Microbial Biomass Responses to Soil Drying-Rewetting and Phosphorus Leaching
- 1School of Engineering and the Environment, Kingston University, London, United Kingdom
- 2Rothamsted Research, Okehampton, United Kingdom
- 3School of Life Sciences, Pharmacy and Chemistry, Kingston University, London, United Kingdom
Soil drying-rewetting is known to enhance soil phosphorus leaching, which in part is due to osmotic shock and lysis of microbial cells upon rewetting. However, it is not entirely clear how this may be influenced by the intensity and duration of soil drying. We hypothesized that the intensity and duration of soil drying play important roles in determining the extent of dissolved reactive phosphorus (DRP) leaching resulting from microbial biomass mortality. To test this hypothesis soil sub-samples of a loamy grassland soil were dried (30 or 40°C for 2 or 14-days), rewetted, and the leachate was analyzed for DRP. Soil drying at 30°C for 2 and 14-days resulted in leachate DRP concentrations which were 71 and 271%, respectively, higher than those in leachate from a control moist counterpart. Relatively greater DRP leaching losses occurred from the soil dried at 40°C for 2 and 14-days (143 and 300%, respectively). To determine the contribution of the microbial biomass to the DRP in leachate, soil sub-samples were fumigated with chloroform either before or after drying (30 or 40°C for 2 or 14-days). All soil treatments were then either leached with water and analyzed for DRP or extracted with 0.5 M sodium bicarbonate solution and analyzed for microbial biomass phosphorus. Fumigating soil samples before or after drying reduced microbial biomass phosphorus. However, the effect of chloroform fumigation was more pronounced in terms of microbial biomass reduction in the DF (drying followed by fumigation) treatment. Moreover, results revealed that in the DF treatment, soils dried at 30°C for 2-days and 14-days had 22 and 13%, respectively, more microbial biomass phosphorus than their counterparts dried at 40°C for 2 and 14-days, respectively. These results suggest that soil drying at higher intensity and for prolonged periods significantly (p < 0.05) affect microbial biomass and subsequently increases soil phosphorus leaching following rewetting, due to enhanced contributions from the microbial biomass. These findings, however, need to be verified over a range of soil types under natural field conditions to better assess soil drying-rewetting effects on nutrient leaching.
Introduction
Predicted changes in climate will potentially increase the intensity and frequency of soil drying-rewetting (DRW) cycles (IPCC, 2014), with implications for nutrient dynamics (plant availability, sequestration, and leaching), crop productivity and catchment water quality. Recent studies have tested the effects of soil DRW cycles on nutrient release. There is some evidence that soil DRW cycles can increase the extractability of soil nutrients (Bunemann et al., 2013; Forber et al., 2017; Sun et al., 2017; Dinh et al., 2018; Homberg and Matzner, 2018). For instance, Koopmans et al. (2006); Styles and Coxon (2006) and Bunemann et al. (2013) reported increased extractability of phosphorus (P) as a result of soil drying. Gordon et al. (2008) reported that DRW induced significant increases in dissolved organic carbon (DOC), dissolved organic nitrogen (DON), and dissolved inorganic nitrogen (DIN) in the leachates from two UK grassland soils. These findings support the notion that the changing pattern of climate has the potential to alter soil nutrient dynamics.
Enhanced nutrient extractability following soil drying-rewetting has been attributed to the disruptive effects of these soil stresses on the soil microbial biomass (Achat et al., 2010; Turner and Romero, 2010; Bunemann et al., 2013; Dinh et al., 2018; Brodlin et al., 2019), aggregate stability (Koopmans et al., 2006; Xiang et al., 2008; Bunemann et al., 2013), and stability of organic matter and organo-metallic complexes (Peltovuori and Soinne, 2005; Styles and Coxon, 2006; Soinne et al., 2010). However, the response to wetting in terms of quantities of nutrients released varies with soil type (Zhao et al., 2010; Achat et al., 2012a,b), microbial tolerance to withstand soil stresses (Styles and Coxon, 2006; Gordon et al., 2008; Bunemann et al., 2013; Dinh et al., 2017), rate of soil rewetting (Turner and Haygarth, 2001; Blackwell et al., 2009, 2013), intensity of drying (Sardans and Penuelas, 2007; Bunemann et al., 2013; Sun et al., 2017), and drying duration (Forber et al., 2017).
The soil microbial biomass plays an important role in nutrient cycling by acting as a source (e.g., mineralization of soil organic matter, releasing nutrients from cytoplasm as a mechanism to equilibrate with surroundings, and cell bursting) or sink by immobilizing nutrients (Achat et al., 2010; Dinh et al., 2017; Wang et al., 2017; Zhang and Marschner, 2018). Reductions in soil microbial biomass following soil DRW have been reported in recent years (Mondini et al., 2002; Blackwell et al., 2013; Chen et al., 2016). Mondini et al. (2002) reported a decrease of 13% in microbial biomass C and 30% in ninhydrin reactive N relative to a moist control following drying. Wu and Brookes (2005) reported a reduction in biomass C of 44% as a result of soil DRW, while Blackwell et al. (2010) reported that soil air-drying and re-wetting may kill up to 70% of the total soil microbial biomass. Reduction in microbial biomass following a DRW stress is attributed to the rapid hydration of microbial cells. If microorganisms do not quickly equilibrate to sudden changes in water potential, their cell walls can burst, and cell lysis takes place. Biomass surviving soil drying are typically a better adapted part of the microbial population which can survive drying stresses by making protective structures e.g., spores or cysts (Borken and Matzner, 2009; Blackwell et al., 2010).
