures were maintained at 20 ± 1°C with 16:8 h light: dark cycle to obtain neonatal cladocerans. ASTM standard medium which consists of 192 mg L−1 NaHCO3, 120 mg L−1 CaSO4.2H2O, 120 mg L−1 MgSO4, and 8 mg L−1 KCl (Barry and Meehan, 2000) was used as the culture media. Daphnids were fed with the alga Pseudokirchneriella subcapitata which was maintained as per the OECD guidelines (OECD, 2011). The medium was aerated to saturation with oxygen before addition of daphnids and renewed three times a week. pH was maintained at 7.5 ± 0.2 throughout and cultures were maintained in glass beakers, which were loosely covered to prevent any contamination and evaporation.
P. australiensis were collected using a sieve (4 mm mesh size) from the Yarra River at Warrandyte, Victoria, Australia (Latitude: 37° 43′ 31.9454″ S Longitude 145° 14′ 8.1744″ E). This is a pristine site in the natural reserve area (pH: 7.12; t°: 12°C and DO: 80 %). They were then transported to our laboratories in plastic containers with aeration provided. Shrimps were maintained in an aerated aquarium containing a mixture of river water and dechlorinated carbon filtered tap water (dilution water) which had a mean conductivity of 0.126 mS cm−1 and an ionic composition of Ca: 6.8 mg L−1, Mg: 2.1 mg L−1, K: 1.0 mg L−1, Na: 8.9 mg L−1, Cl: 15.0 mg L−1, SO4: 9.6 mg L−1, CO3 < 5 mg L−1, and HCO3: 23.0 mg L−1. The temperature was maintained at 15 ± 1°C and the light intensity was 800 lux at the surface of the water with a 16: 8 h light: dark cycle. The river water was replaced with one fifth of dilution water daily and at the end of the first week, they were kept in 100% dilution water. Shrimps were fed with Seramin® tropical flake food twice a day but were not fed for 24 h prior to and during toxicity testing. The animals were acclimated for 2 weeks before the start of toxicity tests.
Tyr-AgNP Temporal Stability and Dissolution in the Test Media
The stability of tyr-AgNPs in different media and MilliQ water was investigated as described by Tejamaya et al. (2012) with some modifications. NP solutions of 5 mg L−1 were incubated in glass vials for 96 h under similar conditions to the acute toxicity tests. The SPR, HDD, zeta potential, and pH of the suspensions were monitored at 0, 24, 48, and 96 h. Release of Ag+ from tyr-AgNPs in each medium was investigated as described by Xia et al. (2008) with some modifications. Briefly, NP suspensions were sampled by extracting 1 mL into Eppendorf tubes and centrifuging for 15 min at 21,000 g in a Sigma 3-KL centrifuge. The tubes were carefully removed, and 0.75 mL of the supernatant was transferred to 15 mL tubes. Samples were diluted with MilliQ water and acidified (2% HNO3) with HNO3 before ICP-MS (7700X, Agilent Technologies) analysis to measure Ag+. The percentages of soluble Ag to the nominal concentrations of AgNP suspensions were calculated (Zhao and Wang, 2012).
Hydra Acute Toxicity Test
Acute tests were conducted as per the methodology described by Trottier et al. (1997) in the hydra medium described above. The definitive test concentrations for all acute tests were selected based on the data obtained from range finding tests (data not shown). The toxicity of ionic silver was significantly higher than AgNPs and therefore, two different range of concentrations were used for toxicity tests. The exposure tests were conducted in 12 well plates in triplicate as each well contains 4 mL test solution. The tyr-AgNP concentrations tested were 10.8, 27, 54, 108, 216, 539.3, 1,078, and 2,157 μg L−1 plus a blank control (0 μg L−1). For each concentration, one Petri dish with the same test solution concentration were used as intermediate “transfer wells” to minimize dilution of test concentrations (Trottier et al., 1997). EDTA is a chelating agent involved in metal complexation which may affect the toxicity (Nugegoda and Rainbow, 1988; O'Brien et al., 1990; Smékalová et al., 2018). Therefore, the experiment was repeated for hydra for the same AgNP concentration range in the absence of EDTA in the medium. The tests were conducted in the dark with gentle agitation for a period of 96 h. Similarly, the concentrations tested for toxicity of Ag+ ions in the hydra medium in the presence and absence of EDTA were 0.94, 1.9, 3.8, 7.5, 15, 30, 50, and 100 μg L−1. At time 0, all the organisms were observed under the microscope at 6–10 times magnification to make sure all organisms were healthy. Any hydra with clubbed or shortened tentacles were replaced with healthy individuals with extended tentacles which were approximately as same length as the body column. The morphological changes in hydra were observed at 24, 48, 72, and 96 h and any hydra at a tulip stage were considered dead (Trottier et al., 1997).
Daphnid Acute Toxicity Test
Semi-static acute toxicity testing of tyr-AgNP to D. carinata was conducted based on OECD guidelines (OECD, 2004). ASTM standard medium was used and six concentrations (10, 20, 40, 60, 80, and 100 μg L−1) of tyr-AgNP plus a blank control (0 μg L−1) were used in quadruplicate. Toxicity of Ag+ ions to daphnids was assessed with the Ag+ concentrations of 0.2, 0.4, 0.6, 0.8, 1.0, and 1.2 μg L−1. Less than 24 h old daphnid neonates from the third brood progeny were used for experiments and each replicate had 6 neonates in a 40 mL glass vial containing 15 mL of test solution. The daphnids were not fed during the 48 h testing period. They were considered immobile if they could not move even after a gentle agitation and immobilization was recorded after 24 and 48 h. The toxicity tests were considered valid if the mortality was not >10% in the control and the DO concentration in all the test solutions exceeded 3 mg L−1.
Shrimp Acute Toxicity Test
Acute toxicity of tyr-AgNPs to P. australiensis was assessed by exposing them to 8 concentrations of tyr-AgNPs (5.4, 10.8, 21.6, 43.2, 75.5, 107.9, 539.3, and 1,078.7 μg L−1) plus a blank control (0 μg L−1). The shrimps (10–15 mm long) were exposed for 96 h in triplicate under static conditions with no replacement of the test solution. Each replicate had 10 animals in an aquarium containing 2 L of test solution and each aquarium was covered with a glass lid to reduce volatilization. The temperature was maintained at 15 ±1°C and a light intensity of 800 lux at the surface of the water with a 16: 8 light: dark cycle. Mortality was defined as lack of movement or response to gentle prodding (ASTM, 1998). Dead animals were removed every 12 h and the individual survival was assessed by counting the total number of dead animals at 24, 48, 72, and 96 h. pH, conductivity and dissolved oxygen (DO) were measured daily. The toxicity tests were considered valid if the mortality was not >10% in the control and the DO level in all the test solutions exceeded 60% saturation. Toxicity of Ag+ ions to shrimps was assessed with the Ag+ concentrations of 0.94, 1.9, 3.8, 7.5, 15, and 30 μg L−1.
Deriving the Species Sensitivity Distribution
For deriving the SSD, experimental toxicity data (LC50) of the present study and additional toxicity data retrieved from other published studies (amphibian, algae, bacteria, fish, nematodes, protozoa, and yeast) were used. Multiple toxicity data for the same species were summarized as geometric means. The SSD plots that show the proportion of species affected at different exposure levels in laboratory toxicity tests were developed by using the CADDIS SSD generator (Barron et al., 2013; Garner et al., 2015) that was downloaded from https://www.epa.gov/caddis-vol4/caddis-volume-4-data-analysis-download-software. The SSD toolbox that fits distributions to acute toxicity data was downloaded from https://www.epa.gov/endangered-species/provisional-models-endangered-species-pesticide-assessments.
Statistical Analysis
The median lethal concentrations (LC50) were obtained using the Trimmed Spearman-Karber method (Hamilton et al., 1977). This method is not subject to the problems of Probit and Logit models and has good statistical properties, is easy to use and recommended for accurate and precise calculations of LC50 values and their 95% confidence interval end points (Hamilton et al., 1977). All statistical analyses were based on a 0.05 significance level and are reported as the value with the range calculated as the standard deviation (±SD). Burr distribution and the maximum likelihood method were chosen for calculating the HC5 value based on the highest probability value generated from Chi square goodness of fit test. The HC5 was determined based on SSDs at a 95% protection level.
