- 1Department of Geography, Environmental Studies and Tourism, University of the Western Cape, Bellville, South Africa
- 2Department of Earth Science, Institute for Water Studies, University of the Western Cape, Bellville, South Africa
- 3Department of Geography, Rhodes University, Grahamstown, South Africa
- 4Kirstenbosch Research Centre, Freshwater Biodiversity Institute, South African National Biodiversity Institute, Cape Town, South Africa
Due to climatic constraints in dryland regions, wetlands usually occur at confluences of flow paths, whether from surface flow, inter-flow or at locations of groundwater discharge. Long-term landscape processes that shape valleys and focus the movement of water and sediment are accountable for providing a suitable template with which hydrology interacts to allow wetland formation. Current hydrogeomorphic classification systems do not address system-scale linkages of sediment and water transport across the landscape, and are therefore unable to contextualise long-term process dynamics. Misunderstanding long-term earth system processes can result in the application of inappropriate restoration strategies that isolate wetlands from longitudinal drivers of their formation. We propose a genetic classification system that focuses on the mode of wetland formation, and is based on the understanding that genetic processes impact on the outcome hydrology, sedimentology, geomorphology, ecosystem service provision, and long-term dynamics of wetlands in drylands. The classification aims to impart understanding of dynamic processes of sediment transport through wetlands, such that restoration plans can be sensitive to long-term landscape processes. The classification system, derived from a combination of international literature and published South African case studies, has four wetland macrotypes based on sediment source (colluvial, alluvial, Aeolian, and geochemical). These are subdivided into eight wetland types; hillslope seep, floodplain, valley-bottom, plain, blocked-valley, alluvial fan, aeolian depression, and geochemical depression. The classification is based on landscape location, shape, and the occurrence of geomorphic characteristics indicative of process.
Introduction
Another Wetland Classification System?
The majority of wetlands in southern Africa are located along drainage lines due to widespread semi-arid conditions associated with the sub-tropical high pressure belt (Ellery et al., 2009). This is in contrast to more humid environments, where wetlands may occur in a wider variety of landscape settings due to a favourable water balance in which rainfall equals or exceeds evaporation. In southern Africa, with the exception of a few coastal and mountainous catchments, annual potential evaporation generally exceeds annual rainfall, in many areas by a factor of two to three (Schulze, 1997). As a result, wetlands usually occur in areas of flow accumulation concentration, whether from surface flow (channel or surface runoff) or inter-flow (within soil and bedrock), or occasionally at locations of groundwater discharge. In dryland regions (MAP:PET < 0.65; UNEP, 1997), long-term geomorphological processes and fluxes of earth surface materials shape valleys and concentrate surface and near-surface flow accumulation, providing the conditions necessary for wetland formation (Tooth and McCarthy, 2007; Tooth et al., 2015; Lidzhegu, 2019; Lisenby et al., 2019).
In this context, wetlands can be considered geomorphic systems that are dynamic in form as well as in their fluxes of water and all constituent matter (sediment, solutes), and are responsive to natural and anthropogenically-induced changes in water, sediment, and solute supply (Tooth, 2018; Lisenby et al., 2019). Wetland dynamics may respond to a combination of autogenic and allogenic forcing (Tooth et al., 2007; Larkin et al., 2017). For instance, valley-bottom wetlands become vulnerable to incision once a threshold slope (sensu Schumm, 1979) is exceeded for a particular catchment size and rainfall (Ellery et al., 2009, 2016). This concept was extended by Tooth (2018) for meandering river floodplain wetlands, through consideration of a threshold of wetland dynamics determined by interactions between discharge, slope, and sediment availability (supply), where systems subject to sudden and substantial morphological changes may be separated from systems subject to gradual and relatively continuous adjustments of form. In some cases, changes to catchment run-off that alter the water to sediment supply ratio may lead to either erosion (where Q > Qs) or aggradation (where Qs > Q) (Gell et al., 2009; Tooth, 2018). In other cases, changes in rates of lateral erosion and accretion reflect a change in the elevation of a geologically controlled local base level (e.g., Tooth et al., 2004; Ellery et al., 2012; Keen-Zebert et al., 2013). Changes in catchment run-off and thus rates of sediment transport, erosion or deposition may also occur naturally due to climatic oscillations (e.g., Aalto et al., 2003; Macklin and Lewin, 2003; Grenfell and Ellery, 2009) or may be forced by anthropogenically-induced climate change. Understanding the temporal and spatial scales of these dynamics, as well as the drivers behind them, is key to successful wetland management and restoration.
In this paper, we propose a new genetic geomorphic classification system that acknowledges the role of geomorphology in wetland formation (Tooth et al., 2015), and aims to connect observable geomorphic features in the landscape with the processes that created them (Buffington and Montgomery, 2013; Lisenby et al., 2019). Wetland classification systems are usually based on a selected wetland definition, the most commonly used being that of Cowardin et al. (1979), which requires that a wetland meets one or more of the following criteria: (1) it supports hydrophytes at least periodically, (2) the substrate is composed of undrained hydric soil and/or (3), the substrate is non-soil and is saturated or covered by shallow water at some time during the growing season of each year. This particular definition has been used as the starting point for several wetland hydrogeomorphic classification systems, including those of Cowardin and Golet (1995), Brinson (1993) and Semeniuk and Semeniuk (1995). According to Finlayson and van der Valk (1995), the primary reason for developing a classification system is to support wetland inventory by standardising and defining terms to describe wetland types to allow successful wetland conservation and management. We argue that a wetland classification system can be more than a system for inventory, and that it can also be used as a tool to improve understanding of wetland processes and dynamics (Kondolf and Piégay, 2016).