Although the soil microbial biomass typically comprises only a small percentage (1–3%) of soil organic matter (Blackwell et al., 2010), it may contain large quantities of phosphorus (Brookes et al., 1984). Currently studies reporting microbially driven soil phosphorus leaching are either limited (Blackwell et al., 2009, 2013) or have considered only a single drying temperature or duration. The novelty of the research reported here lies in how phosphorus leaching is affected by increasing the intensity and duration of drying. The selection of drying temperatures of 30 and 40°C may seem unrealistic within the UK context, but surface soils have been reported to experience such high temperatures during summer (c.f. Blackwell et al., 2009). Moreover, to our knowledge, the effects of chloroform fumigation on soil microbial biomass phosphorus leaching have not yet been studied in pre- and post-dried soil samples. This work investigates how the soil microbial biomass responds to fumigation in the presence or absence of soil moisture and impacts on dissolved reactive phosphorus (DRP) leaching. In the above context the basic objectives of our controlled laboratory experiments were to: (1) test the hypothesis that increases in the intensity and duration of soil drying will significantly increase concentrations of DRP in leachates, (2) determine the soil microbial biomass phosphorus contribution to enhanced leaching of DRP following DRW, and (3) examine how the soil microbial biomass responds to chloroform fumigation in pre- and post-drying soil samples—this will help understand how soil drying-rewetting influences phosphorus leaching when biomass is killed before or after drying. Phosphorus leaching from soils occurs in the forms of DRP, dissolved organic P and particulate-bound P (Hooda et al., 1999). This work, however, considers only DRP leaching because this is the most important factor in terms of water quality and eutrophication as noted in the Water Environment1.
Materials and Methods
Sample Collection and Preparation
The study site is located in North Wyke, Devon, UK and is described in Harrod and Hogan (2008). A bulk composite topsoil (0–10 cm) sample (from nine randomly selected locations) was collected in August 2014 from a sheep and beef cattle grazed permanent grassland field, comprising an area of approximately 3.47 hectares. The soil was non-calcareous typic haplaquept (USDA) of the Hallsworth series (FAO Stagni-vertic cambisol) (hereafter referred to as Hallsworth soil). Soil was prepared by crushing and passing through a 2 mm sieve, removing all non-soil material (grass, roots, earthworms, and stones). Soil was moistened to 54% of the maximum water holding capacity (WHC). For the purpose of analysis, a sub-sample of the soil was oven-dried at 40°C till no further loss in the moisture was observed and the remaining soil was stored at 3°C at 54% of the maximum WHC until required.
Drying-Rewetting Effects on Fumigated and Non-fumigated Soil Samples
Soil sub-samples were fumigated with chloroform either before or after oven drying at 30°C or 40°C for either 2-days or 14-days (2-weeks). Soil was weighed before and after drying to measure the moisture loss at different temperatures. The average moisture contents after 2-days drying were 3.7 and 1.1% at 30 and 40°C, respectively, and after 2-days no further loss in moisture was observed. A control soil was stored moist in vented plastic bags at 3°C until required and maintained at room temperature (21°C) for a day before subjecting to leaching. Each sample was then split into two sub-samples, with one sub-sample used for extracting microbial biomass phosphorus based on the method described by Brookes et al. (1982), while the other sub-sample was leached with deionized (DI) water (18 MΩ) as follows: three replicates, each comprising 30 g dry-weight equivalent (DWE) of soil, gently compacted (to 1.2 g/cm3 density) in plastic Buchner funnels with internal diameter of 76 mm and capacity 186 ml. Grade 1 qualitative filter paper (GE Healthcare Whatman) was placed at the bottom of funnels. In total 60 replicates of the moist unfumigated (MUF), moist fumigated (MF), dried unfumigated (DUF), dried fumigated (DF), and fumigated dried (FD) treatments were prepared in the leaching experiment for the measurement of DRP. Soil was watered with 105 ml of deionized water applied gently in several portions (to mitigate bypass flow and high hydrophobicity effects) over a period of 2 h, using a syringe, simulating an intense rainfall event (approximately equivalent to 23 mm rainfall). Collected leachates (~65 ml/funnel) were filtered through Whatman 0.45 μm cellulose nitrate membrane filters and analyzed within 24 h of collection for DRP by the molybdate blue method. All soil treatments, including the control soil, were rewetted to the same moisture content. Table 1 shows the treatments imposed.
Laboratory Analyses
Soil was characterized in detail (Table 2) using standard procedures and quality controls. In all cases triplicate soil samples (n = 3) were analyzed. Soil pH was measured in 1:2.5 (w/v) soil-water suspensions, using a pre-calibrated pH meter (Oakton™ pH 700 Benchtop Meter). Soil texture was determined by the hydrometer method following pre-treatment with hydrogen peroxide (H2O2), as outlined in Rowell (1994). Soil organic carbon was determined by the Walkley-Black potassium dichromate oxidation method (Walkley and Black, 1934). Cation exchange capacity of the soil was determined by sodium saturation (sodium acetate) and displaced sodium (using ammonium acetate) was then analyzed by flame emission spectrometry (Chapman, 1965). Soil total phosphorus was determined by digesting finely ground soil in perchloric acid (HClO4) on a hot plate for about 40 min at 203°C until the dark color of organic matter disappeared. Digests were filtered (Whatman 541), diluted to 250 ml with de-ionized water and phosphorus in the digestates determined by ICP-AES (Inductively coupled plasma atomic emission spectroscopy; JobinYvon Horiba - ULTIMA 2C/2CE) (Carter and Gregorich, 2008). For the measurement of soil organic phosphorus, soil was ignited at 550°C for 2 h. Both ignited and unignited samples were extracted by 0.5 M H2SO4 before analyzing them by ICP-AES. Total organic phosphorus was then calculated as the difference between phosphorus content of ignited and unignited samples (Carter and Gregorich, 2008).