Results
Nanoparticle Characterization
The UV-visible absorbance spectra of the tyr-AgNPs (Figure 1A) shows an SPR band at 406 nm, which is characteristic of AgNPs, while the peak at ~272 nm is due to the tyrosine coating (Selvakannan et al., 2004; Daima et al., 2014). The presence of NPs was further confirmed by TEM images. Also, TEM images revealed that the NPs were spherical in shape and were reasonably uniform in size. The HDD was 86.8 nm (Figure 1B) while core size determined by TEM was ~30 nm in diameter (Figure 1C). The negative charge of the AgNPs, −42.4 mV (Figure 1D), as determined by zeta potential suggest that tyrosine molecules formed strong coatings on the AgNPs (Daima et al., 2014).
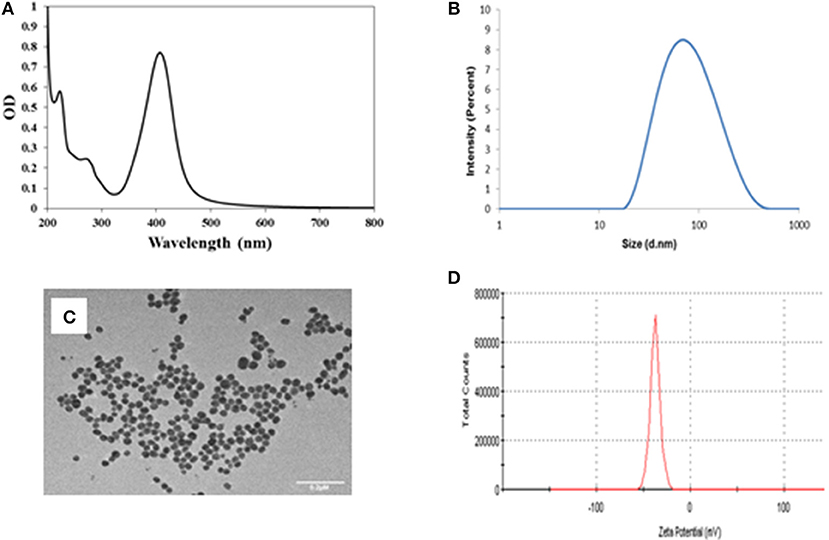
Figure 1. (A) UV visible spectra of the tyr-AgNPs with a peak at 406 nm, (B) SPR spectra of the tyr-AgNPs indicating hydrodynamic diameter of 86.82 nm, (C) TEM image of tyr-AgNPs indicates core size is ~30 nm. Scale bar represents 0.2 μm (D) Zeta potential of the tyr-AgNPs were −42.4 mV.
Tyr-AgNP Stability and Dissolution
Tyr-AgNP suspensions were yellowish in color initially with absorption peaks at 404, 402, and 406 nm in hydra, daphnid and shrimp media, respectively. Visual observation revealed that the color of NP suspension in hydra medium changed to gray within a few minutes (<10 min) and in daphnid medium changed to gray within a few hours (<3 h). The color in MilliQ water was stable even after 96 h. SPR (Figures 2A–D) revealed that the initial (<2 min) peak absorbance values in hydra (0.33) and daphnid media (0.50) were considerably lower than that in MilliQ water (0.77) and led to emergence of a second absorbance peak (broader) at higher wavelengths. The absorption peak in hydra medium completely disappeared within the first 24 h. There was a significant reduction of peak height in daphnid (53%) and shrimp (32%) media while in the case of MilliQ water only a marginal (~1%) decrease was observed. A further 12% decrease in the SPR peak was observed in all media and MilliQ water at 72 h. The HDD of tyr-AgNPs increased significantly in the hydra and daphnid media approximately by 3.5 and 6.5 times, respectively, in <5 min and approximately by 15 and 18 times, respectively, after 24 h (Table 1). The percentage intensity of different sizes (data not shown) in both media (weighted according to the scattering intensity of each particle fraction) revealed that the majority (90%) of aggregates remained in sub-micron level (100–1,000 nm) after 96 h. Less than 1.5 times size increase was observed in shrimp medium and MilliQ water at any time point. Percentage of aggregates at micrometer size in both shrimp medium and MilliQ water did not exceed 2.5% at any given time. Immersion of AgNPs in hydra, daphnid and shrimp media led to a considerable immediate decrease (34–67%) in zeta potential of NPs compared with the zeta potential of NPs in MilliQ water (Table 1). However, the decrease was 1–8% only in the next 3 days. In contrast, zeta potential changed only by 13% in MilliQ water at 4 d. Change of HDD and zeta potential of NPs in hydra and daphnid media and MilliQ water were in good agreement with changes observed in SPR spectra and color. Change of HDD of NPs in shrimp medium was in good agreement, but the significant decrease in zeta potential does not correlate with the other changes observed. Percentage dissolution of Ag ions after 96 h was 0.14, 0.26, and 0.13%, respectively, in hydra, daphnid, and shrimp media while it was 0.97% in the MilliQ water (Table 2).
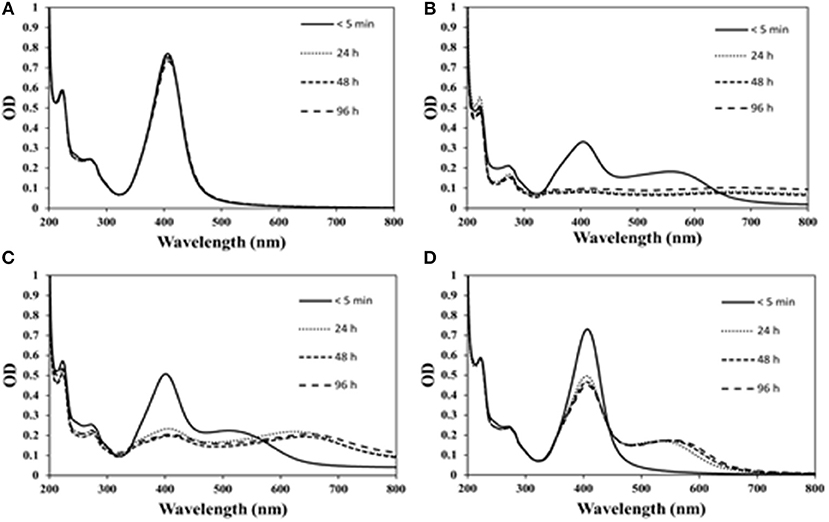
Figure 2. The effect of exposure time on the SPR of Tyr-AgNPs in (A) MilliQ water, (B) hydra, (C) daphnid, and (D) shrimp media.
Comparison of tyr-AgNPs and Ag+ Ion Toxicity to Hydra, Daphnids and Shrimps
Our data suggest that the acute toxicity of tyr-AgNPs and Ag+ ions to the three species tested is considerably different (Table 3). The results of our study indicated that the toxicity of Ag+ ions to all three test species was higher than the toxicity of the tyr-AgNPs. In the presence of EDTA, Ag+ ions were 25 times more toxic to hydra at all-time points. This was 27 times in the absence of EDTA at 24 h, but the ratio decreased to 10.5 at 48 h since the toxicity of AgNPs increased with time. Due to similar reason, the Ag+ ions were 90 times more toxic to daphnids at 24 h but decreased to 57 times at 48 h. Interestingly, the toxicity of Ag+ ions to shrimps increased from 17 times at 24 h to 553 times at 48 h compared with AgNPs due to the toxicity of Ag+ ions increased with time. tyr-AgNPs were 30 and 55 times more toxic to D. carinata than to hydra when 24 h and 48 h LC50 values are compared. However, this gap reduced to 11 and 7 times, respectively, when compared with the LC50 values for hydra in the medium without EDTA. In a similar pattern, Ag+ ions were 150 and 130 times toxic to D. carinata than to hydra when 24 and 48 h LC50 values are compared, but the gap reduced to 37 and 41 times when EDTA was absent in hydra medium. The toxicity of tyr-AgNPs to P. australiensis (LC50: 62.2 μg L−1) was similar to that of D. carinata (LC50: 62.0 μg L−1) in the first 24 h though they were more toxic to daphnids at 48 h (LC50: 35.4 μg L−1) compared to shrimps (LC50: 55.3 μg L−1). Interestingly, though Ag+ ions were 5 times more toxic to daphnids than shrimps at 24 h, they were 6 times more toxic to shrimps (LC50: 0.1 μg L−1) than to daphnids (LC50: 0.62 μg L−1) at 48 h. Overall, the toxicity of tyr-AgNPs and Ag+ ions to the three species was, respectively, in the order of daphnids > shrimps > hydra and shrimps > daphnids > hydra.