A failure to appropriately conceptualise natural and human induced trajectories of change in wetlands, in tandem with a lack of appreciation of the importance of longitudinal (dis)connectivity, can result in poor outcomes for wetland restoration in dryland environments. For example, the hydrogeomorphic classification scheme currently in use in South Africa, developed by Ewart-Smith et al. (2006) and Ollis et al. (2015), incorporates very little geomorphological insight beyond consideration of the landscape setting, and emphasises “product” (landscape setting) over “process” (dynamic feedbacks between process and form) geomorphology (Lisenby et al., 2019). The result is that short-term hydrological processes are considered the master variable in wetlands with developmental histories that in many cases extend over several millennia, and have been shaped by a highly complex interplay between a geological template and dynamic flows and fluxes of earth surface material (Tooth and McCarthy, 2007; Lisenby et al., 2019). As Simenstad et al. (2006) suggest, without recognising landscape dynamics, wetland restoration efforts are unlikely to realise the full ecosystem performance that is sought.
The paper sets out the physical basis of the proposed genetic geomorphic classification system by outlining a generic set of geomorphic modes of wetland formation common in dryland environments, by providing a context for geomorphic change and processes typically associated with each mode of formation, and by clarifying when and how longitudinal (dis)connectivity in material fluxes most significantly influences wetland ecological integrity. The system is considered a genetic classification as it is based on the fundamental landscape processes that result in the formation of each wetland type. The system does not consider hydroperiod explicitly, but rather aims to describe landscape setting and landform, and consider hydrologically-linked processes of sediment erosion, transport and deposition that may be associated with each wetland.
Existing Wetland Classification Systems
Cowardin and Golet (1995) developed one of the most comprehensive and widely used ecologically-based wetland classification systems for the United States. This hierarchical system can be used alongside several hydrological, soil and chemistry modifiers. This is the basis of several regional classification systems used in North America (e.g., US Federal Geographic Data Committee, 2013; Alberta Environment Sustainable Resource Development, 2015). Palustrine wetlands may be divided into seven classes based on the areal extent of either the dominant life form or substrate composition. The selection of life form and substrate as classification criteria was based on the reasoning that these would be easily identifiable, even on satellite imagery, and that they do not change seasonally. The classification is descriptive and its simplicity is useful for inventory purposes. However, a single wetland system may fall within several classes depending on the mapping resolution. Furthermore, the classification provides no indication of the processes that have resulted in the pattern of vegetation being mapped. For instance, a reed-dominated emergent wetland could occur in multiple landscape environments, such as in a tidal lagoon, on the bed of a non-perennial river or on the margins of a floodplain. Thus, while this approach is useful for cataloguing purposes, there is no indication of the geomorphic or hydrological processes that are likely to drive long-term functioning.
The Ramsar “Classification System for Wetland Type” approved by the 1990 Conference of the Contracting Parties (Recommendation 4.7) with subsequent amendments, is a broad framework for rapid identification that consists of three categories with 42 wetland types. The classification is designed for rapid assessment and is therefore not scientifically exhaustive, and makes use of a variety of inconsistently applied discriminant criteria such as geomorphic form, hydroperiod, water quality, substrate type, and vegetation type. As a result, it is possible for a single wetland to fall into multiple types. Once again, the classification is descriptive and is focussed on classifying the outcome variables (i.e., vegetation) rather than the driving factors behind wetland formation.
In contrast to these, Brinson (1993) and Semeniuk and Semeniuk (1995) focussed on landform and hydrology in order to provide a better assessment of the physical, chemical, and biological functioning of different wetland types. The underlying rationale for such an approach was that hydrogeomorphic processes could be used to classify wetlands distributed across a wide range of climatic, geological, soil, and vegetation settings.
In Semeniuk and Semeniuk (1995) geomorphic classification system for Australian inland wetlands, discrimination is based on landform and hydroperiod as these are considered the primary controls on wetland formation. For instance, in a dryland environment, a flat could be permanently dry, while in a more humid environment, the flat might be a wetland. As in Cowardin and Golet (1995), other factors such as water quality and vegetation type may be used to augment the classification. While useful for the purpose of inventory, the classification system provides no information about wetland morphodynamics, which is essential for determining a sustainable approach to wetland restoration (Ellery et al., 2009). For example, a floodplain is classified as a “seasonally inundated flat,” and according to the definition, this wetland type can refer to a flat that may or may not be linked to a river. Thus, all floodplains are classed as one type, despite variations in sediment flux and exchange flux processes, and the implications of such processes for ecosystem dynamics and the delivery of ecosystem services (Kotze et al., 2009). In dryland environments, a deeper understanding of floodplain wetlands, especially with regards to flow variability and its impact on geomorphic processes, is vital if we are to fully appreciate their potential to recover following disturbance, as well as to evaluate ecosystem service delivery (Thoms, 2006; Grenfell S. E. et al., 2009).
Nanson and Croke (1992) provide a comprehensive process-based floodplain classification that is based on the ability of a river to entrain and transport sediment in conjunction with the erosional resistance of floodplain alluvium. The system has three classes (high-energy non-cohesive, medium-energy non-cohesive, and low-energy cohesive) that are sub-divided by predominant floodplain forming processes. The resultant floodplain types are distinctive in terms of morphology and genesis. Furthermore, as the classification is based on processes, it acknowledges that it is possible for floodplains to transform from one type to another. A number of similar approaches have been applied in the empirical discrimination of channel planform pattern (for relatively recent examples see Nanson and Knighton, 1996; Kleinhans, 2010; Kleinhans and Van den Berg, 2011). Although focussed on channel planform, these classifications incorporate morphodynamic processes since channel planform is dependent on feedbacks between bars, channels, floodplain, and vegetation (Kleinhans and Van den Berg, 2011).
A classification system for all inland aquatic ecosystems, including rivers and wetlands, was developed for South Africa by Ewart-Smith et al. (2006) and Ollis et al. (2015). The wetland classification builds on earlier work by Kotze et al. (1994, 2009), with the latter works largely based on Brinson (1993). The system is also hierarchical, with landscape setting incorporated at level 3 (valley floor, bench, plain, or slope). Hydrogeomorphic units, introduced at level 4, are defined according to landform, hydrological characteristics and hydrodynamics, and include river, floodplain, channelled valley-bottom, unchannelled valley-bottom, depression, seep, and wetland flat. Ollis et al. (2015) argue that the “functional unit,” which may be derived at level 5 by applying a secondary discriminator (saturation period) to the hydrogeomorphic type, defines the functioning of the aquatic ecosystem. Functional units at this scale are not useful when planning restoration as this approach does not recognise that wetlands are part of an integrated drainage system with fluxes of sediment and water between functional units that are as important as the units themselves (c.f. Chorley and Kennedy, 1971). Furthermore, these fluxes shape unit dynamics over decadal to centennial timescales (e.g., Tooth et al., 2014).