Bicarbonate extractable phosphorus was measured based on the method described by Olsen (1954). Briefly, 5 g DWE of soil was extracted in 100 ml of 0.5 M NaHCO3 solution adjusted to pH 8.5 along with 1 g acid washed charcoal on a shaker for 1 h. Extracts were then filtered (Whatman No. 42), acidified (0.25 MH2SO4), and phosphorus determined colorimetrically by the Murphy and Riley (1962) method. Microbial biomass phosphorus was measured in both moist and dry soil replicates using the chloroform fumigation-extraction method as described by Brookes et al. (1982). Briefly, three sets each comprising three replicate samples of the soil (5 g DWE) were prepared (all moist and dry soil treatments). One set was sterilized using chloroform fumes under vacuum in a desiccator jar and incubated for 24 h in the dark at 25°C. The second and third sets were incubated in the dark without chloroform in desiccator jars for the same period and at the same temperature. The second unfumigated set was used as a control and the third unfumigated set was spiked with 250 μg P ml−1 solution (1.0975 g KH2PO4 into 1 L DI water). All samples (control, fumigated and spiked) were then extracted with 0.5 M NaHCO3 (100 ml) adjusted to pH 8.5 along with 1 g acid washed charcoal. Each sample was then acidified (5–6 drops of 0.25 M H2SO4/50 ml of sample). The amount of orthophosphate in the NaHCO3 extracts was measured colorimetrically by the Murphy and Riley (1962) method.
Statistical Analysis
The significance of differences between treatments was determined by one-way ANOVA (significance reported as p < 0.05) by SPSS (IBM SPSS statistics 23). Tukey's post-hoc test was employed for multiple comparisons. The data are reported as mean ± standard deviation. Dependent variables were normalized using log10 transformation, when they did not follow a normal distribution to meet the ANOVA assumptions. Effect of interaction between the independent variables (e.g., temperature, duration, and fumigation) on the dependent variables (e.g., DRP concentration or microbial biomass phosphorus) was determined using three-way ANOVA (Table 3). Pearson's correlation analysis was performed to explore relationship between DRP and microbial biomass P in both DF and FD treatments using SPSS (IBM SPSS statistics 23).
Results
Effect of Drying-Rewetting on DRP Leaching
Upon drying, the quantities of DRP in leachates significantly (p < 0.05) increased for all dried treatments (Figure 1). The largest percentage increase in DRP occurred in the soil dried at 40°C for 14-days (300%), relative to the moist unfumigated control. The smallest increase in DRP was observed in the leachate derived from the soil dried at 30°C for 2-days (72% increase) relative to the unfumigated moist control.
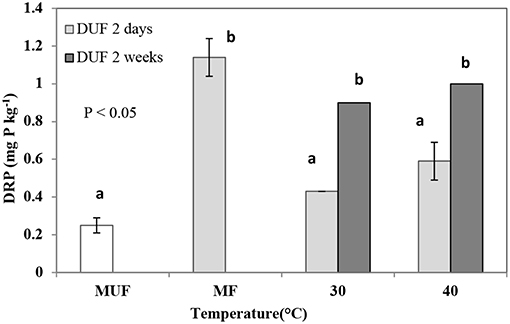
Figure 1. DRP (mg P kg−1) leached in 2 and 14-days dried unfumigated (DUF) treatments at 30 and 40°C. Error bars represent standard deviation (for some columns error bars are too small to be seen), n = 3. MUF, moist unfumigated soil and MF, moist fumigated soil. Means with the same letter are not significantly different from each other at the significance level of P < 0.05 as determined using Tukey's post-hoc test.
For DUF treatments, DRP quantities in the leachates derived from soils dried for 14-days at 30°C and 40°C (0.9 ± 0.2 and 1.0 ± 0.2 mg P kg−1, respectively) were almost double the corresponding DRP quantities in the leachates from soils dried for 2-days at 30 and 40°C (0.43 ± 0.01 and 0.59 ± 0.10 mg P kg−1, respectively; Figure 1). DRP concentration from the moist fumigated (MF) soil was as much as four times (p < 0.001) more than from the corresponding moist unfumigated (MUF) samples (Figure 1).
Two-days drying followed by fumigation treatment (DF) leached 88% and 46% more DRP in the leachates from the soils dried at 30 and 40°C, respectively, with concentrations of 0.75 ± 0.11 mg P kg−1 and 0.86 ± 0.13 mg P kg−1 respectively (Figure 2), relative to 2-days dried unfumigated controls (0.4 ± 0.01 mg P kg−1and 0.59 ± 0.10 mg P kg−1, respectively). Similarly, 14-days drying followed by fumigation (DF) treatment released 11% and 10% more DRP (with concentrations of 1.0 ± 0.2 and 1.1 ± 0.2 mg P kg−1) at 30°C and 40°C, respectively, relative to their 14-days dried unfumigated controls (0.9 ± 0.2 and 1.0 ± 0.2 mg P kg1, respectively). However, these differences in DRP (DF at 30°C and 40°C for 14-days) were not statistically significant (p > 0.05) compared to their dried unfumigated (DUF) counterparts (Figure 3).
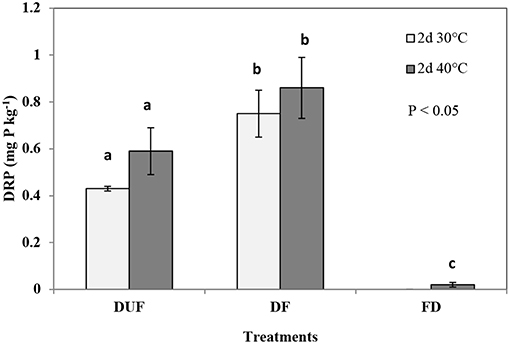
Figure 2. Comparing DRP (mg P kg−1) leached in 2-days drying, unfumigated (DUF), with drying followed by fumigation (DF), and fumigation followed by drying (FD) treatments. Error bars represent standard deviation (for some columns error bars are too small to be seen), n = 3. Means with the same letter are not significantly different from each other at the significance level of P < 0.05 as determined using Tukey's post-hoc test.
