- 1Department of Terrestrial Ecology, Netherlands Institute of Ecology, Wageningen, Netherlands
- 2School of Earth and Environmental Sciences, The University of Manchester, Manchester, United Kingdom
- 3Department of Forest Ecology and Management, Swedish University of Agricultural Sciences, Umeå, Sweden
- 4Department of Ecosystem and Landscape Dynamics (IBED-ELD), Institute of Biodiversity and Ecosystem Dynamics, University of Amsterdam, Amsterdam, Netherlands
Most studies focusing on plant-soil feedbacks (PSFs) have considered direct interactions between plants, abiotic conditions (e. g., soil nutrients) and rhizosphere communities (e.g., pathogens, mutualists). However, few studies have addressed the role of indirect interactions mediated by plant litter inputs. This is problematic because it has left a major gap in our understanding of PSFs in natural ecosystems, where plant litter is a key component of feedback effects. Here, we propose a new conceptual framework that integrates rhizosphere- and litter-mediated PSF effects. Our framework provides insights into the relative contribution of direct effects mediated by interactions between plants and soil rhizosphere organisms, and indirect effects between plants and decomposer organisms mediated by plant root and shoot litter. We distinguish between three pathways through which senesced root and shoot litter may influence PSFs. Specifically, we examine: (1) physical effects of litter (layer) traits on seed germination, soil structure, and plant growth; (2) chemical effects of litter on concentrations of soil nutrients and secondary metabolites (e.g., allelopathic chemicals); and (3) biotic effects of saprotrophic soil communities that can perform different functional roles in the soil food web, or that may have specialized interactions with litter types, thereby altering soil nutrient cycling. We assess the role of litter in PSF effects via physical, chemical and biotic pathways to address how litter-mediated feedbacks may play out relative to, and in interaction with, feedbacks mediated through the plant rhizosphere. We also present one of the first experimental studies to show the occurrence and species-specificity of litter-mediated feedbacks and we identify critical research gaps. By formally incorporating the plant-litter feedback pathway into PSF experiments, we will further our understanding of PSFs under natural conditions.
Introduction
Plants modify their biotic and abiotic soil environment, which in turn has a major influence on subsequent plant growth, also referred to as plant-soil feedback (PSF) (Bever et al., 1997; Wardle et al., 2004). Plant-soil feedbacks are key drivers of plant physiology, growth and community composition (Kulmatiski et al., 2008; van der Putten et al., 2013; Teste et al., 2017) and thereby underlie ecosystem functioning (Bennett et al., 2017; Mariotte et al., 2018). Therefore, managing PSFs can be of importance for improving sustainability in agro-ecosystems (Mariotte et al., 2018), for restoration of natural ecosystems (Kardol and Wardle, 2010 review TREE, Wubs et al., 2016) and for enhancing the resilience of ecosystems under global change and species invasions (van der Putten et al., 2016). For example, exploiting positive PSF effects may allow us to improve yield and reduce the use of artificial fertilizer and pesticides in agricultural fields (Mariotte et al., 2018; Veen et al., 2019). However, to be able to manage PSFs it is crucial to unravel how PSFs operate.
It has been long recognized that PSFs can be mediated via direct interactions between plants and rhizosphere communities, as well as indirect interactions between plants and decomposer communities driven by litter inputs (Figure 1) (Wardle et al., 2004; Ehrenfeld et al., 2005; Kardol et al., 2015). However, most studies focusing on PSFs have considered direct interactions (e.g., pathogens, mutualists), while largely neglecting the role of plant litter and decomposer communities (Elgersma et al., 2012; van der Putten et al., 2016). In natural ecosystems, litter may leave physical, chemical and biotic legacies in the soil that have a strong impact on soil functioning and plant growth (Ehrenfeld et al., 2005; Elgersma et al., 2012). In addition, litter-mediated PSFs may modify rhizosphere-mediated PSFs (Kardol et al., 2015; Ke et al., 2015; Zhang et al., 2016), for example, because saprotrophic soil organisms may suppress plant pathogens as a result of competition for space and nutrients (Rodríguez et al., 2016). Therefore, to understand the importance of PSFs under natural conditions, it will be essential to unravel the role of plant litter and decomposer communities in PSFs (Ke et al., 2015; Veen et al., 2018).
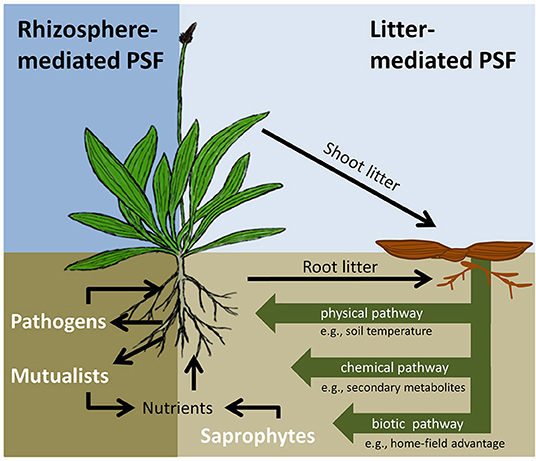
Figure 1. Overview of the plant-soil feedback (PSF) framework integrating rhizosphere- and litter-mediated PSF effects. Rhizosphere-mediated PSFs will be driven by direct interactions between living plant roots and pathogens or mutualists. Litter-mediated PSFs will run via physical (e.g., litter layer thickness; litter physical traits), chemical (e.g., soil nutrient availability; secondary metabolites; allelopathy) or biotic (e.g., soil community composition; biotic interactions; home-field advantage effects) pathways. Litter-mediated PSFs via the physical pathway may be driven by species-specific impacts of e.g., litter layer thickness or light availability underneath litter layers on seedling germination or plant growth (Facelli and Pickett, 1991a). Litter-mediated effects via the chemical pathway may be driven by plant growth responses to the release of primary of secondary chemicals from leaf litter during decomposition (Facelli and Pickett, 1991a). Finally, litter-mediated PSFs via the biotic pathway may be driven by local variation in the composition and activity of decomposer communities and hence the rates at which they control the recycling of plant litter. Rhizosphere-mediated and litter-mediated PSFs interact, for example, through direct competition for space and nutrients between pathogens, mutualists and saprotrophs, the immobilization of nutrients released from litter by biota in the rhizosphere, or responses of pathogens and mutualists to physical and chemical changes in the soil induced by litter. Also, it is important to note that, physical, chemical, and biotic pathways of litter-mediated PSFs interact, for example, because plant-induced changes in the saprotrophic community will affect the rate at which nutrients and chemical compounds from litter are released into the soil. Interactions between the different pathways of litter-mediated PSFs are not specifically depicted in the figure.
Plant-soil feedback effects mediated via the biotic community in the rhizosphere are known to be driven by species-specific relationships between plant species and organisms inhabiting the rhizosphere. Sugars and plant signaling compounds excreted from plant roots attract or repel soil biota inhabiting the rhizosphere (Philippot et al., 2013; el Zahar Haichar et al., 2014; Kaiser et al., 2015), thus creating a plant-specific rhizosphere microbiome (Raaijmakers et al., 2009). Depending on the balance between mutualists and pathogens, this community will directly stimulate or inhibit plant growth (Raaijmakers et al., 2009; van der Putten et al., 2016). Importantly, such species-specific PSFs may also be mediated via plant litter pathways (Elgersma et al., 2012; Mazzoleni et al., 2015). For example, we showed in a greenhouse experiment that the biomass of the grass Festuca rubra was higher on soils previously incubated with conspecific litter (litter-mediated feedback), while biomass of the tree Betula pendula was not affected by litter, but was increased on soils where conspecific plants were grown previously (rhizosphere-mediated feedback) (Box 1; Figures 2A,B). Recent work has indicated multiple mechanisms that may explain such specific litter-mediated PSFs. For example, decomposer communities may have a strong affinity for plant litter types from the plant with which they are associated (Austin et al., 2014; Palozzi and Lindo, 2018), resulting in accelerated litter breakdown (Freschet et al., 2012a; Veen et al., 2015) and plant-specific patterns of nutrient release (Perez et al., 2013). In addition, the release of DNA and toxic compounds from decomposing plant litter can inhibit the growth of the plant from which the litter originates (Mazzoleni et al., 2015; Cartenı̀ et al., 2016). How the negative feedback of self-DNA works is not fully understood, but it is hypothesized that plants have mechanisms to recognize their own DNA (Mazzoleni et al., 2015; Cartenı̀ et al., 2016). Understanding the specificity of litter-mediated PSFs is crucial to elucidate how PSFs control plant growth and coexistence in natural systems (Kardol et al., 2015; van der Putten et al., 2016).