In practise, many wetlands do not fit comfortably into this classification. For instance, wetlands located in blocked valleys have characteristics of valley-bottom wetlands as well as depressions (Grenfell et al., 2008, 2010). In addition, many valley-bottom wetlands are discontinuous and have reaches that are channelled and unchannelled (Grenfell M. C. et al., 2009; Grenfell et al., 2012; Tooth et al., 2014). It is also difficult to objectively determine the difference between a floodplain wetland and a channelled valley-bottom wetland, for which Ollis et al. (2015) recommend the identification of meandering river floodplain geomorphic features (e.g., backwater depressions, meander cut-offs, alluvial ridges, scroll bars, and leveés). The occurrence of these features is skewed toward identifying single-thread meandering river floodplains. From a geomorphic origin and process point of view, it is clear that the channelled valley-bottom is a type of floodplain, and there is a need to discriminate additional floodplain types characterised by floodplain features and processes (Nanson and Croke, 1992; Lisenby et al., 2019).
The decision as to what classification level or unit should be used for different levels of analysis or inventory is complicated by the inter-disciplinary nature of wetland research. Sieben et al. (2011) argue that hydrogeomorphic units exhibit similar hydrological and geomorphological characteristics, and that these units should be used in wetland restoration planning. This is consistent in principle with the view of Ollis et al. (2015). Since some ecosystem services are linked to specific habitat types, Sieben et al. (2018) suggest that in order to more accurately assess ecosystem service provision in individual wetlands, functional HGM units should be further sub-divided by vegetation class. The authors suggest that this is particularly important when considering ecosystem services that are primarily associated with the functional unit but where their delivery is modified by vegetation type. However, the division of wetland systems into smaller management units is risky as it encourages piecemeal planning and conservation efforts. For instance, if management begins to focus on conserving a specific vegetation type within a specific functional unit nested within a larger hydrogeomophic unit, the chance of success is limited by failure to recognise process-linkages between system components (Dollar et al., 2007). Flows of sediment and water occur between functional units and vegetation classes. While wetland restoration planning should consider the restoration of habitat patches in order to conserve threatened species or plant communities, this should be done at a system scale to ensure system integrity.
A genetic Geomorphic Classification System for Palustrine Wetlands in DRYLANDS
Several authors have highlighted the necessity of ensuring any proposed classification and inventory system meets the needs of managing agencies and is accessible and relevant (Finlayson and van der Valk, 1995; Scott and Jones, 1995). We propose that a genetic geomorphic classification system in the context of dryland wetlands can provide more than a series of classes on which to base conservation efforts, but that this classification system will improve understanding of wetland geomorphic processes that govern the movement of water and all constituent matter through the ecosystem at varied spatial and temporal scales (Lisenby et al., 2019). Our proposed classification system considers a wetland unit to be one which meets the Cowardin et al. (1979) definition, and by integrating process and response, is associated with a particular mode of formation. The wetland unit may comprise areas that are hydrologically varied (i.e., some parts may be permanently wet, others seasonally or temporarily), but these zones are often fairly predictable based on the type or sub-type.