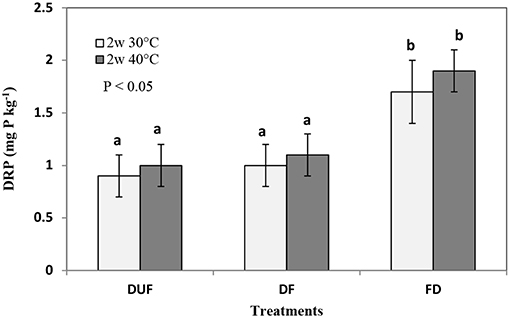
Figure 3. Comparing DRP (mg P kg−1) leached in 14-days drying, unfumigated (DUF), with drying followed by fumigation (DF), and fumigation followed by drying treatments (FD). Error bars represent standard deviation, n = 3. Means with the same letter are not significantly different from each other at the significance level of P < 0.05 as determined using Tukey's post-hoc test.
In the 2-days FD treatment, DRP concentrations in the leachates from soil dried at 30°C were below the level of detection (< LOD) (Figure 2). In contrast, significantly greater quantities of DRP (p < 0.001) were leached in the same treatment (FD) when drying duration was increased to 14-days, with concentrations of 1.7 ± 0.3 mg P kg−1 and 1.9 ± 0.2 mg P kg−1 at 30 and 40°C, respectively (89 and 90% increase relative to that from dried unfumigated counterparts; Figure 3).
Microbial Biomass Contribution to Enhanced DRP Leaching
Results from the leaching experiment showed that fumigating pre-or post-drying increased DRP leaching, with the exception of the 2 days FD (fumigated dried) leaching treatment where DRP was not detected (< LOD) in the leachates from soils dried at 30°C (Figures 2, 3). Also, the effect of soil fumigation on DRP leaching appears not to be clearly influenced by the intensity and duration of drying (Figures 2, 3).
Comparing DRP in leachate from unfumigated and fumigated treatments suggest that increased DRP leaching could in part be caused by microbial cell lysis. To investigate the role of the soil microbial biomass in this phenomenon, biomass associated phosphorus was extracted. Results from the sodium bicarbonate extraction experiment showed that microbial biomass phosphorus in the control soil sample was significantly (p < 0.05) higher than in all treatments with dried soils (Figure 4).
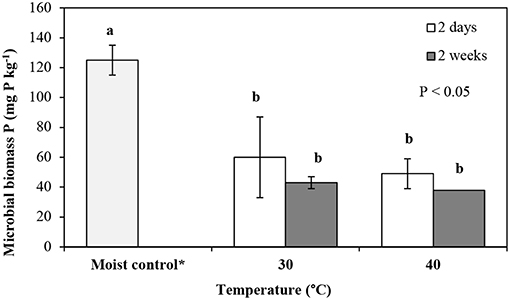
Figure 4. Microbial biomass phosphorus (mg P kg−1) extracted in samples dried for 2 and 14-days followed by fumigation (DF) treatments at 30 and 40°C. Error bars represent standard deviation (for some columns error bars are too small to be seen), n = 3. Means with the same letter are not significantly different from each other at the significance level of P < 0.05 as determined using Tukey's post-hoc test. *Moist control is reference moist soil (MF) stored at 3°C.
In the case of the 2-days DF treatment, soil drying at 30 and 40°C reduced microbial biomass phosphorus by 52 and 61%, respectively, with concentrations of 60 ± 27 mg P kg−1 and 49 ± 10 mg P kg−1, respectively, relative to that of the control soil (125 ± 10 mg P kg−1) (Figure 4). In general, soil drying for a longer duration and at a greater intensity caused a significant reduction in microbial biomass phosphorus (p < 0.05), relative to the control. The greatest decrease occurring in the microbial biomass phosphorus in soil dried at 40°C for 14-days (70% decrease relative to the control soil microbial biomass phosphorus), in the DF treatment (Figure 4). In the case of the 2-days DF treatment, microbial biomass phosphorus was reduced by 18 and 28%, with the increase in drying intensity (from 30 to 40°C) and duration (from 2 to 14-days), respectively, the concentrations ranged from 60 ± 27 to 49 ± 10 mg P kg−1 and 60 ± 27 to 43 ± 11 mg P kg−1, respectively. However, these differences in microbial biomass P due to soil drying intensity and duration were not statistically significant (Figure 4).
Fumigation followed by drying (FD) treatments generally showed similar trends, with a reduction in microbial biomass phosphorus following fumigation and drying relative to the control soil. However, no reduction in microbial biomass phosphorus was observed when the soil was dried for 2-days at 30°C (Figure 5).
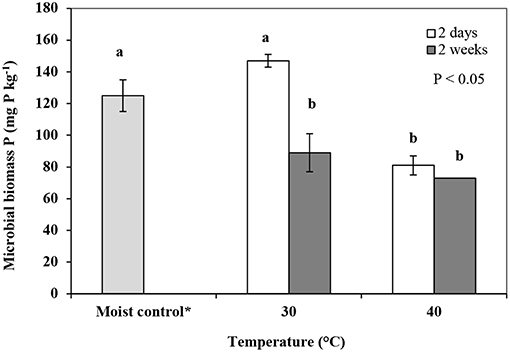
Figure 5. Microbial biomass phosphorus (mg P kg−1) extracted in samples fumigated followed by drying for 2 and 14-days (FD) treatments at 30 and 40°C. Error bars represent standard deviation, n = 3. Means with the same letter(s) are not statistically significant from each other i.e., P > 0.05 as determined using a Tukey post-hoc test. *Moist control is reference moist soil (MF) stored at 3°C.
Discussion
Our results demonstrate that in all treatments DRP quantities significantly (p < 0.05) increased in the leachates from soils dried for a longer duration (14-days) and at a greater intensity (40°C) compared to the corresponding soil samples which were subjected to drying at a lower temperature (30°C) and for a shorter duration (2-days) (Figure 1). We can, therefore, accept the hypothesis that increases in the duration and intensity of drying of soils significantly increases the concentrations of DRP in leachate from the soil used in this experiment. It is likely that more intense soil drying exerts a greater stress on the microbial biomass and thus renders greater release of nutrients upon rewetting. In our study the observed reduction in microbial biomass phosphorus upon drying (Figures 4, 5) explains, at least in part, the increase in DRP in leachates from all treatments (Figures 1–3). This is further supported by a negative relationship between DRP and microbial biomass phosphorus, however, the Pearson's correlation was significant (r = −0.604, p < 0.05) only for the DF treatment. Drying-rewetting induced reduction in microbial biomass has been reported previously (Kaiser et al., 2015; Chen et al., 2016; Brodlin et al., 2019). Results from the NaHCO3 extraction experiment showed that drying at a greater intensity caused a greater reduction in soil microbial biomass phosphorus compared to drying at the lower temperature (30°C) (Figure 5).