Box 1. Description of set up and results of greenhouse experiment. The aim of this experiment is to disentangle rhizosphere- and litter-mediated plant-soil feedback effects for the tree Betula pendula and the grass Festuca rubra.
To test the role of plant litter inputs in driving plant-soil feedback effects, we set up a reciprocal litter transplant experiment in the greenhouse with soils and leaf litters from two plant species, i.e., the tree Betula pendula and the grass Festuca rubra. Soils and litters were collected from six replicate ex-arable grasslands and adjacent forests along a well-established chronosequence, in the nature reserve “the Veluwe” in the Netherlands; located between Ede (52°04′20″N, 5°44′12″) and Wolfheze (52°00′77″ N, 5°48′58″) (Kardol et al., 2006; Morriën et al., 2017; Veen et al., 2018). At each site, soil samples and associated plant litter were collected in October 2014 underneath Betula and Festuca plants (see Veen et al., 2018 for details on sample collection). For each site, soil samples were sieved over 10 mm to remove large roots and stones. Litter subsamples were also homogenized per site, air-dried, cut into 1-cm fragments and sterilized using gamma-irradiation (25 K Gray). We used the soils and litters to set up a two-phase experiment, with a litter incubation phase where we created “litter-specific soils” and a feedback phase where we tested the biomass response of Betula and Festuca seedlings to the incubated soils.
For the litter incubation phase, we used the soils to fill two 0.5-L pots per site, with each pot receiving 160 g of soil on a dry weight basis. Then, 2.0 g of litter was added to each pot following a full-factorial design. This resulted in two soil sources (Betula, Festuca) × 2 litter types (Betula, Festuca) × 6 replicates = 24 pots (Figure 2C, incubation phase). After 3 and 6 months of litter incubation, we added an additional 2.0 g of fresh litter to each of the pots, resulting in a 9-month litter incubation period in total. The amount of litter used was representative of the amount of natural litter fall in temperate grasslands (Penuelas et al., 2007). During incubation, pots were kept at 18°C and 65% water holding capacity (WHC). For the feedback phase, we collected two 32-g subsamples of soil (dry weight basis) from each pot, which we inoculated into two pots with 128 g of sterilized soil (dry weight basis) to test the biomass response of Betula and Festuca, respectively, resulting in 48 pots (Figure 2C, feedback phase). Soil for sterilization was collected from one of the ex-arable fields (van der Putten et al., 2000). We used this approach, which is common in plant-soil feedback research, to reduce the effect of abiotic differences between soil sources on plant growth in the feedback phase (Brinkman et al., 2010). During the feedback phase pots received 2.0 g of the same litter type as what they had received during the incubation phase and were put in the greenhouse for 5 months before growing Betula and Festuca seedlings. Seedlings were grown for 6 weeks according to a full-factorial design with each plant species growing on each combination of soil and litter sources. During the feedback phase pots were kept at day/night temperatures of 21/16°C, relative humidity of 60% and a day length of 16 h and 70% soil WHC. At the end of the experiment, we determined plant shoot and root biomass. Data were analyzed in R Core Team (2013) using general linear mixed models (Bates and Maechler, 2009) with soil source, litter source and plant species as fixed factors and replicate site as a random factor, followed by Tukey HSD tests. We have checked for a normal distribution of the residuals and homogeneity of variances before proceeding with our analyses.
Both shoot (F1,39 = 75.70, P < 0.001) and root (F1,39 = 183.03, P < 0.001) biomass were higher for Festuca than for Betula seedlings (Figures 2A,B). In addition, there was a main effect of soil source, indicating that shoot (F1,39 = 11.03, P = 0.002) and root (F1,39 = 16.65, P < 0.001) biomass were higher in Betula soils than in Festuca soils (Figures 2A,B). Finally, the interaction between litter source and plant species affected root biomass (F1,39 = 13.04, P < 0.001) and tended to affect shoot biomass (F1,39 = 3.64, P = 0.064), indicating that Festuca plants had more root biomass on soils incubated with Festuca litter, while there was no effect of litter incubation treatment on Betula (Figures 2A,B). Our findings show that litter inputs can have species-specific feedback effects to plant growth. As a result, litter feedbacks have the potential to contribute to or modify rhizosphere-mediated feedbacks. From this experiment we cannot disentangle to what extent feedback effects were mediated via litter-induced changes in soil chemistry or via changes in the composition and functioning of the saprotrophic community. However, our findings indicate that we need to integrate litter-feedback effects into the framework of plant-soil feedbacks.
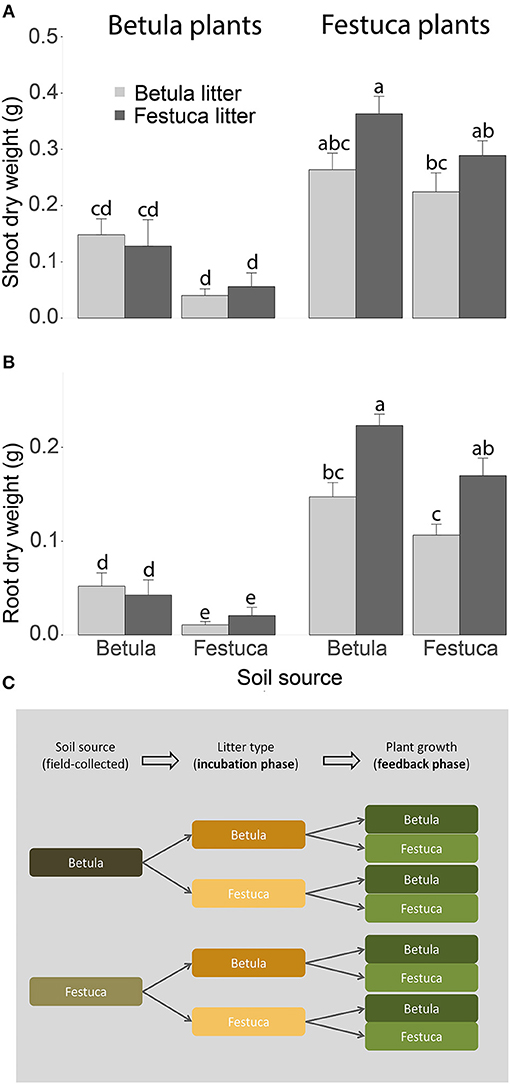
Figure 2. Shoot dry weight (A) and root dry weight (B) of Betula pendula and Festuca rubra plants in response to soil source (the plant type underneath which the soil was collected in the field) and litter incubation treatments (the litter type that was incubated in the soil during the experiment). Different letters above the bars indicate statistical differences at P > 0.05 indicated by Tukey HSD post-hoc tests. (C) Schematic overview of the experimental set up. Soil samples used in this experiment were collected in the field underneath Betula and Festuca plants (left column). Then soil samples were incubated under controlled conditions with plant litter from both plant species using a full-factorial reciprocal transplant design (middle column). Finally, we grew seedlings of both plant species on each soil in a full-factorial design (right column). This resulted in 8 pots for each replicate (n = 6) where each plant species (Betula and Festuca) was grown on each type of litter incubation (Betula and Festuca) and each type of field soil (Betula and Festuca).
Here, we propose a novel framework that integrates rhizosphere- and litter-mediated PSF effects (Figure 1). In this paper we will focus on the role of litter-mediated PSF effects as there are already detailed reviews and meta-analyses focussing on rhizosphere-mediated PSFs (e.g., Kulmatiski et al., 2008; van der Putten et al., 2013). We explore three main pathways via which plant litter can drive PSFs: physical, chemical and biological (Ehrenfeld et al., 2005). For each of these pathways, we describe how they may contribute to explain PSF effects. It is important to note that even though we separate the litter-mediated PSFs via the three different pathways, under natural conditions, effects are often hard to disentangle as PSF effects will be mediated by interactions between the pathways. In addition, we identify ways forward in PSF research to increasing our understanding of interactions between litter-mediated and rhizosphere-mediated PSFs, which will advance our knowledge of PSFs in natural ecosystems.