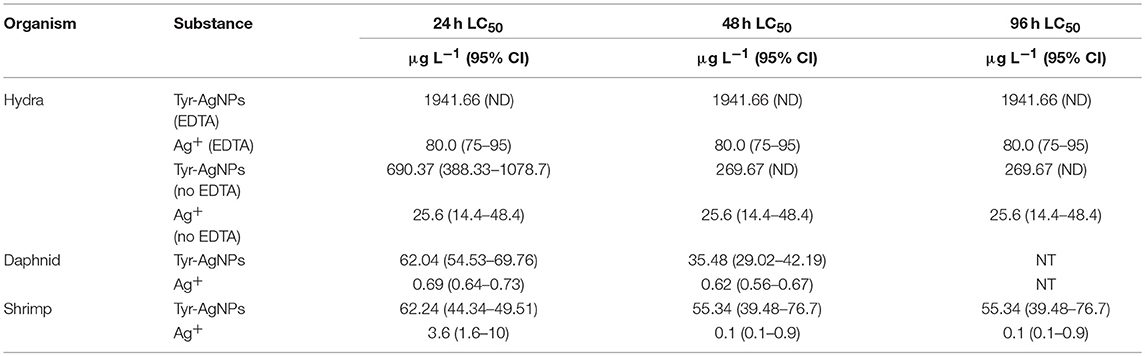
Table 3. Hydra vulgaris, Daphnia carinata and Paratya australiensis lethality (LC50) for tyr-AgNPs and Ag+ (± 95% confidence interval, n = 3) (CI, Confidence interval; ND, Not defined; NT, Not tested).
Effect of Media and Exposure Time on Toxicity of tyr-AgNPs
The 24, 48 and 96 h LC50 values of tyr-AgNPs to H. vulgaris were similar (1941.66 μg L−1 in the presence of EDTA and 690.37, 269.67, and 269.67 μg L−1 in the absence of EDTA. The 96 h LC50 values of Ag+ ions at each time point were similar in the presence (80.0 μg L−1) and absence (25.6 μg L−1) of EDTA. However, NP toxicity to D. carinata approximately doubled from 24 h (LC50: 62.0 μg L−1) to 48 h (LC50: 35.4 μg L−1) while there was no significant change observed for Ag+ ions toxicity from 24 h (LC50: 0.69 μg L−1) to 48 h (LC50: 0.62 μg L−1). Toxicity of NPs to P. australiensis only slightly increased from 24 h (LC50: 62.2 μg L−1) to 96 h (LC50: 55.3 μg L−1). Conversely, the toxicity of Ag+ ion increased by 36 times from 24 h (LC50: 3.6 μg L−1) to 96 h (LC50: 0.1 μg L−1). To summarize, a significant increase in toxicity of tyr-AgNPs and Ag+ ions with time was observed for daphnids and shrimps, respectively. Tyr-AgNPs were effectively toxic to hydra and shrimps within 24 h only and thereafter, no toxic responses were observed.
Hazardous Concentration (HC) of Coated AgNPs and Ag+ Ions
There are several methodologies which are employed by regulatory bodies in different countries to derive HC5 values and we chose the species sensitivity distribution (SSD) approach. Since we only had acute toxicity test results, the USEPA protocol was adopted since it supports using acute toxicity values (Kwak et al., 2016). To predict more accurate HC5 values, toxicity values of sufficient species should be available representing a comprehensive ecosystem. USEPA (1985) recommends having results of acceptable acute tests with 8 different families to derive the SSD for freshwater aquatic organisms. Since we had acute toxicity data of only three families, more data were sourced from published journal papers and the ETOX database (ETOX, 2018). SSDs are models of variance and the model fits of the probability distributions were excellent with R2-values of 0.98 and 0.93 for AgNO3 and AgNPs, respectively (Figures 3A,B). The HC5 value obtained for Ag+ ions and AgNPs in this study were 0.51 and 18.41 μg L−1.
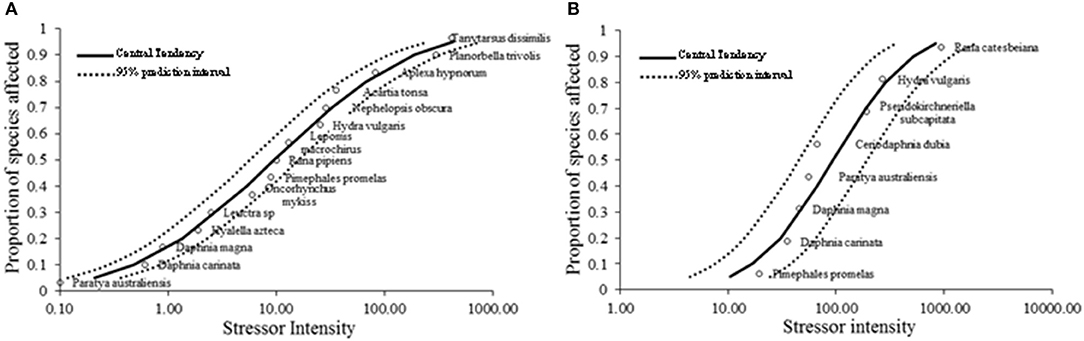
Figure 3. Species sensitivity distribution (SSD) derived by the CADDIS SSD generator and SSD toolbox. (A) SSD of AgNO3 acute toxicity, produced using fifteen species (P. australiensis, D. carinata, D. magna, H. Azteca, Leuctra sp., O. mykiss, P. promelas, R. pipiens, L. macrochirus, H. vulgaris, N. obscura, A. tonsa, A. hypnorum, P. trivolis, and T. dissimilis). (B) SSD of AgNP acute toxicity, produced using eight species (P. promelas, D. carinata, D. magna, P. australiensis, C. dubia, R. subcapitata, H. vulgaris, and R. catesbeiana).
Discussion
Bondarenko et al. (2013) summarized LC50 values of AgNPs (48 h LC50: 1.0–40.0 μg L−1) from 17 previous studies with a median value of 10 μg L−1 and LC50 values of Ag+ ions (48 h LC50: 1.0–9.40 μg L−1) from 8 studies with a median value of 0.85 μg L−1. The authors could find only one study where 24 h LC50 (3.5–10.8 μg L−1) and 48 h LC50 (1.75–4.61 μg L−1) values of AgNPs using D. carinata were reported (Qin et al., 2015). These researchers exposed daphnids in two different media to AgNPs synthesized with the fungus Gibberella sp. and the HDD of the NPs were between 20 and 50 nm. The 48 h LC50 values obtained for D. carinata in our study (35.4 μg L−1) is in general agreement with LC50 values reported by others for Daphnia sp. though it is considerably less sensitive to tyr-AgNPs in comparison with the values obtained by Qin et al. (2015) for D. carinata. This may be due to different physichochemical characteristics of AgNPs tested and different chemical characteristics of the media used. This is the first study which used P. australiensis to assess NP toxicity. Similar toxicity of AgNPs to both shrimps and daphnids at 24 h, but higher toxicity to daphnids at 48 h compared with shrimps may be due to their different food habits. Daphnids being filter feeders take up larger volumes of the aqueous medium by habit as opposed to shrimps which are detritus feeders and utilize water only for respiration. Higher toxicity of Ag+ ions to daphnids at 24 h, but less toxicity at 48 h compared with shrimps may be a result of a change in the interaction of Ag+ ions with other trace elements in the daphnid medium over time changing the bioavailability of Ag+ ions (Wasmuth et al., 2016). Also, higher toxicity of AgNPs to daphnids at 48 h in contrast to shrimps which were more sensitive to Ag+ at 48 h is attributed to different mechanisms of toxicity on two organisms. Though hydra is a simple diploblastic organism with two simple cell layers in their body, the entry of the tested toxicants across their ectodermal cells may be less than across the gills of daphnids and shrimps, resulting in the lower toxicity of tyr-AgNP and Ag+ ions to hydra. Hydra is a predator and hence does not uptake the aquatic medium as much as daphnids that are filter feeders resulting in less exposure to the toxicants as a result.