Drying duration had a greater impact on DRP leaching relative to drying intensity. Extended periods of drying may cause substantial reductions in microbial activity and microbial biomass due to reduced diffusive transport of soluble nutrients and water-dependent activities for prolonged periods (Blackwell et al., 2010). Extended drying and subsequent reduction in microbial biomass could increase nutrient losses upon rewetting due to several factors e.g., accumulated dead microbial cells, release of intracellular solutes, aggregate disruption, and exposure of previously protected labile organic matter, and mineralization of organic matter in the soil (Borken and Matzner, 2009). Results from the leaching experiments showed that soil drying for 14-days generally resulted in the higher concentrations of DRP in leachates relative to the corresponding samples where soil was dried for 2-days (Figure 1). One of the factors contributing to the increase in DRP concentrations in the leachates derived from soil dried for the longer duration was likely to be due to the greater destruction of microbial biomass, as indicated by the microbial biomass phosphorus measurements, which showed significant reductions (p < 0.05) in soils dried for 14-days compared to the control soil (Figures 4, 5). Moreover, prolonged drought slows down microbial growth rate and enzymatic activity by causing sub-lethal damages to cell structure (e.g., DNA, proteins, cell wal; Meisner et al., 2015; Rahman et al., 2018), subsequently increasing potentially leachable phosphorus. This could be another possible reason of higher leaching of phosphorus in the 14-days treatments.
Fumigating soils also influenced DRP in leachates relative to the non-fumigated counterparts (Figures 2, 3). However, the influence was not straightforward e.g., 2-days FD or 2-weeks DF drying treatments where, either no DRP was detected in the leachate (Figure 2) or it was similar to that from the moist control soil (Figure 3). Nonetheless, these results are generally consistent with Bunemann et al. (2013). They observed that drying-rewetting of sterilized soil samples (gamma irradiation and autoclaved) significantly increased resin extractable phosphorus. The observed increased DRP concentrations in leachates following soil fumigation with chloroform in our study are most likely due to lysis of microbial cells and consequent release of phosphorus. Moreover, microbes that survive fumigation (Kieft et al., 1987) can increase their population size by utilization of nutrients made available by subsequent hydrolysation of organic compounds released from microorganisms killed during sterilization (Bunemann et al., 2013). Such a shift in microbial community following soil drying has been observed in drying-rewetting studies (e.g., Butterly et al., 2009; Pezzolla et al., 2019). This process can also result in an increase in mineralized phosphorus (DRP) availability for leaching. Hydrolysis of organic phosphorus compounds could also be one of the possible reasons of increased DRP in the leachate. This explanation, however, remains speculative since in this study we did not measure leachate dissolved organic phosphorus (DOP). Measuring DOP would have allowed a better understanding of the underlying processes, particularly the role of microbial biomass-P and transformation between inorganic and organic P.
Soil drying followed by fumigation (DF) resulted in greater concentrations of DRP relative to the DUF treatments (though the concentration increase was significant only in the case of the 2-days DF and two-weeks FD treatments; Figures 2, 3).This may be because soil drying kills microbial species less adapted to drying. Thus, the biomass surviving drying stress represents a relatively more adapted fraction of the microbial population which can survive drying stress by making protective structures e.g., spores or cysts (Bottner, 1985; Schimel et al., 1999). Subjecting dried soil to chloroform fumigation could have resulted in death of microbial species which survived the original drying stress, consequently enhancing DRP concentrations in the leachate.
However, all DF treatments leached lower concentrations of phosphorus relative to the moist fumigated counterpart (MF) treatment (Figures 1–3). The greater DRP concentrations in leachates from moist fumigated soils probably occurred because of the presence of a larger microbial biomass, since fresh moist soil microbial biomass is always greater than the dried soil microbial biomass, as drying kills some of the native biomass (Blackwell et al., 2013). Moreover, drying before fumigation potentially opens-up the soil aggregates more, exposing more surfaces for phosphorus binding so that when the soil is fumigated after drying, more of the phosphorus released gets bound to the surfaces of newly exposed/accessible soil particles (e.g., clay surfaces), potentially reducing the amount of phosphorus availability for leaching.
In the 2-days FD leaching treatment DRP was not detected in the leachate (< LOD) from the soil dried at 30°C. It is possible that phosphorus assimilation by the recolonizing microbial population as well as retention on soil colloids due to drying induced crystallization (Schonbrunner et al., 2012; Dieter et al., 2015) could be the cause of this result (Figure 2). This is supported at least partially by the NaHCO3 extraction results, which showed no reduction in microbial biomass phosphorus in the soil samples which were fumigated before subjecting to oven drying at 30°C for 2-days (FD treatment; Figure 5), unlike the DF treatment (Figure 4). Immobilization of nutrients by the recolonizing microbial population has also been previously reported (Brookes et al., 1982; Yevdokimov et al., 2016). It is possible that upon warming of soil as the soil drying proceeded, microbial activity at least initially increased causing assimilation of phosphorus released from dead cells as well as soil available mineral phosphorus. The highest concentrations of DRP in leachates came from the 14-days (2 weeks) FD treatments dried at both 30 and 40°C (Figure 3). Significant increases in DRP concentrations in leachates could be associated with the greater impact of prolonged soil drying and subsequent rewetting on a fraction of the microbial biomass that are poorly adapted to drying stresses. The reduction in microbial biomass phosphorus in the 14-days FD treatment at 30 and 40°C was less (Figure 5) relative to the reduction observed in the 14-days DF treatments at 30 and 40°C (Figure 4). This may be due to the effectiveness of the fumigation process, whereby in a dried soil the chloroform has better access to all the micropores, which in a moist soil will be at least partially filled with water preventing the access of chloroform, meaning more microbes survive the fumigation process. Moreover, it is also likely that fumigation followed by drying (FD) for an extended period of time (14-days) triggered microbial growth due to the availability of nutrients from the dead biomass and moisture during moist incubation in the initial stages of oven-drying before the moist-dry threshold was reached. This explanation can be supported by Voroney (2007) and Dinh et al. (2017) who state that fungal communities are better adapted to survive drying stress due to their thick cell wall structure limiting water loss and can increase their biomass due to their greater ability to utilize nutrients released from other microbes. However, fungal communities are less adapted to drying-rewetting stresses likely due to their location at the surfaces of soil aggregates (Blackwell et al., 2010). This work did not consider repeated drying-wetting; nonetheless, some of the observed enhanced leaching of DRP in the 14-days FD treatment might have been contributed by less adapted fungal communities to drying-rewetting stresses.