Physical Pathways
Physical pathways have received little attention in research on litter-mediated PSFs, but it has long been known that plant litter has strong effects on the physical soil environment (Facelli and Pickett, 1991a), both in natural (Facelli and Pickett, 1991b) and agricultural ecosystems, i.e., via crop residues (Teasdale et al., 1991; Walia and Dick, 2018). The effects of plant litter on the physical soil environment have strong potential to feed back to plant performance through effects on seed germination, seedling establishment, and initial plant growth (Olson and Wallander, 2002; Asplund et al., 2018).
A layer of leaf litter may improve the microclimatic conditions for seed germination through moisture retention and buffering against temperature extremes. In laboratory studies, it has been shown that under conditions of strong desiccation, acorns covered by a layer of leaves suffered lower water losses and, therefore, had higher rates of germination (García et al., 2002). However, under field conditions the evidence for improved germination rates in the presence of a litter layer is mixed (e.g., Barrett, 1931; Shaw, 1968; García et al., 2002; Kremer et al., 2019), probably because the extent to which the litter layer improves germination depends on ambient moisture conditions. Further, soil temperature is generally higher under a layer of plant litter (Sharratt, 2002), because the build-up of litter on the ground surface affects the transfer of heat between the soil and the atmosphere, which in turn can lead to increased seed germination rates (Paul et al., 2004). In addition, plant litter may physically protect seeds against predation (García et al., 2002). In contrast, the litter layer can also have negative impacts on seed germination and plant growth, because it can reduce the amount of light reaching the soil surface (Facelli and Pickett, 1991a) and it may act as a physical barrier to seedling emergence (Barrett, 1931). Finally, incorporation of litter into the soil matrix may modify soil structure, which may modify plant growth responses. Although most evidence for physical effects of litter on plant performance comes from studies on leaf litter, there may also be important effects mediated via root litter (Bardgett et al., 2014), but these remain to be tested. As root litter is already incorporated into the soil matrix, it may not form a litter layer and therefore may have different physical PSF effects than leaf litter.
Litter-mediated PSFs via the physical pathway have not been studied extensively, but may be plant species-specific. The retention of soil moisture underneath leaf litter layers is strongly related to the traits of the plant litter species, for example specific leaf area (SLA), three-dimensionality of the litter, or tensile strength (Swift et al., 1979; Makkonen et al., 2013). Also, plant litter may have species-specific effects on soil water repellency, depending on the presence of organic hydrophobic compounds (Cesarano et al., 2016). Similarly, light extinction curves in litter layers differ between litter species (Facelli and Pickett, 1991b). The empirical support is scarce, but these litter-specific effects on environmental conditions strongly suggest that litter-mediated PSFs via the physical pathway have species-specific effects on seed germination and seedling growth.
Plant responses to litter-induced changes in environmental conditions via the physical pathway may also be species-specific (e.g., Olson and Wallander, 2002). In many empirical experiments it remains hard to untangle physical litter PSF effects, from chemical or biological effects. However, measurements on e.g., light penetration or mechanical barriers by litter in pot and field experiments indicate that physical litter-mediated PSF effects may play a role in driving species-specific plant responses. For example, using a controlled pot experiment testing effects of litter on seedling emergence, Donath and Eckstein (2008) found significant interactions between litter type and plant species origin at least partly driven through mechanical effects. Woodland species produced more biomass in presence of oak litter than in presence of grass litter, indicating a positive litter-mediated PSF effect which was explained by oak litter consisting of individual leaves, while grass litter typically forms dense interwoven mats which might be difficult to penetrate and could inhibit shoot emergence. For mechanical inhibition, seed size and seed position can be important in determining the strength of physical litter feedbacks (Donath and Eckstein, 2010). Zhang et al. (2017) found that a moderate amount of litter was beneficial for seedling emergence of small-seeded species, while large-seeded species were more tolerant to high amounts of litter input than small-seeded species. Also, litter accumulation by an exotic plant species Avena fatua reduced the germination of small-sized seeds of native species via increased litter depth and light reduction, thereby facilitating invasion of A. fatua in Californian grasslands (Mariotte et al., 2017). The impact of light reduction suggests that at least physical pathways may play role, but to what extent other pathways also play a role remains to be tested.
Together, these plant-specific impacts on and responses to the litter layer show that the creation of physical barriers and alteration of the abiotic environment by plant litter may play a key role in PSF in natural ecosystems. Understanding how shoot and root litter traits drive such physical litter-mediated PSFs may offer a promising avenue for further exploring the role of litter in PSF (Bardgett et al., 2014; Cortois et al., 2016). However, only few empirical studies have specifically focused on the role of physical effects in driving PSF. This is experimentally challenging, but may provide insights in plant growth responses to litter inputs. It is important to mention that the level of specificity of physical litter-mediated PSF may be lower than for rhizosphere-mediated PSF, because the strength and direction of physical litter feedbacks may be largely determined by generic leaf and seeds traits; however, this warrants further investigation.
Chemical Pathways
During decomposition a wide range of chemical compounds is released from plant litter which can be beneficial or detrimental to subsequent generations of plants (Facelli and Pickett, 1991a). Litter-mediated PSFs via the chemical pathway can be driven by the liberation of plant nutrients, secondary metabolites or DNA from decomposing litter (Facelli and Pickett, 1991a; Mazzoleni et al., 2015). Soil nutrients can be made available through rapid decomposition of nutrient-rich litter. This will increase plant nutrient availability in the next generation. In contrast, litter with a high concentration of structural carbohydrates, such as cellulose, decomposes slower and may produce less positive or even negative PSFs (Vahdat et al., 2011). Although litter-mediated PSF via nutrients may be less species-specific than PSFs mediated via other chemicals, plant species responses to litter-mediated changes in soil nutrient cycling often differ between plant growth strategies. Generally, fast-growing, exploitative plant species will benefit most from positive litter-mediated PSFs via nutrient availability, while plant species with conservative resource-use strategies may be less hampered by slow recycling of nutrients (Wardle et al., 2004). Also, positive litter-mediated PSFs may favor the invasion of exotic plants, that are often better competitors for nutrients released from plant litter (Eppinga et al., 2011). However, generalizing litter-mediated PSFs through nutrients may be complicated because of confounding effects of other chemical compounds or biotic interactions, which may result in unexpected effects on future generations of plants (Hobbie, 2015).
Plant litter also contains a range of secondary metabolites, including alkaloids, phenolic compounds and terpenes, which are often used as a defense mechanism against herbivores and pathogens and therefore play a key role in plant-microbe and plant-herbivore interactions (Chomel et al., 2016). These secondary metabolites are important for determining decomposition rates. For example, tannins may slow rates of litter decomposition (Hättenschwiler and Vitousek, 2000) and phenolic compounds can delay the colonization of litter by decomposer organisms (Ormeno et al., 2006; Chomel et al., 2014). Yet, it is now increasingly acknowledged that some complex compounds, such as lignin, can be degraded more quickly than previously assumed (Lehmann and Kleber, 2015), but that this may depend on the presence of specialized microbial communities, such as certain lignin-degrading fungi (van der Wal et al., 2015). As a result, secondary metabolites can contribute to litter-mediated PSFs via impeding nutrient cycling (Wardle et al., 2012). Secondary metabolites can also have direct impacts on plant growth when released into the soil, and their impact may strongly depend on the residence time in the soil (Chomel et al., 2016). Many chemical compounds that are present in the living plant, such as alkaloids, are quickly metabolized in litter and are thought to have limited effects on soil processes and plant responses (Siegrist et al., 2010). However, other chemicals liberated from plant litter can persist in the soil after senescence and may inhibit the growth or germination of neighboring and next-generation plants (Bonanomi et al., 2011), a phenomenon referred to as allelopathy (Muller, 1966). For example, the growth of tree seedlings may be inhibited by phenol-rich litter (Hättenschwiler and Vitousek, 2000). Also, flavonoids, which are known to play a role in attracting beneficial microbes, such as Rhizobia, may remain in plant tissue after senescence and affect plant growth by scavenging free radicals and improving stress tolerance (Barazani and Friedman, 2001). In addition to secondary metabolites, DNA released from decomposing material may also hamper plant growth of next generations of plants, and this negative feedback is specifically targeted toward congeneric plant species (Mazzoleni et al., 2015).