Fate and behavior of NPs in the media affect bioactivity and bioavailability. Achieving a constant concentration of AgNPs in the media or actual measurement is challenging. However, monitoring certain attributes such as particle agglomeration size, SPR, and dissolution can favor understanding of observed toxicity. Appearance of additional and broader absorbance peaks, rapid absorbance loss, increased particle size and color change are indicative of formation of aggregates (Römer et al., 2011; Stebounova et al., 2011; Tejamaya et al., 2012). Results indicate that NPs highly aggregated in the hydra and daphnid media while it was marginal in shrimp medium. No aggregation was observed in MilliQ water. Decreased zeta potential values in the media with high ionic strength compared to MilliQ water with relatively low ionic strength are in accordance with the classical colloid theory (Hunter, 1981; Elimelech et al., 1995). A reduction of the thickness of the diffuse double layer with increased ionic strength allows for the attractive van der Waals interactions to dominate and increases the particle-particle interaction resulting in increased aggregation (Brant et al., 2005; Sharma, 2009; El Badawy et al., 2010). Aggregation of NPs affect the bioavailable concentration of NPs (Römer et al., 2011; Tejamaya et al., 2012) and hence, inter alia plays an important role in potential toxicity (Reidy et al., 2013; Völker et al., 2013; Park et al., 2015; Carnovale et al., 2016). Smaller particles can penetrate in to the body more easily (Smékalová et al., 2018) and release more Ag+ ions due to higher surface area than larger particles (Allen et al., 2010; Zhao and Wang, 2012). The observed insignificant ion release from AgNPs in the media in this study may also be due to the aggregation. SPR and HDD data from our study show that tyr-AgNPs aggregate fast in the hydra medium, forming a bigger fraction (85%) of aggregates with HDD being larger than 650 nm. This leads to deposition of particles making them less bioavailable in a short period of time. Daphnids and shrimps can eat precipitated NPs on the bottom, increasing their respective exposure rates, but hydras are not able to do that. Another reason for lower toxicity from tyr-AgNPs and Ag+ to hydra is the less bioavailability of NPs in the medium due to aggregation and precipitation.
The composition and the pH of the media affect the ecotoxicological potential of NPs (Seitz et al., 2015; Metreveli et al., 2016). The hydra medium Trottier et al. (1997) used contains EDTA. The hydra toxicity test was extended to see the effect of EDTA on toxicity by exposing hydra to both tyr-AgNPs and Ag+ ions in the absence of EDTA. The LC50 values at each time point for both AgNPs and Ag+ ions in the absence of EDTA are less than the corresponding LC50 values in the media with EDTA. EDTA chelates metals which may cause reduced toxicity due to decreased rate of metal uptake (O'Brien et al., 1990). Therefore, lower toxicity of both tyr-AgNPs and Ag+ ions in the EDTA containing hydra medium is mainly attributed to the complexation of Ag+ ions by EDTA. Also, hydra in the medium with EDTA are more robust than in the medium without EDTA since the former is the recommended recipe for optimum growth of hydra (Trottier et al., 1997). Some of the ions released are trapped by the halides (e.g., Cl−1) present in the media making insoluble precipitations (Römer et al., 2011) resulting in less detectable free ions. Precipitation of Ag+ ions as AgCl by high concentration of Cl−1 in the hydra medium is also a possible factor for observed less toxicity of AgNPs and Ag+ ions to hydra. AgNPs oxidize more easily with increasing pH resulting in higher dissolution (Liu and Hurt, 2010; Zhao and Wang, 2012). Higher pH of the media compared with MilliQ (pH: 6.44) water is a possible factor for observed lower dissolution of Ag+ ions in media.
It is well-understood that soluble silver compounds are highly toxic to aquatic organisms and best explained by the Biotic Ligand Model (BLM) (Bianchini and Wood, 2003). However, the mechanisms of how AgNPs are toxic to organisms are still in debate and there are divergent opinions on the effects (Völker et al., 2013). Several researchers have shown that the dissolved ions from the NPs is the dominant cause for acute toxicity (Li et al., 2015; Sakamoto et al., 2015; Shen et al., 2015) while some have claimed that the toxicity is attributed not only to the liberated ions from AgNPs, but also to the NPs themselves (Navarro et al., 2008b; Lapresta-Fernández et al., 2012; Davoudi et al., 2014). Corresponding Ag+ ion concentrations at LC50 values of tyr-AgNPs, calculated based on percentage dissolution are lower than the LC50 values of Ag+ ions. This indicates that there is a synergistic effect from both tyr-AgNPs and Ag+ ions in causing toxicity. Certain aquatic organisms such as algae alter the toxicity of NPs by releasing exopolymeric substances (Zhou et al., 2016) which is not expected with the tested organisms in the current study. However, reduced Ag concentrations in the media due to sorption of NPs to the organisms or excreta cannot be excluded (Bone et al., 2012).
The HC5 value we found (0.51 μg L−1) for ionic silver based on the acute toxicity values in this study and 12 other freshwaters species is about 6 times lower than the USEPA Criterion Maximum Concentration (CMC) value (3.2 μg L−1) (USEPA, 1985). However, it is 2 and 10 times higher than the recommended long-term freshwater Canadian water quality guideline (CWQG) for ionic silver (0.25 μg L−1) (CCME, 2015) and the Australia and New Zealand's water quality guideline (ANZWQG) for ionic silver (0.05 μg L−1) (ANZECC, 2000), respectively. Relatively higher ionic silver guideline values by USEPA is likely because CMC values reflect only acute effects in contrast to lower values by CWQG and ANZWQG which use chronic toxicity data (Kwak et al., 2016). The HC5 value (0.51 μg L−1) for ionic silver from this study is lower than the reported values by Garner et al. (2015) (~1.0 μg L−1) and Chen et al. (2018) (3.6 and 5.7 μg L−1). Ionic silver is significantly toxic compared to the HC5 values reported for other metallic ions (10–4,000 μg L−1) (Garner et al., 2015; Chen et al., 2018). The HC5 value derived in this study for ionic silver is 36 times lower than the HC5 value obtained for AgNPs (18.41 μg L−1). The HC5 value derived in this study for AgNPs is less than the HC5 value reported by Kwak et al. (2016) for PVP coated AgNPs (31.73 μg L−1). However, Chen et al. (2018) reported comparatively lower HC5 values for PVP coated (1.1 μg L−1), sodium citrate coated (3.0 μg L−1), and uncoated (6.3 μg L−1) AgNPs. The reported HC5 values for other metallic NPs fall in the range of 22–4,000 μg L−1.
There is a growing interest in using SSDs to derive hazard levels of contaminants. SSD approach has significant influence on assessment and decision making of chemical exposure to ecosystems (Belanger et al., 2017). The risk assessment approaches of NPs have not been tested and validated yet (Mattsson and Simkó, 2017). There are many reasons for the uncertainty in measuring the hazard of AgNPs such as broad range of parameters of NPs, indirect effects, lack of information, modifications of NPs in the environment, questionable validity and methods of test systems (Mattsson and Simkó, 2017). Several researchers highlighted the importance of SSDs in deriving HCs to protect native fauna from NPs (Adam et al., 2015; Garner et al., 2015; Kwak et al., 2016). Traditionally, SSDs were derived using the toxicity values based on the effects of the contaminant on organisms at different hierarchies. Experiments with several species with the same batch of each nanoparticle is challenging and therefore, it is not possible to safeguard the biota by concentrating on specific nanoparticles. In line with this, several researchers (Adam et al., 2015; Garner et al., 2015) have sourced toxicity values from literature to derive SSDs. Further, the accuracy of HCs predicted will increase when more data are available (Garner et al., 2015). Since this is the only study with tyrosine coated AgNPs, we sourced the toxicity values of AgNPs from published literature. However, this will bring some uncertainty due to several variabilities such as different exposure conditions, physichochemical characteristics of NPs, which has caused to question the applicability of using SSDs for deriving HCs for NPs (Adam et al., 2015; Garner et al., 2015). Better estimates can be obtained by developing SSDs based on grouping of NPs (Gottschalk and Nowack, 2013; Garner et al., 2015). However, Chen et al. (2018) did not observe a significant difference in the derived HC5 values for AgNPs based on the size, shape and exposure duration. This similarity was attributed to the transformation of particles in the medium and the biological effects are likely to result from the release of ions.
Also, chronic sublethal effects have enormous sublethal effects on organisms (Garner et al., 2015) and thus, chronic test results should be used in developing SSDs. However, studies on chronic end points are scarce in comparison to the acute test results which leads to a bias toward SSDs based on acute test data. Therefore, more useful chronic tests are recommended to generate SSDs. Besides, as observed in this study, it is acknowledged the difficulty in maintaining constant NP concentrations in the test media for such experiments.
This study shows the different sensitivity to tyrosine coated AgNPs across species using Australian native species and highlights the importance of considering species with different life habits in assessing toxicity of NPs. There is a considerable difference in fate and behavior of tyr-AgNPs in different media and quantification of such changes is important when performing and interpreting toxicity assessments. Also, it appreciates the importance of SSD in evaluating HC levels of differently coated NPs and adds new knowledge which will be important in AgNP risk assessment (Posthuma et al., 2001) and setting up water quality guidelines (Smetanová et al., 2014). Based on the results, the authors recommend further NP exposure studies for differently coated NPs using a broad range of freshwater aquatic species with different life habits to assess the toxicity of the range of novel manufactured NPs.