Conclusions
The results clearly support our hypothesis that increase in the duration and intensity of drying of soils significantly increases the concentration of DRP in leachate relative to the control from the soil used in this experiment. A reduction in microbial biomass phosphorus as a result of soil drying is the most likely reason that the quantities of DRP in leachates increased significantly relative to the control, concurrent with the drying intensity and duration. The results show that soil drying at higher intensity and for prolonged duration affect the microbial biomass to a greater extent than low intensity-short duration drying, and subsequently cause the leaching of higher concentrations of DRP following rewetting. However, drying duration seems to have a greater influence on DRP concentrations in leachates relative to drying intensity. This means high intensity-short duration drying conditions may not mobilize as much phosphorus as more persistent drying at moderate temperatures. Our results also showed that fumigating soil samples before or after drying exhibit similar trends with reduction in microbial biomass phosphorus concurrent with drying intensity and duration. However, the effect of chloroform fumigation was more pronounced in terms of microbial biomass reduction in the DF treatments. These results indicate that the patterns of soil drying and rewetting under future climate change could have significant impacts on DRP leaching from soils, and add to the growing body of evidence on this topic. However, there remains a need to understand soil drying-rewetting impacts on DRP leaching on a wider scale and in a range of soil types, as well as under natural field conditions, to obtain a more realistic assessment of nutrient leaching.
Data Availability
All datasets generated for this study are included in the manuscript/supplementary files.
Author Contributions
SK, PH, and MB equally contributed to the conceptual development, field sampling, and writing of the manuscript. SK led experimental design, material analysis, and data analysis. RB contributed to laboratory analysis and manuscript preparation.
Funding
This work was supported by Kingston University London, United Kingdom.
Conflict of Interest Statement
The authors declare that the research was conducted in the absence of any commercial or financial relationships that could be construed as a potential conflict of interest.
Acknowledgments
We thank Kingston University London for funding this research in the form of a Ph. D. Studentship Award to SK.
Footnotes
1. ^Water Environment (Water Framework Directive) (England and Wales) (Amendment) Regulations 2015. Available online at: http://www.legislation.gov.uk/uksi/2015/1623/pdfs/uksi_20151623_en.pdf (accessed August 7, 2019).
References
Achat, D. L., Augusto, L., Bakker, M. R., Gallet-Budynek, A., and Morel, C. (2012a). Microbial processes controlling P availability in forest spodosols as affected by soil depth and soil properties. Soil Biol. Biochem. 44, 39–48. doi: 10.1016/j.soilbio.2011.09.007
Achat, D. L., Augusto, L., Gallet-Budynek, A., and Bakker, M. R. (2012b). Drying-induced changes in phosphorus status of soils with contrasting soil organic matter contents–implications for laboratory approaches. Geoderma 187, 41–48. doi: 10.1016/j.geoderma.2012.04.014
Achat, D. L., Bakker, M. R., Zeller, B., Pellerin, S., Bienaimé, S., and Morel, C. (2010). Long-term organic phosphorus mineralization in Spodosols under forests and its relation to carbon and nitrogen mineralization, Soil Biol. Biochem. 42, 1479–1490. doi: 10.1016/j.soilbio.2010.05.020
Blackwell, M., Brookes, P., de La Fuente-Martinez, N., Gordon, H., Murray, P., Snars, K., et al. (2010). Phosphorus solubilization and potential transfer to surface waters from the soil microbial biomass following drying–rewetting and freezing–thawing. Adv. Agron. 106, 1–35. doi: 10.1016/S0065-2113(10)06001-3
Blackwell, M., Brookes, P., De la Fuente-Martinez, N., Murray, P., Snars, K., Williams, J., et al. (2009). Effects of soil drying and rate of re-wetting on concentrations and forms of phosphorus in leachate. Biol. Fertil. Soils 45, 635–643. doi: 10.1007/s00374-009-0375-x
Blackwell, M. S., Carswell, A. M., and Bol, R. (2013). Variations in concentrations of N and P forms in leachates from dried soils rewetted at different rates. Biol. Fertil. Soils 49, 79–87. doi: 10.1007/s00374-012-0700-7
Borken, W., and Matzner, E. (2009). Reappraisal of drying and wetting effects on C and N mineralization and fluxes in soils. Glob. Chang. Biol. 15, 808–824. doi: 10.1111/j.1365-2486.2008.01681.x
Bottner, P. (1985). Response of microbial biomass to alternate moist and dry conditions in a soil incubated with 14 C-and 15 N-labelled plant material. Soil Biol. Biochem. 17, 329–337. doi: 10.1016/0038-0717(85)90070-7
Brodlin, D., Kaiser, K., Kessler, A., and Hagedorn, F. (2019). Drying and rewetting foster phosphorus depletion of forest soils. Soil Biol. Biochem. 128, 22–34. doi: 10.1016/j.soilbio.2018.10.001
Brookes, P. C., Powlson, D. S., and Jenkinson, D. S. (1982). Measurement of microbial biomass phosphorus in soil. Soil Biol. Biochem. 14, 319–329. doi: 10.1016/0038-0717(82)90001-3
Brookes, P. C., Powlson, D. S., and Jenkinson, D. S. (1984). Phosphorus in the soil microbial biomass. Soil Biol. Biochem. 16, 169–175. doi: 10.1016/0038-0717(84)90108-1
Bunemann, E. K., Keller, B., Hoop, D., Jud, K., Boivin, P., and Frossard, E. (2013). Increased availability of phosphorus after drying and rewetting of a grassland soil: processes and plant use. Plant Soil 370, 511–526. doi: 10.1007/s11104-013-1651-y
Butterly, C., Bünemann, E., McNeill, A., Baldock, J., and Marschner, P. (2009). Carbon pulses but not phosphorus pulses are related to decreases in microbial biomass during repeated drying and rewetting of soils. Soil Biol. Biochem. 41, 1406–1416. doi: 10.1016/j.soilbio.2009.03.018
Carter, M. R., and Gregorich, E. G. (Eds.) (2008). Soil Sampling and Methods of Analysis. Boca Raton, FL:CRC Press. doi: 10.1201/9781420005271
Chapman, H. D. (1965). “Cation-exchange capacity,” in Methods of Soil Analysis, Part 2, Chemical and Microbiological Properties, ed C. A. Black (Madison, WI: ASA, 891–901.