Litter-mediated PSFs via chemical compounds may strongly differ between above- and belowground plant organs. Although decomposition rates of shoots, stems and roots broadly correlate across large-scale fertility gradients, at the level of sites or individual plant species decomposition rates may differ between plant organs (Hobbie et al., 2010). This means that above- and belowground plant organs, as well as different types of roots, may play a different role in ecosystem processes (Freschet et al., 2012b). For example, leaf litter decomposes often more rapidly than root litter (Freschet et al., 2013), and finer roots, often richer in nitrogen (Pregitzer et al., 1997), faster than coarser roots. Although this may affect litter-mediated PSF, only a few studies have focused on the impacts of root litter on PSF (e.g., Mazzoleni et al., 2015; Zhang et al., 2016), and none of them compare how PSFs induced by root and shoot litter differ. Yet, root decomposition is likely to play a key role in subsurface soil layers and in ecosystems, such as tundra and grasslands, where half to three quarters of plant biomass is produced belowground as roots (Poorter et al., 2012). Therefore, it is essential to start disentangling the role of root and shoot litter driving chemical litter-mediated PSF effects.
Biotic Pathways
Local variation in decomposer community composition and activity drives variation in decomposition rates (Hattenschwiler and Gasser, 2005; Vos et al., 2011; Bradford et al., 2014, 2017). Variation in decomposer composition may be tightly linked to plant composition (Bezemer et al., 2010), indicating that decomposer composition and activity may have an important role in driving litter-mediated PSFs. The litter-fragmenting community (or detritivores), including earthworms, millipedes, myriapods, diplopods, and various insect larvae, transforms a large part of plant litter into feces, thereby increasing the surface area for microbial decomposition, which often results in accelerated litter breakdown (Hattenschwiler and Gasser, 2005; David, 2014; Joly et al., 2018). The impact of litter shredders on decomposition may be strongly affected by decomposers actively selecting certain litter types or compounds. For example, earthworms often prefer palatable residues, such as litter with low carbon:nitrogen ratios and tend to avoid ingestion of root litter (Curry and Schmidt, 2007; Vidal et al., 2017). In addition, the functioning of the gut microbiome of litter shredders, which is likely to be picked up from the soil (Hannula et al., 2019), may affect decomposition processes. Saprotrophic fungi and bacteria in the soil are involved in the mineralization of nutrients into inorganic forms that can be taken up by plants. How this affects plant species may be strongly determined by the stoichiometric constraints of both plants and the microorganisms involved in soil nutrient cycling (Capek et al., 2018). Depending on how plants drive shifts in the composition and activity of detritivores, saprotrophs, and gut microbes, variation in the soil community will contribute to litter-mediated PSFs by altering soil nutrient availability (Joly et al., 2018) and liberating secondary metabolites from plant litter. Also, interactions between decomposers and other soil organisms (e.g., predators in the soil) may have a large impact on litter-mediated PSFs. For example, consumption of detritivores and microbial decomposers by predators in the soil could inhibit decomposition (Liu et al., 2014) and thereby short-circuit litter-mediated PSFs.
Litter-mediated PSFs may also operate via home-field advantage effects, which is the process that litter decomposition is accelerated near the plant where the litter originates from relative to litter decomposition further away from that plant (see Gholz et al., 2000; Ayres et al., 2009; Veen et al., 2015; Palozzi and Lindo, 2018). The hypothesis is that home-field advantage effects are driven by species-specific associations between plants and decomposer communities (Freschet et al., 2012a; Austin et al., 2014). Recent experimental work showed that different litter types can indeed harbor specific litter microbiomes (Keiser et al., 2011; Lin et al., 2019) and that variation in abundant litter fungal groups can contribute to home-field advantage effects (Veen et al., 2019). This results in plant-specific patterns in nutrient release (Perez et al., 2013) and, hence, may contribute to litter-mediated PSFs. In general, home-field advantage effects may be expected to favor the plants where the litter originated from Zhang et al. (2016), but nutrients released from litter may also be taken up by neighboring plant species (Hood et al., 2000; Collins et al., 2007). Therefore, the extent of plant specificity in litter-induced feedbacks via home-field advantage remains to be tested (van der Putten et al., 2016; Zhang et al., 2016).
Most studies focus on shoot litter decomposition, but decomposition of root litter may be equally or more important in driving biotic litter-mediated PSFs (Li et al., 2015; Zhang et al., 2016), because it takes place in or near the rhizosphere of plants. As a result, saprotrophic communities involved in root litter breakdown closely interact with mutualists- and pathogens located in the rhizosphere, via competition for carbon and nutrients (Rousk and Bååth, 2011; Boddy, 2016; Money, 2016; Sokol and Bradford, 2019). The extent of these interactions depends on the availability of easily available sugars (Rousk and Bååth, 2011; Ballhausen and de Boer, 2016), the stoichiometry of plant litter (e.g., how much nitrogen is available) (Zhang and Elser, 2017), the chemical composition of the litter (Gartner et al., 2012) and accessibility of other soil organic material to saprotrophs (Rillig and Mummey, 2006). In turn, the mutualistic, pathogenic, and saprotrophic components of the microbial community are consumed by organisms, such as collembola that graze on fungal mycelium (Scheu and Schulz, 1996) or protists that feed on unicellular bacteria and fungi (Radosa et al., 2019). In addition, saprotrophs can compete for root exudates with plant parasites or ectomycorrhiza in the rhizosphere. As a result, both rhizosphere- and litter-mediated PSFs can be controlled by similar trophic top-down and bottom-up factors (De Long et al., 2019). It is therefore important not to regard them as separate units, but as intertwined operating pathways imbedded in the same food-web context.
The Way Forward
Here, we demonstrated how root and shoot litter can affect PSFs through physical, chemical and biotic pathways. Although an emerging body of literature supports the potential importance of litter-mediated PSFs, many critical knowledge gaps remain which require further investigation. Here we identify five important avenues for further research into the role of litter-mediated PSFs:
(1) Disentangle the interactions between litter-mediated vs. rhizosphere-mediated PSFs. We already know that rhizosphere pathogens or symbionts play a strong role in mediating PSFs (Bennett et al., 2017; Semchenko et al., 2018) and litter-mediated PSFs are gaining more attention (Freschet et al., 2013; Hobbie, 2015; Zhang et al., 2016; Manrubia et al., 2019), but interactions and their relative hierarchy of importance needs to be elucidated. For example, modeling work showed that rhizosphere-mediated PSFs may modify or override litter-mediated PSFs (Ke et al., 2015). Also, microbes in the rhizosphere can immobilize the nutrients liberated from plant litter, thereby buffering litter-mediated PSFs via nutrient cycling (Miki et al., 2010). These findings indicate the need to study rhizosphere- and litter-mediated PSFs in combination (see Box 1 for an example) to disentangle their interactions and relative importance. This will require full-factorial experiments where rhizosphere- and litter-mediated PSFs are manipulated. Within such studies it will be important to carefully consider spatial and temporal scales at which litter- and rhizosphere-mediated PSF effects occur, as these are not necessarily the same. In addition (stage-structured), models could be powerful to further explore how litter-mediated PSFs could operate (Ke et al., 2015; Campbell et al., 2016).
(2) Gain a better understanding of interactions between physical, chemical and biotic pathways of litter-mediated PSFs. For example, litter cover (i.e., physical) may enhance seedling establishment by creating favorable microclimatic conditions (Facelli et al., 1999), but it may have negative impacts on seedling survival due to allelopathy (i.e., chemical) (Wardle et al., 1998) or damage by litter fungal pathogens (i.e., biotic) (Beckstead et al., 2012). Integrating research across all three pathways will help us to untangle the key mechanisms driving litter-mediated PSFs. This will require experiments that specifically manipulate the physical, chemical or biotic pathway, for example by using sterilized litter to rule our biotic effects or fake (e.g., plastic) litter to identify physical effects. Alternatively, experiments using a more “holistic” approach including physical, chemical and biotic effects of litter without specifically disentangling them, may provide a better understanding of the overall impact of plant litter on PSFs. For example, plant roots from the first generation of plants can be left in the soil intact to allow for physical, chemical and biotic legacy effects of root litter on next generations of plants.
(3) Elucidate the species-specificity of litter-mediated PSF effects. Evidence is accumulating that plant litters build up unique decomposer communities (Lin et al., 2019; Veen et al., 2019), which may drive litter-mediated PSF via the biotic plant pathway. This may however generate relatively unspecific feedback effects, because different plant species may all profit from nutrients liberated from litter by specialized decomposer communities, however this is not tested. Future work should disentangle to what extent litter-mediated PSFs via the physical, chemical or biotic pathways result in species-specific effects on the growth and performance of next-generation plants.