The first level of the hierarchical classification divides wetlands according to the dominant sediment source responsible for the formation of the wetland (Table 1). The wetland macrotype may be described as either colluvial (sediment deposited under the influence of gravity by slope processes), alluvial (sediment deposited by fluvial processes), aeolian (related to or arising from wind action), or geochemical (sediment derived from in situ weathering of mineral parent material and/or solution translocation and precipitation). The wetland macrotype therefore distinguishes the dominant geomorphic processes driving wetland formation.
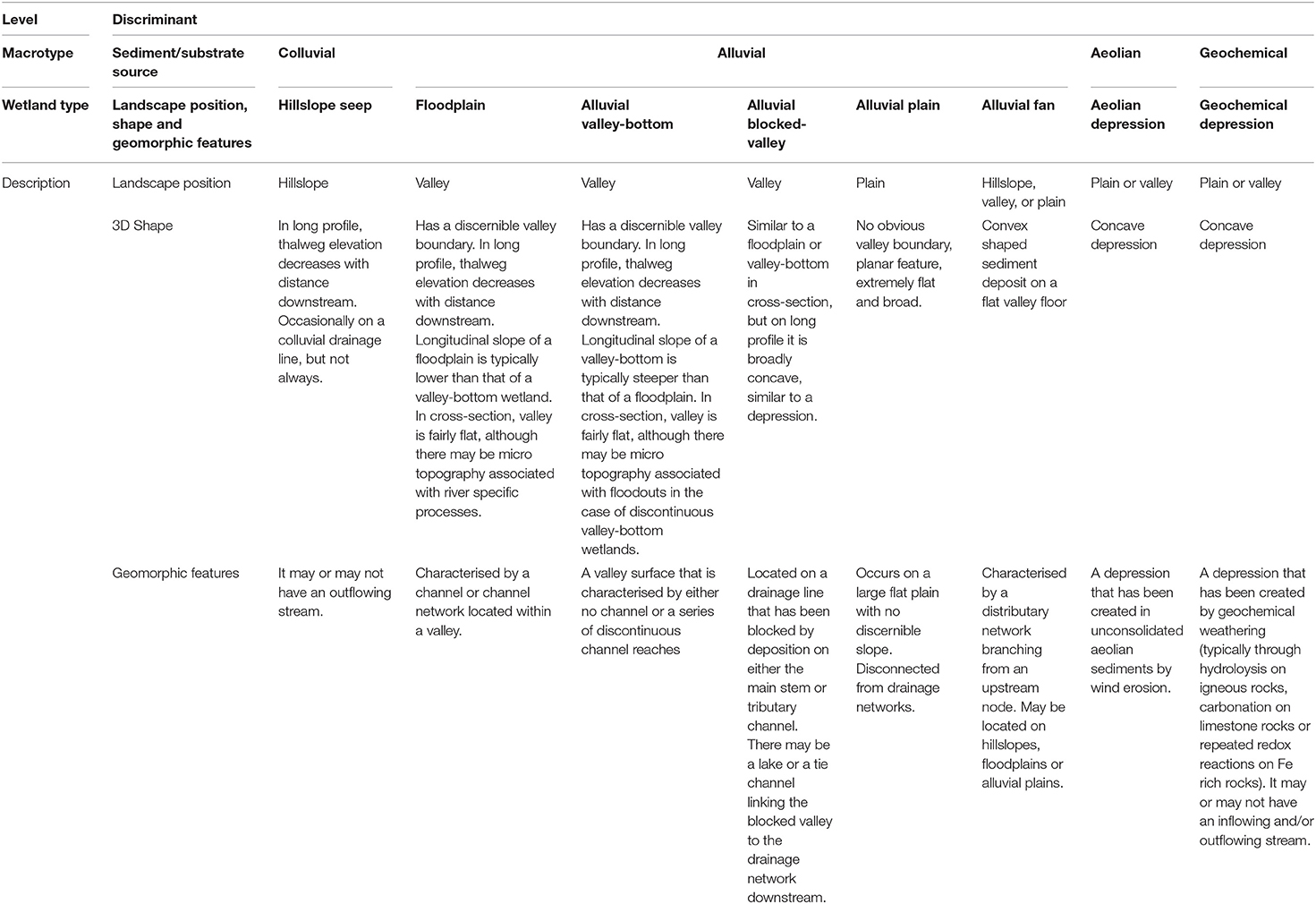
Table 1. Macrotypes and types of a genetic geomorphic classification of inland wetlands for dryland regions, sub-types are provided on subsequent tables where applicable.
Level 2 of the classification system describes wetland type, where discrimination is based on three variables; (1) landscape position (hillslope, valley or plain), (2) 3-dimensional shape (a description of cross-section and longitudinal slope), and (3) typical geomorphic features (presence or absence of channel, connectivity to channel network, and/or being within a depression). This is not an attempt to classify the wetland based on topography, but rather an acknowledgment that the landscape, particularly the wetland landform, is a product of geomorphic processes. Therefore, in contrast with Lisenby et al. (2019), we do use the terms “slope” and “flat” where required, but explicitly conceptualise these terms in the context of geomorphic mode of origin. The wetland type may be classified as a hillslope seep, hillslope plateau, alluvial floodplain, alluvial valley-bottom, alluvial plain, alluvial blocked-valley, alluvial fan, aeolian depression or geochemical depression.
Colluvial hillslope seeps, alluvial floodplains and valley-bottoms, and geochemical depressions may be further subdivided into sub-types at level 3 on the basis of process dynamics (characteristic processes of erosion and deposition, or dissolution and precipitation in the case of geochemical depressions). Hillslope seeps may be divided based on the presence or absence of discontinuous channel outflow (as in Kotze et al., 2009), valley-bottoms may be divided based on the presence or absence of a discontinuous river channel, floodplains may be discriminated based on indicators of lateral channel activity and sinuosity, while geochemical depressions may be separated based on the type of underlying bedrock. A detailed descriptor for each type and sub-type is provided below, while satellite images of distinct types are provided in Figure 1.
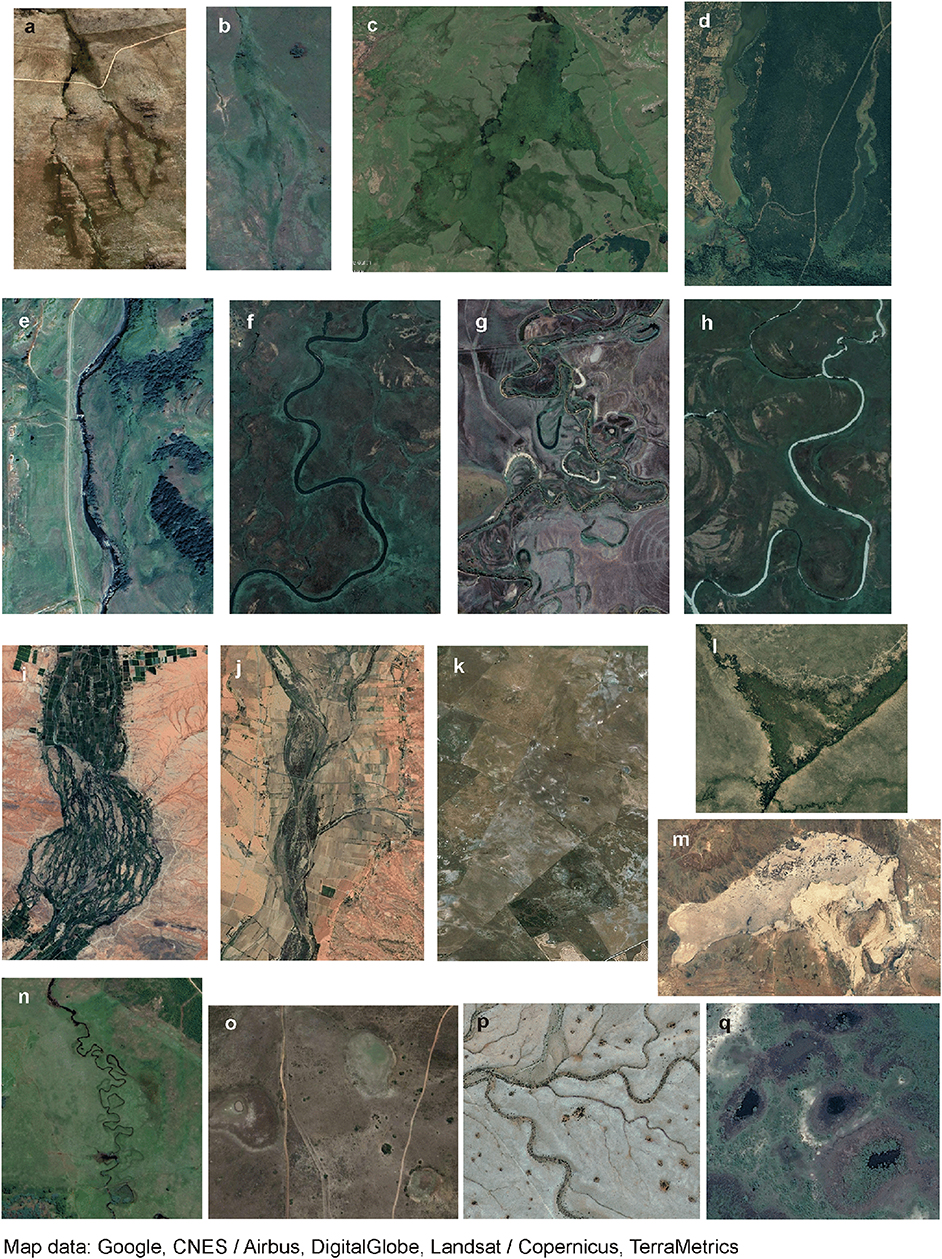