Chen, H., Lai, L., Zhao, X., Li, G., and Lin, Q. (2016). Soil microbial biomass carbon and phosphorus as affected by frequent drying–rewetting. Soil Res. 54, 321–327. doi: 10.1071/SR14299
Dieter, D., Herzog, C., and Hupfer, M. (2015). Effects of drying on phosphorus uptake in re-flooded lake sediments. Environ. Sci. Pollut. Res. 22, 17065–17081. doi: 10.1007/s11356-015-4904-x
Dinh, M., Guhr, A., Weig, A. R., and Matzner, E. (2018). Drying and rewetting of forest floors: dynamics of soluble phosphorus, microbial biomass-phosphorus, and the composition of microbial communities. Biol. Fertil. Soils 54, 761–768. doi: 10.1007/s00374-018-1300-y
Dinh, M. V., Guhr, A., Spohn, M., and Matzner, E. (2017). Release of phosphorus from soil bacterial and fungal biomass following drying/rewetting. Soil Biol. Biochem. 110, 1–7. doi: 10.1016/j.soilbio.2017.02.014
Forber, K. J., Ockenden, M. C., Wearing, C., Hollaway, M. J., Falloon, P. D., Kahana, R., et al. (2017). Determining the effect of drying time on phosphorus solubilization from three agricultural soils under climate change scenarios. J. Environ. Qual. 46, 1131–1136. doi: 10.2134/jeq2017.04.0144
Gordon, H., Haygarth, P. M., and Bardgett, R. D. (2008). Drying and rewetting effects on soil microbial community composition and nutrient leaching. Soil Biol. Biochem. 40, 302–311. doi: 10.1016/j.soilbio.2007.08.008
Harrod, T., and Hogan, D. (2008). The Soils of North Wyke and Rowden. Soil Survey of England and Wales. Okehampton: Rothamsted Research.
Homberg, A., and Matzner, E. (2018). Effects of drying and rewetting on soluble phosphorus and nitrogen in forest floors: an experiment with undisturbed columns. J. Plant Nutr. Soil Sci. 181, 177–184. doi: 10.1002/jpln.201700380
Hooda, P. S., Moynagh, M., Svoboda, I., Edwards, A., Anderson, H., and Sym, G. (1999). Phosphorus loss in drainflow from intensively managed grassland soils. J. Environ. Qual. 28, 1235–1242. doi: 10.2134/jeq1999.00472425002800040026x
IPCC (2014). Climate Change 2014: Synthesis Report: Contribution of Working Groups I, II and III to the Fifth Assessment Report of the Intergovernmental Panel on Climate Change [Core Writing Team], eds R.K. Pachauri and L.A. Meyer. Geneva: IPCC, 151.
Kaiser, M., Kleber, M., and Berhe, A. A. (2015). How air-drying and rewetting modify soil organic matter characteristics: an assessment to improve data interpretation and inference. Soil Biol. Biochem. 80, 324–340. doi: 10.1016/j.soilbio.2014.10.018
Kieft, T. L., Soroker, E., and Firestone, M. K. (1987). Microbial biomass response to a rapid increase in water potential when dry soil is wetted. Soil Biol. Biochem.19, 119–126. doi: 10.1016/0038-0717(87)90070-8
Koopmans, G. F., Chardon, W. J., Dekker, P. H. M., Römkens, P. F. A. M., and Schoumans, O. F. (2006). Comparing different extraction methods for estimating phosphorus solubility in various soil types. Soil Sci. 171, 103–116. doi: 10.1097/01.ss.0000187361.00600.d6
Meisner, A., Rousk, J., and Bååth, E. (2015). Prolonged drought changes the bacterial growth response to rewetting. Soil Biol. Biochem. 88, 314–322. doi: 10.1016/j.soilbio.2015.06.002
Mondini, C., Contin, M., Leita, L., and De Nobili, M. (2002). Response of microbial biomass to air-drying and rewetting in soils and compost. Geoderma 105, 111–124. doi: 10.1016/S0016-7061(01)00095-7
Murphy, J., and Riley, J. P. (1962). A modified single solution method for the determination of phosphate in natural waters. Anal. Chim. Acta 27, 31–36. doi: 10.1016/S0003-2670(00)88444-5
Olsen, S. R. (1954). Estimation of Available Phosphorus in Soils by Extraction With Sodium Bicarbonate. Washington, DC: United States Department of Agriculture.