(4) More emphasis is needed on the role of root litter decomposition in driving PSFs. So far, most work has focused on aboveground litter pathways, but root decomposition may be key in driving litter-mediated PSFs and, importantly, in modifying rhizosphere-mediated PSFs. Future studies should build on established concepts originating from work on shoot litter decomposition (Wardle et al., 2004; Austin et al., 2014), but they should also focus on the fundamental differences between root and shoot litter, such as impacts of continuous vs. seasonal litter inputs, or the depth of the roots in the soil.
(5) Litter-mediated PSFs need to be investigated under natural conditions, with a particular emphasis on the contexts under which such effects are most important. As PSF research moves out of the glasshouse and into the field (Kulmatiski and Kardol, 2008; De Long et al., 2019), it will be imperative to include litter-mediated PSFs alongside the more traditional rhizosphere-mediated PSFs when designing and executing experiments. Only via inclusion of litter-mediated PSFs will we be able to generate a more comprehensive understanding of PSFs and the ecological processes they control under natural conditions. Also, field PSF experiments allow for testing litter- and rhizosphere-mediated PSFs across larger spatial (i.e., at the level of plant communities) and temporal scales (i.e., multiple plant generations).
Data Availability Statement
The datasets generated for this study are available on request to the corresponding author and will be stored in the data archive of NIOO-KNAW.
Author Contributions
All authors have contributed to develop the ideas presented in this manuscript. GV and FH designed and performed the experiment (Box 1). GV has led the writing of the manuscript and all authors have contributed to individual sections. All authors have read and approved the final version of this manuscript.
Funding
GV (no. 863.14.013) and EM (no 863.15.021) were supported by a VENI grant from the Netherlands Organisation for Scientific Research (NWO). JD was supported by a VICI grant from NWO (no. 865.14.006). PK acknowledges support from the Swedish Research Council (Vetenskapsrådet).
Conflict of Interest
The authors declare that the research was conducted in the absence of any commercial or financial relationships that could be construed as a potential conflict of interest.
Acknowledgments
We thank Vereniging Natuurmonumenten for permission to collect soil and litter samples. We thank Tanja Bakx-Schotman, Erik Reichman, and Marta Manrubia for technical assistance with the experiment presented in Box 1. We thank Robin Heinen for drawing the plant in Figure 1. GV was supported by an NWO-VENI grant (no. 863.14.013). This is NIOO publication 6833.
References
Asplund, J., Hustoft, E., Nybakken, L., Ohlson, M., and Lie, M. H. (2018). Litter impair spruce seedling emergence in beech forests: a litter manipulation experiment. Scand. J. For. Res. 33, 332–337. doi: 10.1080/02827581.2017.1388440
Austin, A. T., Vivanco, L., González-Arzac, A., and Pérez, L. I. (2014). There's no place like home? An exploration of the mechanisms behind plant litter–decomposer affinity in terrestrial ecosystems. New Phytol. 204, 307–314. doi: 10.1111/nph.12959
Ayres, E., Steltzer, H., Simmons, B. L., Simpson, R. T., Steinweg, J. M., Wallenstein, M. D., et al. (2009). Home-field advantage accelerates leaf litter decomposition in forests. Soil Biol. Biochem. 41, 606–610. doi: 10.1016/j.soilbio.2008.12.022
Ballhausen, M.-B., and de Boer, W. (2016). The sapro-rhizosphere: carbon flow from saprotrophic fungi into fungus-feeding bacteria. Soil Biol. Biochem. 102, 14–17. doi: 10.1016/j.soilbio.2016.06.014
Barazani, O., and Friedman, J. (2001). Allelopathic bacteria and their impact on higher plants. Crit. Rev. Microbiol. 27, 41–55. doi: 10.1080/20014091096693
Bardgett, R. D., Mommer, L., and De Vries, F. T. (2014). Going underground: root traits as drivers of ecosystem processes. Trends Ecol. Evol. 29, 692–699. doi: 10.1016/j.tree.2014.10.006
Barrett, L. (1931). Influence of forest litter on the germination and early survival of chestnut oak, Quercus montana, Willd. Ecology 12, 476–484. doi: 10.2307/1928993
Beckstead, J., Miller, L. E., and Connolly, B. M. (2012). Direct and indirect effects of plant litter on a seed-pathogen interaction in Bromus tectorum seed banks. Seed Sci. Res. 22, 135–144. doi: 10.1017/S096025851100047X
Bennett, J. A., Maherali, H., Reinhart, K. O., Lekberg, Y., Hart, M. M., and Klironomos, J. (2017). Plant-soil feedbacks and mycorrhizal type influence temperate forest population dynamics. Science 355:181–184. doi: 10.1126/science.aai8212
Bever, J. D., Westover, K. M., and Antonovics, J. (1997). Incorporating the soil community into plant population dynamics: the utility of the feedback approach. J. Ecol. 85, 561–573. doi: 10.2307/2960528
Bezemer, T. M., Foutain, M. T., Barea, J. M., Christensen, S., Dekker, S. C., Duyts, H., et al. (2010). Divergent composition but similar function of soil food webs beneath individual plants: plant species and community effects. Ecology 91, 3027–3036. doi: 10.1890/09-2198.1
Boddy, L. (2016). “Interactions between fungi and other microbes,” in The Fungi, eds S. C. Watkinson, L. Boddy, and N. P. Money (Oxford, UK: Academic Press; Elsevier, 337–360.
Bonanomi, G., Incerti, G., Barile, E., Capodilupo, M., Antignani, V., Mingo, A., et al. (2011). Phytotoxicity, not nitrogen immobilization, explains plant litter inhibitory effects: evidence from solid-state 13C NMR spectroscopy. New Phytol. 191, 1018–1030. doi: 10.1111/j.1469-8137.2011.03765.x
Bradford, M. A., Veen, G. F., Bonis, A., Bradford, E. M., Classen, A. T., Cornelissen, J. H. C., et al. (2017). A test of the hierarchical model of litter decomposition. Nat. Ecol. Evol. 1, 1836–1845. doi: 10.1038/s41559-017-0367-4
Bradford, M. A., Warren, R. J. I. I., Baldrian, P., Crowther, T. W., Maynard, D. S., Oldfield, E. E., et al. (2014). Climate fails to predict wood decomposition at regional scales. Nat. Clim. Chang. 4, 625–630. doi: 10.1038/nclimate2251
Brinkman, E. P., van der Putten, W. H., Bakker, E. J., and Verhoeven, K. J. F. (2010). Plant-soil feedback: experimental approaches, statistical analyses and ecological interpretations. J. Ecol. 98, 1063–1073. doi: 10.1111/j.1365-2745.2010.01695.x
Campbell, E. E., Parton, W. J., Soong, J. L., Paustian, K., Hobbs, N. T., and Cotrufo, M. F. (2016). Using litter chemistry controls on microbial processes to partition litter carbon fluxes with the Litter Decomposition and Leaching (LIDEL) model. Soil Biol. Biochem. 100, 160–174. doi: 10.1016/j.soilbio.2016.06.007
Capek, P., Manzoni, S., Kaštovská, E., Wild, B., Diáková, K., Bárta, J., et al. (2018). A plant–microbe interaction framework explaining nutrient effects on primary production. Nat. Ecol. Evol. 2, 1588–1596. doi: 10.1038/s41559-018-0662-8
Cartenì, F., Bonanomi, G., Giannino, F., Incerti, G., Vincenot, C. E., Chiusano, M. L., et al. (2016). Self-DNA inhibitory effects: underlying mechanisms and ecological implications. Plant Signal. Behav. 11, e1158381. doi: 10.1080/15592324.2016.1158381
Cesarano, G., Incerti, G., and Bonanomi, G. (2016). The influence of plant litter on soil water repellency: insight from 13C NMR spectroscopy. PLoS ONE 11:e0152565. doi: 10.1371/journal.pone.0152565
Chomel, M., Fernandez, C., Bousquet-Mélou, A., Gers, C., Monnier, Y., Santonja, M., et al. (2014). Secondary metabolites of Pinus halepensis alter decomposer organisms and litter decomposition during afforestation of abandoned agricultural zones. J. Ecol. 102, 411–424. doi: 10.1111/1365-2745.12205