Conclusions
Freshwater aquatic organisms are potentially exposed to AgNPs and Ag+ ions. The objectives of the present study were to examine the toxicity of tyrosine-coated AgNPs (tyr-AgNPs) and Ag+ ions to three freshwater invertebrates at different trophic levels, to compare species sensitivity and the influence of culture media on the toxicity of AgNPs. The toxicity of tyr-AgNPs and Ag+ ions to the three species was, respectively, in the order of daphnids > shrimps > hydra and shrimps > daphnids > hydra. Fate and behavior of AgNPs and Ag+ ions were dependent on the test media and should be considered a key factor in assessing NP toxicity. To protect aquatic organisms from AgNP exposures, it is necessary to set water quality guidelines based on data gathered from toxicity studies relevant to different species in such environments. Assessing the sensitivity of species with different life habits and native species to AgNPs and Ag+ ions are highlighted.
Author Contributions
SL and AM participated in designing the study, performed the experiments, interpreted the data, wrote the manuscript, and revised it until its final version. VL synthesized the nanoparticles. AA characterized the nanoparticles and involved in manuscript writing. RS, VB, and DN planned the study, participated in designing the study, made significant contributions in interpreting data, and edited the manuscript. VB was also involved in coordination of nanoparticle production and characterization. All of the authors examined and agreed with the final version of the manuscript.
Funding
This material is based upon work which was supported by the Australian Government through an Australian Government Research Training program Scholarship to SL and VB acknowledges the Australian Research Council for a Future Fellowship and funding support through FT140101285. Any opinions, findings, and conclusions or recommendations expressed in this material are those of the authors and do not necessarily reflect the views of the Australian Government.
Conflict of Interest Statement
The authors declare that the research was conducted in the absence of any commercial or financial relationships that could be construed as a potential conflict of interest.
Acknowledgments
We acknowledge the support from the RMIT Microscopy and Microanalysis Facility (RMMF) for technical assistance and providing access to characterization facilities. VB also thanks the Ian Potter Foundation for supporting the establishment of an Ian Potter NanoBioSensing Facility at RMIT University.
References
Abramenko, N. B., Demidova, T. B., Abkhalimov, E. V., Ershov, B. G., Krysanov, E. Y., and Kustov, L. M. (2018). Ecotoxicity of different-shaped silver nanoparticles: case of zebrafish embryos. J. Hazard. Mater. 347, 89–94. doi: 10.1016/j.jhazmat.2017.12.060
Adam, N., Schmitt, C., De Bruyn, L., Knapen, D., and Blust, R. (2015). Aquatic acute species sensitivity distributions of ZnO and CuO nanoparticles. Sci. Total Environ. 526, 233–242. doi: 10.1016/j.scitotenv.2015.04.064
Alarcon, E. I., Griffith, M., and Udekwu, K. I. (2015). Silver Nanoparticle Applications. Springer International Publishing. doi: 10.1007/978-3-319-11262-6
Allen, H. J., Impellitteri, C. A., Macke, D. A., Heckman, J. L., Poynton, H. C., Lazorchak, J. M., et al. (2010). Effects from filtration, capping agents, and presence/absence of food on the toxicity of silver nanoparticles to Daphnia magna. Environ. Toxicol. Chem. 29, 2742–2750. doi: 10.1002/etc.329
Altshuler, I., Demiri, B., Xu, S., Constantin, A., Yan, N. D., and Cristescu, M. E. (2011). An integrated multi-disciplinary approach for studying multiple stressors in freshwater ecosystems: daphnia as a model organism. Integr. Comp. Biol. 51, 623–33. doi: 10.1093/icb/icr103
ANZECC (2000). Australian and New Zealand Guidelines for Fresh and Marine Water Quality. Canberra, ACT: Agriculture and Resource Management Council of Australia and New Zealand.
ASTM (1998). Standard guide for conducting acute toxicity tests on aqueouseffluents with fishes, macroinvertebrates, and amphibians. Am. Soc. Test. Mater. 11.06, E1192–E1197. doi: 10.1520/E1192-97R14
Barron, M. G., Hemmer, M. J., and Jackson, C. R. (2013). Development of aquatic toxicity benchmarks for oil products using species sensitivity distributions. Integr. Environ. Assess. Manag. 9, 610–615. doi: 10.1002/ieam.1420
Barry, M. J., and Meehan, B. J. (2000). The acute and chronic toxicity of lanthanum to Daphnia carinata. Chemosphere 41, 1669–1674. doi: 10.1016/S0045-6535(00)00091-6
Batley, G. E., Kirby, J. K., and Mclaughlin, M. J. (2013). Fate and risks of nanomaterials in aquatic and terrestrial environments. Acc. Chem. Res. 46, 854–862. doi: 10.1021/ar2003368
Beach, M. J., and Pascoe, D. (1998). The role of Hydra vulgaris (Pallas) in assessing the toxicity of freshwater pollutants. Water Res. 32, 101–106. doi: 10.1016/S0043-1354(97)00180-2
Belanger, S., Barron, M., Craig, P., Dyer, S., Galay-Burgos, M., Hamer, M., et al. (2017). Future needs and recommendations in the development of species sensitivity distributions: estimating toxicity thresholds for aquatic ecological communities and assessing impacts of chemical exposures. Integr. Environ. Assess. Manag. 13, 664–674. doi: 10.1002/ieam.1841
Benn, T. M., and Westerhoff, P. (2008). Nanoparticle silver released into water from commercially available sock fabrics. Environ. Sci. Technol. 42, 4133–4139. doi: 10.1021/es7032718
Bianchini, A., and Wood, C. M. (2003). Mechanism of acute silver toxicity in Daphnia magna. Environ. Toxicol. Chem. 22, 1361–1367. doi: 10.1897/1551-5028(2003)022<1361:MOASTI>2.0.CO;2
Bondarenko, O., Juganson, K., Ivask, A., Kasemets, K., Mortimer, M., and Kahru, A. (2013). Toxicity of Ag, CuO and ZnO nanoparticles to selected environmentally relevant test organisms and mammalian cells in vitro: a critical review. Arch. Toxicol. 87, 1181–1200. doi: 10.1007/s00204-013-1079-4
Bone, A. J., Colman, B. P., Gondikas, A. P., Newton, K. M., Harrold, K. H., Cory, R. M., et al. (2012). Biotic and abiotic interactions in aquatic microcosms determine fate and toxicity of Ag nanoparticles: part 2–toxicity and Ag speciation. Environ. Sci. Technol. 46, 6925–6933. doi: 10.1021/es204683m
Brant, J., Lecoanet, H., and Wiesner, M. R. (2005). Aggregation and deposition characteristics of fullerene nanoparticles in aqueous systems. J. Nanoparticle Res. 7, 545–553. doi: 10.1007/s11051-005-4884-8
Capek, I. (2004). Preparation of metal nanoparticles in water-in-oil (w/o) microemulsions. Adv. Colloid Interface Sci. 110, 49–74. doi: 10.1016/j.cis.2004.02.003
Carnovale, C., Bryant, G., Shukla, R., and Bansal, V. (2016). Size, shape and surface chemistry of nano-gold dictate its cellular interactions, uptake and toxicity. Prog. Mater. Sci. 83, 152–190. doi: 10.1016/j.pmatsci.2016.04.003
CCME (2015). Scientific Criteria Document for the Development of the Canadian Water Quality Guidelines for the Protection of Aquatic Life: Silver Winnipeg. Winnipeg, MB: Canadian Council of Ministers of the Environment.
Chen, G., Peijnenburg, W. J. G. M., Xiao, Y., and Vijver, M. G. (2018). Developing species sensitivity distributions for metallic nanomaterials considering the characteristics of nanomaterials, experimental conditions, and different types of endpoints. Food Chem. Toxicol. 112, 563–570. doi: 10.1016/j.fct.2017.04.003
Daima, H. K., Selvakannan, P., Kandjani, A. E., Shukla, R., Bhargava, S. K., and Bansal, V. (2014). Synergistic influence of polyoxometalate surface corona towards enhancing the antibacterial performance of tyrosine-capped Ag nanoparticles. Nanoscale 6, 758–765. doi: 10.1039/C3NR03806H
Davoudi, Z. M., Kandjani, A. E., Bhatt, A. I., Kyratzis, I. L., O'mullane, A. P., and Bansal, V. (2014). Hybrid antibacterial fabrics with extremely high aspect ratio Ag/AgTCNQ nanowires. Adv. Funct. Mater. 24, 1047–1053. doi: 10.1002/adfm.201302368
Durán, N., Durán, M., De Jesus, M. B., Seabra, A. B., Fávaro, W. J., and Nakazato, G. (2016). Silver nanoparticles: a new view on mechanistic aspects on antimicrobial activity. Nanomed. Nanotechnol. Biol. Med. 12, 789–799. doi: 10.1016/j.nano.2015.11.016
Edwards, R., and Pascoe, D. (2018). Single Species Toxicity Tests. Aquatic Ecotoxicology. Boca Raton, FL: CRC Press.