Peltovuori, T., and Soinne, H. (2005). Phosphorus solubility and sorption in frozen, air-dried and field-moist soil. Euro J Soil Sci. 56, 821–826. doi: 10.1111/j.1365-2389.2005.00726.x
Pezzolla, D., Cardenas, L. M., Mian, I. A., Carswell, A., Donovan, N., Dhanoa, M. S., et al. (2019). Responses of carbon, nitrogen and phosphorus to two consecutive drying–rewetting cycles in soils. J. Plant Nutr. Soil Sci. 182, 217–228. doi: 10.1002/jpln.201800082
Rahman, M. M., Hicks, L. C., Verheyen, K., Rousk, J., and Carnol, M. (2018). Effects of drought legacy and tree species admixing on bacterial growth and respiration in a young forest soil upon drying and rewetting. Soil Biol. Biochem. 127, 148–155. doi: 10.1016/j.soilbio.2018.09.026
Sardans, J., and Penuelas, J. (2007). Drought changes phosphorus and potassium accumulation patterns in an evergreen Mediterranean forest. Funct. Ecol. 21, 191–201. doi: 10.1111/j.1365-2435.2007.01247.x
Schimel, J. P., Gulledge, J. M., Clein-Curley, J. S., Lindstrom, J. E., and Braddock, J. F. (1999). Moisture effects on microbial activity and community structure in decomposing birch litter in the Alaskan taiga. Soil Biol. Biochem. 31, 831–838. doi: 10.1016/S0038-0717(98)00182-5
Schonbrunner, I. M., Preiner, S., and Hein, T. (2012). Impact of drying and re-flooding of sediment on phosphorus dynamics of river-floodplain systems. Sci. Total Environ. 432, 329–337. doi: 10.1016/j.scitotenv.2012.06.025
Soinne, H., Raty, M., and Hartikainen, H. (2010). Effect of air-drying on phosphorus fractions in clay soil. J. Plant Nutr. Soil Sci. 173, 332–336. doi: 10.1002/jpln.200900225
Styles, D., and Coxon, C. (2006). Laboratory drying of organic-matter rich soils: phosphorus solubility effects, influence of soil characteristics, and consequences for environmental interpretation. Geoderma 136, 120–135. doi: 10.1016/j.geoderma.2006.03.017
Sun, D., Bi, Q., Xu, H., Li, K., Liu, X., Zhu, J., et al. (2017). Degree of short-term drying before rewetting regulates the bicarbonate-extractable and enzymatically hydrolyzable soil phosphorus fractions. Geoderma 305, 136–143. doi: 10.1016/j.geoderma.2017.05.040
Turner, B. L., and Haygarth, P. M. (2001). Biogeochemistry: phosphorus solubilization in rewetted soils. Nature 411, 258. doi: 10.1038/35077146
Turner, B. L., and Romero, T. E. (2010). Stability of hydrolytic enzyme activity and microbial phosphorus during storage of tropical rain forest soils. Soil Biol. Biochem. 42, 459–465. doi: 10.1016/j.soilbio.2009.11.029
Voroney, R. P. (2007). The Soil Habitat, Soil Microbiology, Ecology and Biochemistry, 4th Edn. London, UK; Oxford: Academic Press; Elsevier. doi: 10.1016/B978-0-08-047514-1.50006-8
Walkley, A., and Black, I. A. (1934). An examination of the Degtjareff method for determining soil organic matter, and a proposed modification of the chromic acid titration method. Soil Sci. 37, 29–38. doi: 10.1097/00010694-193401000-00003
Wang, Y., Jensen, C. R., and Liu, F. (2017). Nutritional responses to soil drying and rewetting cycles under partial root-zone drying irrigation. Agric. Water Manage. 179, 254–259. doi: 10.1016/j.agwat.2016.04.015
Wu, J., and Brookes, P. (2005). The proportional mineralisation of microbial biomass and organic matter caused by air-drying and rewetting of a grassland soil. Soil Biol. Biochem. 37, 507–515. doi: 10.1016/j.soilbio.2004.07.043
Xiang, S., Doyle, A., Holden, P. A., and Schimel, J. P. (2008). Drying and rewetting effects on C and N mineralization and microbial activity in surface and subsurface California grassland soils. Soil Biol. Biochem. 40, 2281–2289. doi: 10.1016/j.soilbio.2008.05.004
Yevdokimov, I., Larionova, A., and Blagodatskaya, E. (2016). Microbial immobilisation of phosphorus in soils exposed to drying-rewetting and freeze-thawing cycles. Biol. Fertil. Soils 52, 685–696. doi: 10.1007/s00374-016-1112-x
Zhang, Y., and Marschner, P. (2018). Respiration, microbial biomass and nutrient availability are influenced by previous and current soil water content in plant residue amended soil. J. Soil Sci. Plant Nutr. 18, 173–187. doi: 10.4067/S0718-95162018005000703
Keywords: microbial biomass, soil, climate change, drying-rewetting, phosphorus leaching
Citation: Khan SU, Hooda PS, Blackwell MSA and Busquets R (2019) Microbial Biomass Responses to Soil Drying-Rewetting and Phosphorus Leaching. Front. Environ. Sci. 7:133. doi: 10.3389/fenvs.2019.00133
Received: 23 May 2019; Accepted: 28 August 2019;
Published: 12 September 2019.
Edited by:
Balwant Singh, University of Sydney, AustraliaReviewed by:
Nabeel Khan Niazi, University of Agriculture, PakistanHannes Schmidt, University of Vienna, Austria
Copyright © 2019 Khan, Hooda, Blackwell and Busquets. This is an open-access article distributed under the terms of the Creative Commons Attribution License (CC BY). The use, distribution or reproduction in other forums is permitted, provided the original author(s) and the copyright owner(s) are credited and that the original publication in this journal is cited, in accordance with accepted academic practice. No use, distribution or reproduction is permitted which does not comply with these terms.
*Correspondence: Peter S. Hooda, p.hooda@kingston.ac.uk