Figure 1. Google Earth images of representative wetland types and sub-types, scale is variable to ensure visibility of features. (a) Hillslope seeps with and without stream, Swartberg, South Africa; (b) discontinuous valley-bottom, Mooi River catchment, South Africa; (c) unchannelled valley-bottom, Ntsikeni, South Africa; (d) blocked-valley depression, Muzi pan with Mkuze River towards south, South Africa; (e) straight river floodplain, Lotheni River, South Africa; (f) sinuous river floodplain, Okavango Delta, Botswana; (g) meandering river floodplain, Mzimvubu River, South Africa; (h) anastomosed river floodplain Okavango Delta, Botswana; (i) mixed bedrock-alluvial anabranching river floodplain, Orange River, South Africa; (j) wandering river floodplain, Olifants River, South Africa; (k) alluvial flat, Agulhas Plain, South Africa; (l) alluvial fan, Linyanti Swamp, Botswana; (m) aeolian depression, Verneukpan, South Africa; (n) subsidence depression, Dartmoor, South Africa; (o) redox depression, Shadowvlei, South Africa; (p) dissolution depressions, Ubib embayment, Namibia; and (q) margin-aggradation depression, Okavango Delta, Botswana.
Colluvial WetlandS
Hillslope Seep Wetlands
Sediment in colluvial wetlands accumulates via colluviation from upslope as well as by in situ pedogenesis which is enhanced by the presence of water. Seep wetlands originate under the combined influence of topography and underlying stratigraphy, and as a result, Brinson (1993) discriminates between topographic seeps, which form at the convergence of lateral subsurface water in concave areas, and stratigraphic seeps which occur where a slope intersects the lateral flow of water. In dryland environments, hillslope topographic seeps fed by regional groundwater aquifer discharge have a restricted distribution associated with relatively rare artesian flow systems. Instead, almost all hillslope seeps are stratigraphic and form where a layer with restricted permeability deflects water that has filtered through the soils of the upper slope via gravity. The layer of restricted permeability may occur either at the soil/bedrock interface or within the soil where there is a change in soil texture associated with reduced hydraulic conductivity (Kuenene et al., 2011, 2013; Van Tol et al., 2011). The relative source area of water contribution determines the degree of saturation as well as the duration and timing of inundation of the seep wetland (Jaeger et al., 2007; Job and Le Roux, 2018).
The landscape form of seep wetlands is variable (Figure 2). Hillslope longitudinal slope can range from very steep to relatively flat, provided there is sufficient slope for unidirectional subsurface flow of water. Cross-sectional morphology can range from slight to moderately concave. Such wetlands have a low hydraulic radius and high roughness due to dense vegetation cover, which both contribute to diffuse flow. All hillslope seep wetlands are vulnerable to activities in the catchment that interrupt or reduce the infiltration and percolation of water into the sub-surface soil and/or rock, such as the introduction of hardened surfaces or development of tree plantations. Hillslope seep wetlands may be divided into sub-types on the basis of the geomorphic process of channel initiation which has implications for processes of sediment transport downslope (Table 2).
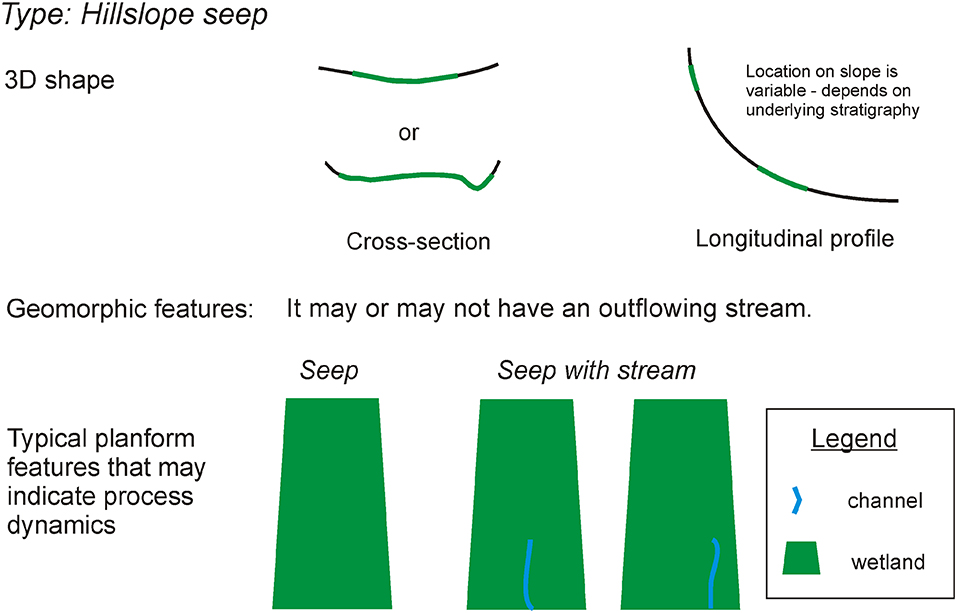
Figure 2. Schematic shape and geomorphic features that are used to classify hillslope seep wetland type, followed by typical planform features that are process indicators used to classify wetland sub-type.