Chomel, M., Guittonny-Larchevêque, M., Fernandez, C., Gallet, C., DesRochers, A., Paré, D., et al. (2016). Plant secondary metabolites: a key driver of litter decomposition and soil nutrient cycling. J. Ecol. 104, 1527–1541. doi: 10.1111/1365-2745.12644
Collins, H. P., Delgado, J. A., Alva, A. K., and Follett, R. F. (2007). Use of nitrogen-15 isotopic techniques to estimate nitrogen cycling from a mustard cover crop to potatoes. Agron. J. 99, 27–35. doi: 10.2134/agronj2005.0357
Cortois, R., Schröder-Georgi, T., Weigelt, A., van der Putten, W. H., and De Deyn, G. B. (2016). Plant–soil feedbacks: role of plant functional group and plant traits. J. Ecol. 104, 1608–1617. doi: 10.1111/1365-2745.12643
Curry, J. P., and Schmidt, O. (2007). The feeding ecology of earthworms–a review. Pedobiologia 50, 463–477. doi: 10.1016/j.pedobi.2006.09.001
David, J.-F. (2014). The role of litter-feeding macroarthropods in decomposition processes: a reappraisal of common views. Soil Biol. Biochem. 76, 109–118. doi: 10.1016/j.soilbio.2014.05.009
De Long, J. R., Fry, E. L., Veen, G., and Kardol, P. J. F. E. (2019). Why are plant–soil feedbacks so unpredictable, and what to do about it? 33, 118–128. doi: 10.1111/1365-2435.13232
Donath, T. W., and Eckstein, R. L. (2008). Grass and oak litter exert different effects on seedling emergence of herbaceous perennials from grasslands and woodlands. J. Ecol. 96, 272–280. doi: 10.1111/j.1365-2745.2007.01338.x
Donath, T. W., and Eckstein, R. L. (2010). Effects of bryophytes and grass litter on seedling emergence vary by vertical seed position and seed size. Plant Ecol. 207, 257–268. doi: 10.1007/s11258-009-9670-8
Ehrenfeld, J. G., Ravit, B., and Elgersma, K. (2005). Feedback in the plant-soil system. Annu. Rev. Environ. Resour. 30, 75–115. doi: 10.1146/annurev.energy.30.050504.144212
el Zahar Haichar, F., Santaella, C., Heulin, T., and Achouak, W. (2014). Root exudates mediated interactions belowground. Soil Biol. Biochem. 77, 69–80. doi: 10.1016/j.soilbio.2014.06.017
Elgersma, K. J., Yu, S., Vor, T., and Ehrenfeld, J. G. (2012). Microbial-mediated feedbacks of leaf litter on invasive plant growth and interspecific competition. Plant Soil 356, 341–355. doi: 10.1007/s11104-011-1117-z
Eppinga, M. B., Kaproth, M. A., Collins, A. R., and Molofsky, J. (2011). Litter feedbacks, evolutionary change and exotic plant invasion. J. Ecol. 99, 503–514. doi: 10.1111/j.1365-2745.2010.01781.x
Facelli, J. M., and Pickett, S. T. A. (1991a). Plant litter–its dynamics and effects on plant community structure. Bot. Rev. 57, 1–32. doi: 10.1007/BF02858763
Facelli, J. M., and Pickett, S. T. A. (1991b). Plant litter–light interception and effects on an old-field plant community. Ecology 72, 1024–1031. doi: 10.2307/1940602
Facelli, J. M., Williams, R., Fricker, S., and Ladd, B. (1999). Establishment and growth of seedlings of Eucalyptus obliqua: interactive effects of litter, water, and pathogens. Austr. J. Ecol. 24, 484–494. doi: 10.1046/j.1440-169x.1999.00988.x
Freschet, G. T., Aerts, R., and Cornelissen, J. H. C. (2012a). Multiple mechanisms for trait effects on litter decomposition: moving beyond home-field advantage with a new hypothesis. J. Ecol. 100, 619–630. doi: 10.1111/j.1365-2745.2011.01943.x
Freschet, G. T., Aerts, R., and Cornelissen, J. H. C. (2012b). A plant economics spectrum of litter decomposability. Funct. Ecol. 26, 56–65. doi: 10.1111/j.1365-2435.2011.01913.x
Freschet, G. T., Cornwell, W. K., Wardle, D. A., Elumeeva, T. G., Liu, W., Jackson, B. G., et al. (2013). Linking litter decomposition of above and belowground organs to plant-soil feedbacks worldwide. J. Ecol. 101, 943–952. doi: 10.1111/1365-2745.12092
García, D. M., Bañuelos, J., and Houle, G. (2002). Differential effects of acorn burial and litter cover on Quercus rubra recruitment at the limit of its range in eastern North America. Can. J. Bot. 80, 1115–1120. doi: 10.1139/b02-102
Gartner, T. B., Treseder, K. K., Malcolm, G. M., and Sinsabaugh, R. L. (2012). Extracellular enzyme activity in the mycorrhizospheres of a boreal fire chronosequence. Pedobiologia 55, 121–127. doi: 10.1016/j.pedobi.2011.12.003
Gholz, H. L., Wedin, D. A., Smitherman, S. M., Harmon, M. E., and Parton, W. J. (2000). Long-term dynamics of pine and hardwood litter in contrasting environments: toward a global model of decomposition. Glob. Chang. Biol. 6, 751–765. doi: 10.1046/j.1365-2486.2000.00349.x
Hannula, S. E., Zhu, F., Heinen, R., and Bezemer, T. M. (2019). Foliar-feeding insects acquire microbiomes from the soil rather than the host plant. Nat. Commun. 10:1254. doi: 10.1038/s41467-019-09284-w
Hattenschwiler, S., and Gasser, P. (2005). Soil animals alter plant litter diversity effects on decomposition. Proc. Natl. Acad. Sci. U.S.A. 102, 1519–1524. doi: 10.1073/pnas.0404977102
Hättenschwiler, S., and Vitousek, P. M. (2000). The role of polyphenols in terrestrial ecosystem nutrient cycling. Trends Ecol. Evol. 15, 238–243. doi: 10.1016/S0169-5347(00)01861-9
Hobbie, S. E. (2015). Plant species effects on nutrient cycling: revisiting litter feedbacks. Trends Ecol. Evol. 30, 357–363. doi: 10.1016/j.tree.2015.03.015
Hobbie, S. E., Oleksyn, J., Eissenstat, D. M., and Reich, P. B. (2010). Fine root decomposition rates do not mirror those of leaf litter among temperate tree species. Oecologia 162, 505–513. doi: 10.1007/s00442-009-1479-6
Hood, R., Merckx, R., Jensen, E. S., Powlson, D., Matijevic, M., and Hardarson, G. (2000). Estimating crop N uptake from organic residues using a new approach to the 15N isotope dilution technique. Plant Soil 223, 33–46. doi: 10.1023/A:1004789103949
Joly, F. X., Coq, S., Coulis, M., Nahmani, J., and Hättenschwiler, S. (2018). Litter conversion into detritivore faeces reshuffles the quality control over C and N dynamics during decomposition. Funct. Ecol. 32, 2605–2614. doi: 10.1111/1365-2435.13178
Kaiser, C., Kilburn, M. R., Clode, P. L., Fuchslueger, L., Koranda, M., Cliff, J. B., et al. (2015). Exploring the transfer of recent plant photosynthates to soil microbes: mycorrhizal pathway vs direct root exudation. New Phytol. 205, 1537–1551. doi: 10.1111/nph.13138
Kardol, P., Bezemer, T. M., and van der Putten, W. H. (2006). Temporal variation in plant-soil feedback controls succession. Ecol. Lett. 9, 1080–1088. doi: 10.1111/j.1461-0248.2006.00953.x
Kardol, P., Veen, G. F., Teste, F. P., and Perring, M. P. (2015). Peeking into the black box: a trait-based approach to predicting plant-soil feedback. New Phytol. 206, 1–4. doi: 10.1111/nph.13283
Kardol, P., and Wardle, D. A. (2010). How understanding aboveground-belowground linkages can assist restoration ecology. Trends Ecol. Evol. 25, 670–679. doi: 10.1016/j.tree.2010.09.001
Ke, P.-J., Miki, T., and Ding, T.-S. (2015). The soil microbial community predicts the importance of plant traits in plant-soil feedback. New Phytol. 206, 329–341. doi: 10.1111/nph.13215
Keiser, A. D., Strickland, M. S., Fierer, N., and Bradford, M. A. (2011). The effect of resource history on the functioning of soil microbial communities is maintained across time. Biogeosciences 8, 1477–1486. doi: 10.5194/bg-8-1477-2011
Kremer, K. N., Promis, Á. A., Mancilla, G., and Magni, C. R. (2019). Leaf litter and irrigation can increase seed germination and early seedling survival of the recalcitrant-seeded tree Beilschmiedia miersii. Aust. Ecol. 44, 86–94. doi: 10.1111/aec.12655
Kulmatiski, A., Beard, K. H., Stevens, J. R., and Cobbold, S. M. (2008). Plant-soil feedbacks: a meta-analytical review. Ecol. Lett. 11, 980–992. doi: 10.1111/j.1461-0248.2008.01209.x
Kulmatiski, A., and Kardol, P. (2008). “Getting plant—soil feedbacks out of the greenhouse: experimental and conceptual approaches,” in Progress in botany, eds U. Lüttge, W. Beyschlag, and J. Murata (Berlin; Heidelberg: Springer, 449–472.