El Badawy, A. M., Luxton, T. P., Silva, R. G., Scheckel, K. G., Suidan, M. T., and Tolaymat, T. M. (2010). Impact of environmental conditions (pH, ionic strength, and electrolyte type) on the surface charge and aggregation of silver nanoparticles suspensions. Environ. Sci. Technol. 44, 1260–1266. doi: 10.1021/es902240k
Elimelech, M., Gregory, J., Jia, X., and Williams, R. A. (1995). Particle Deposition and Aggregation: Measurement, Modeling and Simulation. Oxford: Butterworth-Heinemann.
ETOX (2018). ETOX: Information System Ecotoxicology and Environmental Quality Targets [Online]. Available online at: https://webetox.uba.de/webETOX/index.do (Accessed September 30, 2018).
Fabrega, J., Luoma, S. N., Tyler, C. R., Galloway, T. S., and Lead, J. R. (2011). Silver nanoparticles: behaviour and effects in the aquatic environment. Environ. Int. 37, 517–531. doi: 10.1016/j.envint.2010.10.012
Fekete-Kertész, I., Piszmán, D., and Molnár, M. (2017). Particle size and concentration dependent ecotoxicity of nano- and microscale TiO2—comparative study by different aquatic test organisms of different trophic levels. Water Air Soil Pollut. 228:245. doi: 10.1007/s11270-017-3394-5
Garner, K. L., Suh, S., Lenihan, H. S., and Keller, A. A. (2015). Species sensitivity distributions for engineered nanomaterials. Environ. Sci. Technol. 49, 5753–5759. doi: 10.1021/acs.est.5b00081
Gottschalk, F., Kost, E., and Nowack, B. (2013). Engineered nanomaterials in water and soils: a risk quantification based on probabilistic exposure and effect modeling. Environ. Toxicol. Chem. 32, 1278–1287. doi: 10.1002/etc.2177
Gottschalk, F., and Nowack, B. (2013). A probabilistic method for species sensitivity distributions taking into account the inherent uncertainty and variability of effects to estimate environmental risk. Integr. Environ. Assess. Manag. 9, 79–86. doi: 10.1002/ieam.1334
Gottschalk, F., Sonderer, T., Scholz, R. W., and Nowack, B. (2009). Modeled environmental concentrations of engineered nanomaterials (TiO2, ZnO, Ag, CNT, Fullerenes) for different regions. Environ. Sci. Technol. 43, 9216–9222. doi: 10.1021/es9015553
Grieger, K. D., Linkov, I., Hansen, S. F., and Baun, A. (2012). Environmental risk analysis for nanomaterials: review and evaluation of frameworks. Nanotoxicology 6, 196–212. doi: 10.3109/17435390.2011.569095
Gubbins, E. J., Batty, L. C., and Lead, J. R. (2011). Phytotoxicity of silver nanoparticles to Lemna minor L. Environ. Pollut. 159, 1551–1559. doi: 10.1016/j.envpol.2011.03.002
Hamilton, M. A., Russo, R. C., and Thurston, R. V. (1977). Trimmed Spearman-Karber method for estimating median lethal concentrations in toxicity bioassays. Environ. Sci. Technol. 11, 714–719. doi: 10.1021/es60130a004
Hjorth, R., Skjolding, L. M., Sørensen, S. N., and Baun, A. (2017). Regulatory adequacy of aquatic ecotoxicity testing of nanomaterials. NanoImpact 8, 28–37. doi: 10.1016/j.impact.2017.07.003
Hu, X., Li, D., Gao, Y., Mu, L., and Zhou, Q. (2016). Knowledge gaps between nanotoxicological research and nanomaterial safety. Environ. Int. 94, 8–23. doi: 10.1016/j.envint.2016.05.001
Hughes, J. M., Bunn, S. E., Kingston, D. M., and Hurwood, D. A. (1995). Genetic differentiation and dispersal among populations of Paratya australiensis (Atyidae) in rainforest streams in Southeast Queensland, Australia. J. North Am. Benthol. Soc. 14, 158–173. doi: 10.2307/1467731
Hund-Rinke, K., Baun, A., Cupi, D., Fernandes, T. F., Handy, R., Kinross, J. H., et al. (2016). Regulatory ecotoxicity testing of nanomaterials–proposed modifications of OECD test guidelines based on laboratory experience with silver and titanium dioxide nanoparticles. Nanotoxicology 10, 1442–1447. doi: 10.1080/17435390.2016.1229517
Hunter, R. J. (1981). Zeta Potential in Colloid Science: Principles and Applications. London, Academic Press.
Kaegi, R., Voegelin, A., Sinnet, B., Zuleeg, S., Hagendorfer, H., Burkhardt, M., et al. (2011). Behavior of metallic silver nanoparticles in a pilot wastewater treatment plant. Environ. Sci. Technol. 45, 3902–3908. doi: 10.1021/es1041892
Keller, A. A., and Lazareva, A. (2014). Predicted releases of engineered nanomaterials: from global to regional to local. Environ. Sci. Technol. Lett. 1, 65–70. doi: 10.1021/ez400106t
Keller, A. A., Mcferran, S., Lazareva, A., and Suh, S. (2013). Global life cycle releases of engineered nanomaterials. J. Nanoparticle Res. 15, 1–17. doi: 10.1007/s11051-013-1692-4
Kumar, A., Correll, R., Grocke, S., and Bajet, C. (2010a). Toxicity of selected pesticides to freshwater shrimp, Paratya australiensis (Decapoda: Atyidae): use of time series acute toxicity data to predict chronic lethality. Ecotoxicol. Environ. Saf. 73, 360–369. doi: 10.1016/j.ecoenv.2009.09.001
Kumar, A., Doan, H., Barnes, M., Chapman, J., and Kookana, R. (2010b). Response and recovery of acetylcholinesterase activity in freshwater shrimp, Paratya australiensis (Decapoda: Atyidae) exposed to selected anti-cholinesterase insecticides. Ecotoxicol. Environ. Saf. 73, 1503–1510. doi: 10.1016/j.ecoenv.2010.07.016
Kumar, A., Singh, S., Shanker, R., and Dhawan, A. (2017). Nanotoxicology: Challenges for Biologists. Cambridge: Royal Society of Chemistry.