Seep
In situations where subsurface discontinuities in geological units (e.g., faults) or water-bearing geologies (e.g., fractured bedrock) intersect the ground surface, the water source may be expressed as localised patches that are seasonally to permanently wet within the overall seep wetland. Depending on the size and climate of the source area, flows may maintain saturated conditions year-round, resulting in a shallow but predictably stable local water-table. Continuous water replacement may result in more frequent oxidising conditions relative to the more dominant reducing conditions of stagnant saturated soils.
Seep With Stream
Streams are initiated when a threshold defined by water supply and slope is exceeded. This is described by Montgomery and Dietrich (1988), who found that source area above a channel head decreased as slope increased, suggesting that in steep, humid landscapes, channels are initiated by land sliding. The relationship between source area and slope of channel initiation varies depending on local climatic and geological conditions. For the same slope, drier regions have larger source areas (Montgomery and Dietrich, 1988).
Where seeps occur in more gentle settings, channel initiation may be through overland flow where the amount of contributed water exceeds the storage capacity of the soils due to the presence of an underlying aquitard, resulting in surface flow. The resulting stream either occurs toward the centre of the wetland in a concave setting, or if slight sediment accumulation occurs in the middle of the wetland, the channel may form to the side.
In regions where subsurface lateral flows dominate the water source, it is possible to establish a relationship between duration and depth of inundation and the source area. In contrast, where the water is sourced primarily from fractures, bedding planes or faults in bedrock, the relationship between hydroperiod and source area is not consistent (Job and Le Roux, 2018). Channels that flow out of seep wetlands typically connect to the drainage network, and although of extremely low power, they do sporadically contribute to the downslope movement of colluvially derived sediment through land sliding in steeper areas, or surface flow sediment transport in more gentle areas.
Alluvial Wetlands
Floodplain Wetlands
The floodplain classification presented here is adapted from Kleinhans and Van den Berg (2011). Braided river floodplains have been omitted as they are rare in southern Africa, probably because there have been no recent glaciations in the region to support mechanical weathering processes required to provide large volumes of gravel and cobble sized sediment. Where stream powers are high enough to support the development of braided rivers and there is a sufficient supply of bedload sediment, rivers are confined within a well-dissected drainage network developed in relatively erosion-resistant lithologies (e.g., basalt, dolerite, quartzite) or in lithologies that weather readily to sand, silt, and clay (sandstones, mudstones). Furthermore, braided river floodplains do not generally support edaphic environments suited to the widespread establishment of wetland vegetation—dynamic braidplains are characterised by low vegetation densities, as stable multi-channel networks are favoured where vegetation is sufficiently dense (Kleinhans, 2010). Floodplains may be classified into sub-types based on the presence of single or multiple channels, sinuosity, and evidence of lateral erosion and deposition (Figure 3, Table 3). In all floodplains, the dominant water input is from the channel and associated overtopping, while in some instances, lateral seepage from the valley sides may be important.
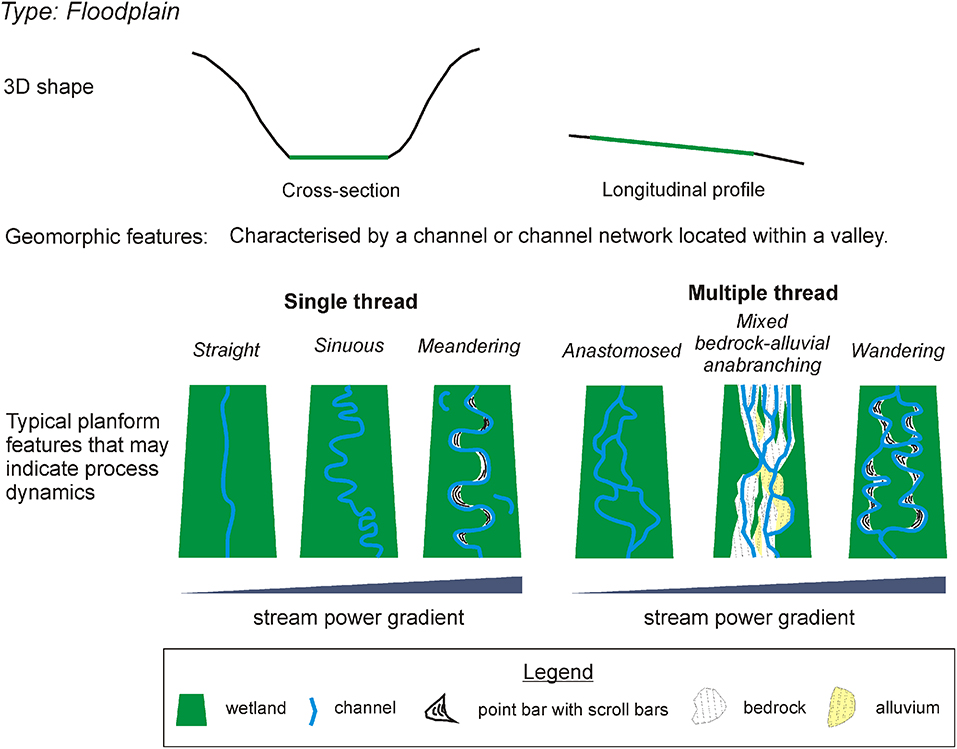
Figure 3. Schematic shape and geomorphic features that are used to classify floodplain wetland type, followed by typical planform features that are used to classify sub-type.