Lehmann, J., and Kleber, M. (2015). The contentious nature of soil organic matter. Nature 528, 60–68. doi: 10.1038/nature16069
Li, A., Fahey, T. J., Pawlowska, T. E., Fisk, M. C., and Burtis, J. (2015). Fine root decomposition, nutrient mobilization and fungal communities in a pine forest ecosystem. Soil Biol. Biochem. 83, 76–83. doi: 10.1016/j.soilbio.2015.01.019
Lin, D., Pang, M., Fanin, N., Wang, H., Qian, S., Zhao, L., et al. (2019). Fungi participate in driving home-field advantage of litter decomposition in a subtropical forest. Plant Soil 434, 467–480. doi: 10.1007/s11104-019-04210-x
Liu, S., Chen, J., He, X., Hu, J., and Yang, X. (2014). Trophic cascade of a web-building spider decreases litter decomposition in a tropical forest floor. Eur. J. Soil Biol. 65, 79–86. doi: 10.1016/j.ejsobi.2014.10.004
Makkonen, M., Berg, M. P., van Logtestijn, R. S., van Hal, J. R., and Aerts, R. (2013). Do physical plant litter traits explain non-additivity in litter mixtures? A test of the improved microenvironmental conditions theory. Oikos 122, 987–997. doi: 10.1111/j.1600-0706.2012.20750.x
Manrubia, M., Van der Putten, W. H., Weser, C., and Veen, G. F. (2019). Rhizosphere and litter feedbacks to range-expanding plant species and related natives. J. Ecol. doi: 10.1111/1365-2745.13299. [Epub ahead of print].
Mariotte, P., Mehrabi, Z., Bezemer, T. M., De Deyn, G. B., Kulmatiski, A., Drigo, B., et al. (2018). Plant–soil feedback: bridging natural and agricultural sciences. Trends Ecol. Evol. 33, 129–142. doi: 10.1016/j.tree.2017.11.005
Mariotte, P., Spotswood, E. N., Farrer, E. C., and Suding, K. N. (2017). Positive litter feedbacks of an introduced species reduce native diversity and promote invasion in Californian grasslands. Appl. Veg. Sci. 20, 28–39. doi: 10.1111/avsc.12291
Mazzoleni, S., Bonanomi, G., Incerti, G., Chiusano, M. L., Termolino, P., Mingo, A., et al. (2015). Inhibitory and toxic effects of extracellular self-DNA in litter: a mechanism for negative plant–soil feedbacks? New Phytol. 205, 1195–1210. doi: 10.1111/nph.13121
Miki, T., Ushio, M., Fukui, S., and Kondoh, M. (2010). Functional diversity of microbial decomposers facilitates plant coexistence in a plant–microbe–soil feedback model. Proc. Natl. Acad. Sci. U.S.A. 107, 14251–14256. doi: 10.1073/pnas.0914281107
Money, N. P. (2016). “Fungal cell biology and development,” in The Fungi, eds S. C. Watkinson, L. Boddy, and N. P. Money (Oxford, UK: Academic Press; Elsevier, 37–66.
Morriën, E., Hannula, S. E., Snoek, L. B., Helmsing, N. R., Zweers, H., de Hollander, M., et al. (2017). Soil networks become more connected and take up more carbon as nature restoration progresses. Nat. Commun. 8:14349. doi: 10.1038/ncomms14349
Muller, C. H. (1966). The role of chemical inhibition (allelopathy) in vegetational composition. Bull. Torrey Bot. Club. 93, 332–351. doi: 10.2307/2483447
Olson, B. E., and Wallander, R. T. (2002). Effects of invasive forb litter on seed germination, seedling growth and survival. Basic Appl. Ecol. 3, 309–317. doi: 10.1078/1439-1791-00127
Ormeno, E., Baldy, V., Ballini, C., Larchevêque, M., Périssol, C., and Fernandez, C. (2006). Effects of environmental factors and leaf chemistry on leaf litter colonization by fungi in a Mediterranean shrubland. Pedobiologia 50, 1–10. doi: 10.1016/j.pedobi.2005.07.005
Palozzi, J. E., and Lindo, Z. (2018). Are leaf litter and microbes team players? Interpreting home-field advantage decomposition dynamics. Soil Biol. Biochem. 124, 189–198. doi: 10.1016/j.soilbio.2018.06.018
Paul, K. I., Polglase, P. J., Smethurst, P. J., O'Connell, A. M., Carlyle, C. J., and Khanna, P. K. (2004). Soil temperature under forests: a simple model for predicting soil temperature under a range of forest types. Agric. For. Meteorol. 121, 167–182. doi: 10.1016/j.agrformet.2003.08.030
Penuelas, J., Prieto, P., Beier, C., Cesaraccio, C., de Angelis, P., de Dato, G., et al. (2007). Response of plant species richness and primary productivity in shrublands along a north-south gradient in Europe to seven years of experimental warming and drought: reductions in primary productivity in the heat and drought year of 2003. Glob. Chang. Biol. 13, 2563–2581. doi: 10.1111/j.1365-2486.2007.01464.x
Perez, G., Aubert, M., Decaens, T., Trap, J., and Chauvat, M. (2013). Home-field advantage: a matter of interaction between litter biochemistry and decomposer biota. Soil Biol. Biochem. 67, 245–254. doi: 10.1016/j.soilbio.2013.09.004
Philippot, L., Raaijmakers, J. M., Lemanceau, P., and Van Der Putten, W. H. (2013). Going back to the roots: the microbial ecology of the rhizosphere. Nat. Rev. Microbiol. 11, 789–799. doi: 10.1038/nrmicro3109
Poorter, H., Niklas, K. J., Reich, P. B., Oleksyn, J., Poot, P., and Mommer, L. (2012). Biomass allocation to leaves, stems and roots: meta-analyses of interspecific variation and environmental control. New Phytol. 193, 30–50. doi: 10.1111/j.1469-8137.2011.03952.x
Pregitzer, K. S., Kubiske, M. E., Yu, C. K., and Hendrick, R. L. (1997). Relationships among root branch order, carbon, and nitrogen in four temperate species. Oecologia 111, 302–308. doi: 10.1007/s004420050239
R Core Team (2013). R: A Language and Environment for Statistical Computing. Vienna: R Foundation for Statistical Computing.