Kumar, S. S. D., Houreld, N. N., Kroukamp, E. M., and Abrahamse, H. (2018). Cellular imaging and bactericidal mechanism of green-synthesized silver nanoparticles against human pathogenic bacteria. J. Photochem. Photobiol. B. Biol. 178, 259–269. doi: 10.1016/j.jphotobiol.2017.11.001
Kwak, J. I., Cui, R., Nam, S. H., Kim, S. W., Chae, Y., and An, Y. J. (2016). Multispecies toxicity test for silver nanoparticles to derive hazardous concentration based on species sensitivity distribution for the protection of aquatic ecosystems. Nanotoxicology 10, 521–530. doi: 10.3109/17435390.2015.1090028
Lanctôt, C., Wilson, S., Fabbro, L., Leusch, F., and Melvin, S. (2016). Comparative sensitivity of aquatic invertebrate and vertebrate species to wastewater from an operational coal mine in central Queensland, Australia. Ecotoxicol. Environ. Saf. 129, 1–9. doi: 10.1016/j.ecoenv.2016.03.003
Lapresta-Fernández, A., Fernández, A., and Blasco, J. (2012). Nanoecotoxicity effects of engineered silver and gold nanoparticles in aquatic organisms. TrAC Trends Anal. Chem. 32, 40–59. doi: 10.1016/j.trac.2011.09.007
León-Silva, S., Fernández-Luqueño, F., and López-Valdez, F. (2016). Silver nanoparticles (AgNP) in the environment: a review of potential risks on human and environmental health. Water Air Soil Pollut. 227:306. doi: 10.1007/s11270-016-3022-9
Li, L., Wu, H., Ji, C., Van Gestel, C. A., Allen, H. E., and Peijnenburg, W. J. (2015). A metabolomic study on the responses of daphnia magna exposed to silver nitrate and coated silver nanoparticles. Ecotoxicol. Environ. Saf. 119, 66–73. doi: 10.1016/j.ecoenv.2015.05.005
Li, Y., Qin, T., Ingle, T., Yan, J., He, W., Yin, J.-J., et al. (2017). Differential genotoxicity mechanisms of silver nanoparticles and silver ions. Arch. Toxicol. 91, 509–519. doi: 10.1007/s00204-016-1730-y
Limbach, L. K., Bereiter, R., Müller, E., Krebs, R., Galli, R., and Stark, W. J. (2008). Removal of oxide nanoparticles in a model wastewater treatment plant: influence of agglomeration and surfactants on clearing efficiency. Environ. Sci. Technol. 42, 5828–5833. doi: 10.1021/es800091f
Liu, J., and Hurt, R. H. (2010). Ion release kinetics and particle persistence in aqueous nano-silver colloids. Environ. Sci. Technol. 44, 2169–2175. doi: 10.1021/es9035557
Mattsson, M.-O., and Simkó, M. (2017). The changing face of nanomaterials: risk assessment challenges along the value chain. Regulat. Toxicol. Pharmacol. 84, 105–115. doi: 10.1016/j.yrtph.2016.12.008
McGillicuddy, E., Murray, I., Kavanagh, S., Morrison, L., Fogarty, A., Cormican, M., et al. (2017). Silver nanoparticles in the environment: sources, detection and ecotoxicology. Sci. Total Environ. 575, 231–246. doi: 10.1016/j.scitotenv.2016.10.041
Metreveli, G., Frombold, B., Seitz, F., Grün, A., Philippe, A., Rosenfeldt, R. R., et al. (2016). Impact of chemical composition of ecotoxicological test media on the stability and aggregation status of silver nanoparticles. Environ. Sci. Nano 3, 418–433. doi: 10.1039/C5EN00152H
Miller, G., and Wickson, F. (2015). Risk analysis of nanomaterials: exposing nanotechnology's naked emperor. Rev. Policy Res. 32, 485–512. doi: 10.1111/ropr.12129
Mitrano, D. M., Lesher, E. K., Bednar, A., Monserud, J., Higgins, C. P., and Ranville, J. F. (2012). Detecting nanoparticulate silver using single-particle inductively coupled plasma-mass spectrometry. Environ. Toxicol. Chem. 31, 115–121. doi: 10.1002/etc.719
Nam, S.-H., Shin, Y.-J., Lee, W.-M., Kim, S. W., Kwak, J. I., Yoon, S.-J., et al. (2015). Conducting a battery of bioassays for gold nanoparticles to derive guideline value for the protection of aquatic ecosystems. Nanotoxicology 9, 326–335. doi: 10.3109/17435390.2014.930531
Navarro, E., Baun, A., Behra, R., Hartmann, N. B., Filser, J., Miao, A.-J., et al. (2008a). Environmental behavior and ecotoxicity of engineered nanoparticles to algae, plants, and fungi. Ecotoxicology 17, 372–386. doi: 10.1007/s10646-008-0214-0
Navarro, E., Piccapietra, F., Wagner, B., Marconi, F., Kaegi, R., Odzak, N., et al. (2008b). Toxicity of silver nanoparticles to Chlamydomonas reinhardtii. Environ. Sci. Technol. 42, 8959–8964. doi: 10.1021/es801785m
Nowack, B., and Bucheli, T. D. (2007). Occurrence, behavior and effects of nanoparticles in the environment. Environ. Pollut. 150, 5–22. doi: 10.1016/j.envpol.2007.06.006
Nugegoda, D., and Rainbow, P. (1988). Effect of a chelating agent (EDTA) on zinc uptake and regulation by Palaemon elegans (Crustacea: Decapoda). J. Mar. Biol. Assoc. U. K. 68, 25–40. doi: 10.1017/S0025315400050074
O'Brien, P., Rainbow, P. S., and Nugegoda, D. (1990). The effect of the chelating agent EDTA on the rate of uptake of zinc by Palaemon elegans (Crustacea: Decapoda). Mar. Environ. Res. 30, 155–159. doi: 10.1016/0141-1136(90)90015-G
OECD (2011). Test No. 201: Freshwater Alga and Cyanobacteria, Growth Inhibition Test. Paris: OECDPublishing.
Pakrashi, S., Tan, C., and Wang, W. X. (2017). Bioaccumulation-based silver nanoparticle toxicity in Daphnia magna and maternal impacts. Environ. Toxicol. Chem. 36, 3359–3366. doi: 10.1002/etc.3917
Park, S., Woodhall, J., Ma, G., Veinot, J. G., and Boxall, A. (2015). Do particle size and surface functionality affect uptake and depuration of gold nanoparticles by aquatic invertebrates? Environ. Toxicol. Chem. 34, 850–859. doi: 10.1002/etc.2868
Park, Y. (2014). New paradigm shift for the green synthesis of antibacterial silver nanoparticles utilizing plant extracts. Toxicol. Res. 30:169. doi: 10.5487/TR.2014.30.3.169
Petersen, E. J., Diamond, S. A., Kennedy, A. J., Goss, G. G., Ho, K., Lead, J., et al. (2015). Adapting OECD aquatic toxicity tests for use with manufactured nanomaterials: key issues and consensus recommendations. Environ. Sci. Technol. 49, 9532–9547. doi: 10.1021/acs.est.5b00997
Phyu, Y. L., Warne, M. S., and Lim, R. (2005). Toxicity and bioavailability of atrazine and molinate to the freshwater shrimp (Paratya australiensis) under laboratory and simulated field conditions. Ecotoxicol. Environ. Saf. 60, 113–122. doi: 10.1016/j.ecoenv.2004.07.006
Posthuma, L., Suter, G. W. II., and Traas, T. P. (2001). Species Sensitivity Distributions in Ecotoxicology. Boca Raton, FL: CRC press. doi: 10.1201/9781420032314
Qin, G., Xiong, Y., Tang, S., Zhao, P., Doering, J. A., Beitel, S. C., et al. (2015). Impact of predator cues on responses to silver nanoparticles in Daphnia carinata. Arch. Environ. Contam. Toxicol. 69, 494–505. doi: 10.1007/s00244-015-0165-4
Rasmussen, K., Rauscher, H., Mech, A., Riego Sintes, J., Gilliland, D., González, M., et al. (2018). Physico-chemical properties of manufactured nanomaterials - Characterisation and relevant methods. An outlook based on the OECD Testing Programme. Regulat. Toxicol. Pharmacol. 92, 8–28. doi: 10.1016/j.yrtph.2017.10.019
Ratte, H. T. (1999). Bioaccumulation and toxicity of silver compounds: a review. Environ. Toxicol. Chem. 18, 89–108. doi: 10.1002/etc.5620180112
Reidy, B., Haase, A., Luch, A., Dawson, K. A., and Lynch, I. (2013). Mechanisms of silver nanoparticle release, transformation and toxicity: a critical review of current knowledge and recommendations for future studies and applications. Materials 6, 2295–2350. doi: 10.3390/ma6062295
Ribeiro, F., Gallego-Urrea, J. A., Jurkschat, K., Crossley, A., Hassellöv, M., Taylor, C., et al. (2014). Silver nanoparticles and silver nitrate induce high toxicity to Pseudokirchneriella subcapitata, Daphnia magna and Danio rerio. Sci. Total Environ. 466, 232–241. doi: 10.1016/j.scitotenv.2013.06.101
Römer, I., White, T. A., Baalousha, M., Chipman, K., Viant, M. R., and Lead, J. R. (2011). Aggregation and dispersion of silver nanoparticles in exposure media for aquatic toxicity tests. J. Chromatogr. A 1218, 4226–4233. doi: 10.1016/j.chroma.2011.03.034