Channel pattern is indicative of the amount and size of bedload being transported, stream power, and channel stability (Kleinhans, 2010). As such, channel pattern should be considered along a morphological continuum that constantly evolves as conditions change. Large proportions of silt and clay increase floodplain cohesivity, and may therefore increase channel sinuosity. In contrast, as the proportion of fine sediment and vegetation is reduced, sinuosity decreases (Braudrick et al., 2009). The amount of sediment supplied to a channel, relative to stream power, may also have an effect on channel pattern; increasing sediment supply can result in braiding, whereas reducing supply can induce meandering (Church, 2006).
An increase in stream power relative to resisting forces of bed material calibre or bank material strength is typically associated with increased rates of lateral activity, such that for single thread channels, there is a continuum of form from low stream power (sinuous and straight, laterally inactive) to high stream power (meandering, laterally active; Nanson and Croke, 1992; Kleinhans and Van den Berg, 2011).
Anabranching rivers do not fit within low-energy meandering to high-energy braided planform continua (Kleinhans, 2010), and they generally have banks that are highly resistant to erosion, occur on extremely low gradients, and are often associated with mechanisms that block flow in the river channel, triggering avulsion (Nanson and Knighton, 1996). Nanson and Knighton (1996) divide anabranching rivers into six types, but for simplicity, we distinguish between three multiple-thread floodplain sub-types in this classification which may be distinguished by the degree of lateral activity, which is often indicative of dominant particle size and bank cohesivity.
Straight River Floodplain Wetland
Rivers that hold a straight planform for long distances are rare in nature, and tend to occur in headwater settings where valley confinement and supply of coarse material limit the efficacy of particle sorting processes, such that emergent alternate bars are not expressed in the planform (Parker, 1976; Kleinhans, 2010). Alternately, they may form where a channel has become set within the structural grain of an underlying fault, joint or fracture network (e.g., incision within the joint/fracture network of dolerite sills described by Tooth et al., 2002). Hydraulic instabilities in the flow, which are subsequently enhanced by alternate bar formation with sufficient sediment supply, tend otherwise to generate meandering (sand-dominated) or braided (gravel-dominated) channel patterns and associated floodplain features (Kleinhans, 2010). Riparian wetlands that flank straight channels are typically narrow, and derive from reworking of material transferred episodically through the system by hillslope-channel coupling (Harvey, 2001). Colluvial supply of material is therefore important, but the narrow floodplains that develop owe their origin to the way in which colluvial material is reworked by the river channel. An example of a straight river floodplain wetland is along the foothills zone of the Lotheni River, South Africa (29° 27′ 10.72″ S, 29° 31′ 45.43″ E).
Sinuous River Floodplain Wetland
Sinuous river floodplain channels are characterised by a lack of active lateral migration, and are therefore devoid of typical active meandering river floodplain features like scroll bars, ox bow lakes, or meander scars. The lack of active meander migration in these systems may either be associated with low stream power, floodplain factors such as dense vegetation or a large proportion of fine sediment in the banks and channel bed, or planform ossification due to backwater effects. The attainment of high sinuosity is possible through very slow processes of bank collapse. A key distinguishing feature of stable sinuous and active meandering river floodplains (section Meandering river floodplain wetland) is the relative dominance of vertical accretion (overbank deposits) over lateral accretion. An example of a sinuous river is described by Tooth and McCarthy (2004) for some channels of the Okavango Delta, Botswana.
Meandering River Floodplain Wetland
Meandering rivers are sinuous, laterally active, single thread channels (Figure 4). Depending on the rate of lateral migration and sediment supply, an alluvial ridge is produced that elevates the channel and leveés above the floodplain. The characteristic migration of the channel across the floodplain results in the development of point bars and scroll bars, while old channel locations may be marked by ox bow lakes. Lateral accretion deposits (stacked point bars) dominate the sedimentary fill of meandering river floodplains. Backswamp areas of the distal floodplain environments may be substantially wetter than the alluvial ridge due to elevation differences. While the majority of meandering river floodplain wetlands receive most of their water from overbank flooding of the channel, they may also receive lateral valley inputs from tributaries, hillslope runoff, and seepage. Meandering river floodplains are sites of sediment exchange; their ability to act as sites of sediment storage depends on a variety of factors such as tectonic setting, rate of lateral migration and frequency of overtopping.
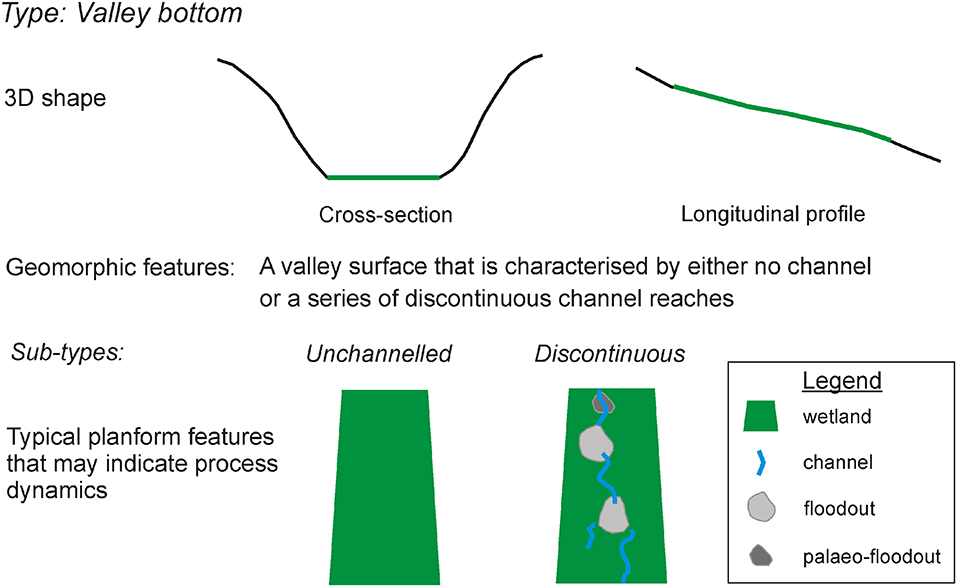
Figure 4. Schematic shape and geomorphic features that are used to classify valley-bottom wetland type, followed by typical planform features that are process indicators used to classify unchannelled and discontinuous wetland sub-type.