Raaijmakers, J. M., Paulitz, T. C., Steinberg, C., Alabouvette, C., and Moënne-Loccoz, Y. (2009). The rhizosphere: a playground and battlefield for soilborne pathogens and beneficial microorganisms. Plant Soil 321, 341–361. doi: 10.1007/s11104-008-9568-6
Radosa, S., Ferling, I., Sprague, J. L., Westermann, M., and Hillmann, F. (2019). The different morphologies of yeast and filamentous fungi trigger distinct killing and feeding mechanisms in a fungivorous amoeba. Environ. Microbiol. 21, 1809–1820. doi: 10.1111/1462-2920.14588
Rillig, M. C., and Mummey, D. L. (2006). Mycorrhizas and soil structure. New Phytol. 171, 41–53. doi: 10.1111/j.1469-8137.2006.01750.x
Rodríguez, G. A. A., de Abreu, M. S., Pinto, F. A. M. F., Monteiro, A. C. A., Núñez, Á. M. P., de Resende, M. L. V., et al. (2016). Phialomyces macrosporus decreases anthracnose severity on coffee seedlings by competition for nutrients and induced resistance. Biol. Contr. 103, 119–128. doi: 10.1016/j.biocontrol.2016.08.009
Rousk, J., and Bååth, E. (2011). Growth of saprotrophic fungi and bacteria in soil. FEMS Microbiol. Ecol. 78, 17–30. doi: 10.1111/j.1574-6941.2011.01106.x
Scheu, S., and Schulz, E. (1996). Secondary succession, soil formation and development of a diverse community of oribatids and saprophagous soil macro-invertebrates. Biodivers. Conserv. 5, 235–250. doi: 10.1007/BF00055833
Semchenko, M., Leff, J. W., Lozano, Y. M., Saar, S., Davison, J., Wilkinson, A., et al. (2018). Fungal diversity regulates plant-soil feedbacks in temperate grassland. Sci. Adv. 4:eaau4578. doi: 10.1126/sciadv.aau4578
Sharratt, B. (2002). Corn stubble height and residue placement in the northern US Corn Belt: Part I. Soil physical environment during winter. Soil Tillage Res. 64, 243–252. doi: 10.1016/S0167-1987(01)00260-4
Shaw, M. W. (1968). Factors affecting the natural regeneration of sessile oak (Quercus petraea) in North Wales: II. Acorn losses and germination under field conditions. J. Ecol. 56, 647–660. doi: 10.2307/2258097
Siegrist, J. A., McCulley, R. L., Bush, L. P., and Phillips, T. D. (2010). Alkaloids may not be responsible for endophyte-associated reductions in tall fescue decomposition rates. Funct. Ecol. 24, 460–468. doi: 10.1111/j.1365-2435.2009.01649.x
Sokol, N. W., and Bradford, M. A. (2019). Microbial formation of stable soil carbon is more efficient from belowground than aboveground input. Nat. Geosci. 12:46–53. doi: 10.1038/s41561-018-0258-6
Swift, M. J., Heal, O. W., and Anderson, J. M. (1979). Decomposition in Terrestrial Ecosystems. Oxford, UK: Blackwell Scientific.
Teasdale, J. R., Beste, C. E., and Potts, W. E. (1991). Response of weeds to tillage and cover crop residue. Weed Sci. 39, 195–199. doi: 10.1017/S0043174500071460
Teste, F. P., Kardol, P., Turner, B. L., Wardle, D. A., Zemunik, G., Renton, M., et al. (2017). Plant-soil feedback and the maintenance of diversity in Mediterranean-climate shrublands. Science 355, 173–176. doi: 10.1126/science.aai8291
Vahdat, E., Nourbakhsh, F., and Basiri, M. (2011). Lignin content of range plant residues controls N mineralization in soil. Eur. J. Biol. 47, 243–246. doi: 10.1016/j.ejsobi.2011.05.001
van der Putten, W. H., Bardgett, R. D., Bever, J. D., Bezemer, T. M., Casper, B. B., Fukami, T., et al. (2013). Plant-soil feedbacks: the past, the present and future challenges. J. Ecol. 101, 265–276. doi: 10.1111/1365-2745.12054
van der Putten, W. H., Bradford, M. A., Brinkman, E. P., van de Voorde, T. F. J., and Veen, G. F. (2016). Where, when and how plant-soil feedback matters in a changing world. Funct. Ecol. 30, 1109–1121. doi: 10.1111/1365-2435.12657
van der Putten, W. H., Mortimer, S. R., Hedlund, K., Van Dijk, C., Brown, V. K., Leps, J., et al. (2000). Plant species diversity as a driver of early succession in abandoned fields: a multi-site approach. Oecologia 124, 91–99. doi: 10.1007/s004420050028
van der Wal, A., Ottosson, E., and de Boer, W. (2015). Neglected role of fungal community composition in explaining variation in wood decay rates. Ecology 96, 124–133. doi: 10.1890/14-0242.1
Veen, G. F., Freschet, G. T., Ordonez, A., and Wardle, D. A. (2015). Litter quality and environmental controls of home-field advantage effects on litter decomposition. Oikos 124, 187–195. doi: 10.1111/oik.01374
Veen, G. F., Keiser, A. D., van der Putten, W. H., and Wardle, D. A. (2018). Variation in home-field advantage and ability in leaf litter decomposition across successional gradients. Funct. Ecol. 32, 1563–1574. doi: 10.1111/1365-2435.13107
Veen, G. F., Snoek, B. L., Bakx-Schotman, T., Wardle, D. A., and van der Putten, W. H. (2019). Relationships between fungal community composition in decomposing leaf litter and home-field advantage effects. Funct. Ecol. 33, 1524–1535. doi: 10.1111/1365-2435.13351
Vidal, A., Quenea, K., Alexis, M., Tu, T. N., Mathieu, J., Vaury, V., et al. (2017). Fate of 13C labelled root and shoot residues in soil and anecic earthworm casts: A mesocosm experiment. Geoderma 285, 9–18. doi: 10.1016/j.geoderma.2016.09.016
Vos, V. C., van Ruijven, J., Berg, M. P., Peeters, E. T., and Berendse, F. (2011). Macro-detritivore identity drives leaf litter diversity effects. Oikos 120, 1092–1098. doi: 10.1111/j.1600-0706.2010.18650.x
Walia, M. K., and Dick, W. A. (2018). Selected soil physical properties and aggregate-associated carbon and nitrogen as influenced by gypsum, crop residue, and glucose. Geoderma 320, 67–73. doi: 10.1016/j.geoderma.2018.01.022
Wardle, D. A., Bardgett, R. D., Klironomos, J. N., Setälä, H., van der Putten, W. H., and Wall, D. H. (2004). Ecological linkages between aboveground and belowground biota. Science 304, 1629–1633. doi: 10.1126/science.1094875
Wardle, D. A., Jonsson, M., Bansal, S., Bardgett, R. D., Gundale, M. J., and Metcalfe, D. B. (2012). Linking vegetation change, carbon sequestration and biodiversity: insights from island ecosystems in a long-term natural experiment. J. Ecol. 100, 16–30. doi: 10.1111/j.1365-2745.2011.01907.x
Wardle, D. A., Nilsson, M. C., Gallet, C., and Zackrisson, O. (1998). An ecosystem-level perspective of allelopathy. Biol. Rev. 73, 305–319. doi: 10.1111/j.1469-185X.1998.tb00033.x
Wubs, E. J., van der Putten, W. H., Bosch, M., and Bezemer, T. M. (2016). Soil inoculation steers restoration of terrestrial ecosystems. Nat. Plants 2:16107. doi: 10.1038/nplants.2016.107
Zhang, J., and Elser, J. J. (2017). Carbon: nitrogen: phosphorus stoichiometry in fungi: a meta-analysis. Front. Microbiol. 8:1281. doi: 10.3389/fmicb.2017.01281
Zhang, N., Van der Putten, W. H., and Veen, G. F. (2016). Effects of root decomposition on plant–soil feedback of early- and mid-successional plant species. New Phytol. 212, 220–231. doi: 10.1111/nph.14007
Keywords: decomposition, indirect plant-soil feedback effects, allelopathy, home-field advantage, rhizosphere-mediated feedback, litter-mediated feedback
Citation: Veen GF, Fry EL, ten Hooven FC, Kardol P, Morriën E and De Long JR (2019) The Role of Plant Litter in Driving Plant-Soil Feedbacks. Front. Environ. Sci. 7:168. doi: 10.3389/fenvs.2019.00168
Received: 13 June 2019; Accepted: 07 October 2019;
Published: 22 October 2019.
Edited by:
Jasmin Joshi, University of Applied Sciences Rapperswil, SwitzerlandReviewed by:
Alix Vidal, Technical University of Munich, GermanyLucia Fuchslueger, University of Antwerp, Belgium
Copyright © 2019 Veen, Fry, ten Hooven, Kardol, Morriën and De Long. This is an open-access article distributed under the terms of the Creative Commons Attribution License (CC BY). The use, distribution or reproduction in other forums is permitted, provided the original author(s) and the copyright owner(s) are credited and that the original publication in this journal is cited, in accordance with accepted academic practice. No use, distribution or reproduction is permitted which does not comply with these terms.
*Correspondence: G. F. (Ciska) Veen, Yy52ZWVuQG5pb28ua25hdy5ubA==