Sakamoto, M., Ha, J. Y., Yoneshima, S., Kataoka, C., Tatsuta, H., and Kashiwada, S. (2015). Free silver ion as the main cause of acute and chronic toxicity of silver nanoparticles to cladocerans. Arch. Environ. Contam. Toxicol. 68, 500–509. doi: 10.1007/s00244-014-0091-x
Seitz, F., Rosenfeldt, R. R., Storm, K., Metreveli, G., Schaumann, G. E., Schulz, R., et al. (2015). Effects of silver nanoparticle properties, media pH and dissolved organic matter on toxicity to Daphnia magna. Ecotoxicol. Environ. Saf. 111, 263–270. doi: 10.1016/j.ecoenv.2014.09.031
Selvakannan, P., Swami, A., Srisathiyanarayanan, D., Shirude, P. S., Pasricha, R., Mandale, A. B., et al. (2004). Synthesis of aqueous Au core-Ag shell nanoparticles using tyrosine as a pH-dependent reducing agent and assembling phase-transferred silver nanoparticles at the air-water interface. Langmuir 20, 7825–7836. doi: 10.1021/la049258j
Sharma, V. K. (2009). Aggregation and toxicity of titanium dioxide nanoparticles in aquatic environment—a review. J. Environ. Sci. Health Part A 44, 1485–1495. doi: 10.1080/10934520903263231
Shen, M. H., Zhou, X. X., Yang, X. Y., Chao, J. B., Liu, R., and Liu, J. F. (2015). Exposure Medium: key in identifying free Ag+ as the exclusive species of silver nanoparticles with acute toxicity to Daphnia magna. Sci. Rep. 5:9674. doi: 10.1038/srep09674
Siripattanakul-Ratpukdi, S., and Fürhacker, M. (2014). Review: issues of silver nanoparticles in engineered environmental treatment systems. Water Air Soil Pollut. 225, 1–18. doi: 10.1007/s11270-014-1939-4
Skjolding, L. M., Sørensen, S. N., Hartmann, N. B., Hjorth, R., Hansen, S. F., and Baun, A. (2016). Aquatic ecotoxicity testing of nanoparticles—the quest to disclose nanoparticle effects. Angew. Chem. Int. Ed. 55, 15224–15239. doi: 10.1002/anie.201604964
Smékalová, M., Panáček, A., Jančula, D., Maršálek, B., Kolarík, J., Prucek, R., et al. (2018). Culture medium mediated aggregation and re-crystallization of silver nanoparticles reduce their toxicity. Appl. Mater. Today 12, 198–206. doi: 10.1016/j.apmt.2018.05.004
Smetanová, S., Bláha, L., Liess, M., Schäfer, R., and Beketov, M. A. (2014). Do predictions from Species Sensitivity Distributions match with field data? Environ. Pollut. 189, 126–133. doi: 10.1016/j.envpol.2014.03.002
Sohn, E. K., Johari, S. A., Kim, T. G., Kim, J. K., Kim, E., Lee, J. H., et al. (2015). Aquatic toxicity comparison of silver nanoparticles and silver nanowires. Biomed Res. Int. 2015:893049. doi: 10.1155/2015/893049
Sørensen, S. N., Hjorth, R., Delgado, C. G., Hartmann, N. B., and Baun, A. (2015). Nanoparticle ecotoxicity-physical and/or chemical effects. Integr. Environ. Assess. Manag. 11, 722–724. doi: 10.1002/ieam.1683
Stebounova, L. V., Guio, E., and Grassian, V. H. (2011). Silver nanoparticles in simulated biological media: a study of aggregation, sedimentation, and dissolution. J. Nanoparticle Res. 13, 233–244. doi: 10.1007/s11051-010-0022-3
Steinhäuser, K. G., and Sayre, P. G. (2017). Reliability of methods and data for regulatory assessment of nanomaterial risks. NanoImpact 7, 66–74. doi: 10.1016/j.impact.2017.06.001
Sun, T. Y., Mitrano, D. M., Bornhöft, N. A., Scheringer, M., Hungerbühler, K., and Nowack, B. (2017). Envisioning nano release dynamics in a changing world: using dynamic probabilistic modeling to assess future environmental emissions of engineered nanomaterials. Environ. Sci. Technol. 51, 2854–2863. doi: 10.1021/acs.est.6b05702
Tejamaya, M., Römer, I., Merrifield, R. C., and Lead, J. R. (2012). Stability of Citrate, PVP, and PEG coated silver nanoparticles in ecotoxicology media. Environ. Sci. Technol. 46, 7011–7017. doi: 10.1021/es2038596
Tian, X., Jiang, X., Welch, C., Croley, T. R., Wong, T.-Y., Chen, C., et al. (2018). Bactericidal effects of silver nanoparticles on Lactobacilli and the underlying mechanism. ACS Appl. Mater. Interfaces 10, 8443–8450. doi: 10.1021/acsami.7b17274
Trottier, S., Blaise, C., Kusui, T., and Johnson, E. (1997). Acute toxicity assessment of aqueous samples using a microplate-based Hydra attenuata assay. Environ. Toxicol. Water Quality 12, 265–271. doi: 10.1002/(SICI)1098-2256(1997)12:3<265::AID-TOX10>3.0.CO;2-9
USEPA (1985). National Recommended Water Quality Criteria - Aquatic Life Criteria Table. Washington, DC: U.S. Environmental Protection Agency.
USEPA (2012). Nanomaterial Case Study: Nanoscale Silver in Disinfectant Spray (Final Report). Washington, DC, EPA/600/R-10/081F: U.S. Environmental Protection Agency.
USEPA (2015). Chemical Substances When Manufactured or Processed as Nanoscale Materials: TSCA Reporting and Recordkeeping Requirements. U.S.EPA. Available online at: http://www.regulations.gov/document?D=EPA-HQ-OPPT-2010-0572-0001.
Völker, C., Boedicker, C., Daubenthaler, J., Oetken, M., and Oehlmann, J. (2013). Comparative toxicity assessment of nanosilver on three Daphnia species in acute, chronic and multi-generation experiments. PLoS ONE 8:e75026. doi: 10.1371/journal.pone.0075026
Von der Ohe, P. C., and Liess, M. (2004). Relative sensitivity distribution of aquatic invertebrates to organic and metal compounds. Environ. Toxicol. Chem. 23, 150–156. doi: 10.1897/02-577
Wang, Y. F., Westerhoff, P., and Hristovski, K. D. (2012). Fate and biological effects of silver, titanium dioxide, and C-60 (fullerene) nanomaterials during simulated wastewater treatment processes. J. Hazard. Mater. 201, 16–22. doi: 10.1016/j.jhazmat.2011.10.086
Wasmuth, C., Rüdel, H., Düring, R.-A., and Klawonn, T. (2016). Assessing the suitability of the OECD 29 guidance document to investigate the transformation and dissolution of silver nanoparticles in aqueous media. Chemosphere 144, 2018–2023. doi: 10.1016/j.chemosphere.2015.10.101
Wei, L., Lu, J., Xu, H., Patel, A., Chen, Z.-S., and Chen, G. (2015). Silver nanoparticles: synthesis, properties, and therapeutic applications. Drug Discov. Today 20, 595–601. doi: 10.1016/j.drudis.2014.11.014
Xia, T., Kovochich, M., Liong, M., Mädler, L., Gilbert, B., Shi, H., et al. (2008). Comparison of the mechanism of toxicity of zinc oxide and cerium oxide nanoparticles based on dissolution and oxidative stress properties. ACS Nano 2, 2121–2134. doi: 10.1021/nn800511k
Yue, Y., Li, X., Sigg, L., Suter, M. J., Pillai, S., Behra, R., et al. (2017). Interaction of silver nanoparticles with algae and fish cells: a side by side comparison. J. Nanobiotechnol. 15:16. doi: 10.1186/s12951-017-0254-9
Zhao, C. M., and Wang, W. X. (2012). Importance of surface coatings and soluble silver in silver nanoparticles toxicity to Daphnia magna. Nanotoxicology 6, 361–370. doi: 10.3109/17435390.2011.579632
Keywords: silver, nanoparticles, toxicity, freshwater, species, daphnia, hydra, shrimp
Citation: Lekamge S, Miranda AF, Abraham A, Li V, Shukla R, Bansal V and Nugegoda D (2018) The Toxicity of Silver Nanoparticles (AgNPs) to Three Freshwater Invertebrates With Different Life Strategies: Hydra vulgaris, Daphnia carinata, and Paratya australiensis. Front. Environ. Sci. 6:152. doi: 10.3389/fenvs.2018.00152
Received: 18 October 2018; Accepted: 29 November 2018;
Published: 13 December 2018.
Edited by:
Eduardo Alves Almeida, Universidade Regional de Blumenau, BrazilReviewed by:
Denis Moledo De Souza Abessa, São Paulo State University, BrazilSamuel Ita Eduok, University of Uyo, Nigeria
Copyright © 2018 Lekamge, Miranda, Abraham, Li, Shukla, Bansal and Nugegoda. This is an open-access article distributed under the terms of the Creative Commons Attribution License (CC BY). The use, distribution or reproduction in other forums is permitted, provided the original author(s) and the copyright owner(s) are credited and that the original publication in this journal is cited, in accordance with accepted academic practice. No use, distribution or reproduction is permitted which does not comply with these terms.
*Correspondence: Sam Lekamge, samlekamge@gmail.com