Key to meandering river dynamics is the integrity of lateral migration processes. River straightening, hardening or focussing flow through culverts can disrupt longitudinal sediment fluxes, resulting in channel incision and a reduction in overbank flooding. Excessive water abstraction either directly from or upstream of meandering river floodplains, such as for irrigation or mining, reduces stream power and may cause a meandering channel to switch from laterally active, to an inactive sinuous channel. Examples of meandering river floodplains include those of the Nsonge and Mfolozi rivers (Grenfell M. C. et al., 2009; Grenfell S. E. et al., 2009) and the Klip River (Tooth et al., 2002) in South Africa.
Anastomosed River Floodplain Wetland
Anastomosed rivers are characterised by low stream power, cohesive banks and low channel w/d ratios (Nanson and Croke, 1992). Channel sinuosity of individual branches varies. However, while occasionally highly sinuous, individual channel branches are laterally stable such that cut banks, point bars and oxbow lakes are generally absent (Makaske et al., 2002). Anastomosed floodplains typically occur where channel bed aggradation exceeds floodplain and leveé aggradation, driving channel avulsion (Nanson and Knighton, 1996; Jerolmack and Mohrig, 2007). In this classification system, an anastomosed river floodplain is akin to Nanson and Knighton (1996) type 1 or 2, where dominant sediment type can vary from mud to sand. An example of an anastomosing river floodplain is Cooper Creek in Australia (Gibling et al., 1998) and part of the Panhandle in the Okavango Delta, Botswana (Smith et al., 1997).
Wandering River Floodplain Wetland
A wandering river floodplain is characterised by multiple channels, typically 2–3, which are laterally active. In contrast to an anastomosing river floodplain, it is characterised by features of floodplain erosion and accretion (e.g., point bars, scroll bars, cut banks etc.) that are indicative of rapid lateral activity. They tend to have a higher stream power than anastomosing rivers, and in terms of sediment, they vary from mixed load to sand or gravel dominated (Nanson and Knighton, 1996). A wandering river floodplain encapsulates (Nanson and Knighton, 1996) type 3, 4 and occasionally type 5 floodplains. When gravel dominated, the channel pattern may vary from moderately braided to transitional between meandering and braided (Kleinhans and Van den Berg, 2011). Wandering river floodplains may either be the result of non-fluvial forcing (e.g., debris piles, reduction in flows) or disequilibrium dynamics (Kleinhans and Van den Berg, 2011). Many of the dry semi-arid to arid (UNEP, 1997 aridity index) South African floodplains are characterised by disequilibrium dynamics due to the impact of highly variable flows.
In dryland environments with variable flow regimes, these floodplains may support hydrophytic vegetation within the channel during periods of low or no flow. While the entire floodplain will be inundated during extreme flood events, certain types of wetland vegetation may withstand these floods to re-establish as flows are reduced. An example of a wandering river floodplain is the Touws River Floodplain in the semi-arid Karoo of South Africa (33° 37′ 48.62″ S, 20° 55′ 59.98″ E), and the Zambezi River Floodplain in Caprivi, Namibia.
Mixed Bedrock-Alluvial Anabranching Floodplain Wetland
This sub-type encompasses the ridge form and irregular sinuous anabranching rivers of Kleinhans and Van den Berg (2011), and is largely an outcome of episodically high stream power paired with resistant bedrock channel substrate and banks resulting in multiple channels. In addition to the occurrence of jointed/fractured granitoid outcrops which result in erosion resistant banks and islands, Tooth and McCarthy (2004) suggest that a variable flow regime may be an important factor in channel dynamics.
Channels are eroded preferentially along joints and fractures in the underlying bedrock. In order to accommodate variable discharge, multiple channels form around stable islands that may be composed of alluvium or bedrock. In low gradient reaches, sediment supply may exceed channel capacity, resulting in a surficial veneer of alluvial islands that becomes vegetated (Tooth and McCarthy, 2004), while small geomorphologically-transient palustrine wetlands may form in temporarily inactive channel branches. In steeper reaches, bedrock may outcrop more extensively as sediment transport capacity locally exceeds supply. An example of a mixed bedrock-alluvial anabranching floodplain wetland is that of the Orange River in the vicinity of Eksteenskuil, South Africa (Tooth and McCarthy, 2004).
Valley-Bottom Wetlands
Valley-bottom wetlands are those that occur on valley floors where a continuous channel is absent (Table 4, Figure 4). They are typically well-vegetated, fairly flat in cross-section and often perpendicularly abut large floodplain systems which act as a local base level control on incision. Valley-bottom wetlands are typically steeper and have smaller catchments than floodplain wetlands (Ellery et al., 2016). We discern two main sub-types; an unchannelled valley-bottom and a discontinuous valley-bottom. Geomorphic processes of the latter are better documented, and in general, an improved understanding of the development of unchannelled valley-bottoms is required.
In the southern parts of the Western and Eastern Cape of South Africa, where valley-bottom wetlands have formed due to repeated cycles of cutting and filling (Pulley et al., 2018), the gradual release of groundwater onto the valley floor from adjacent highly folded and fractured mountain landscapes sustains low flows along the valley floor (Tanner et al., 2018). Given this pattern of inputs, flows are sustained but typically low, with low stream power, such that the valley-bottom wetland remains wet for long periods.
Discontinuous Valley-Bottom Wetlands
Discontinuous valley-bottom wetlands are characterised by short reaches of stream channel, separated by patches of aggradation in floodout features. However, floodout features may not always be very well-developed, or may not be visible due to vegetation cover. The channel is deepest at the wetland head, where it may be actively eroding, and becomes progressively shallower downstream until it loses confinement completely and deposits sediment on a prograding fan. Sediment deposited on the floodout fan fines toward the distal reaches. Channels tend to reform toward the base of the fan due to localised steepening. In some cases, tributary streams may locally increase capacity and competence, resulting in reforming channel initiation (e.g., Nylsvlei, McCarthy et al., 2011). Due to the complexity of the local topography, the hydroperiod of such wetlands is varied, with the wetland close to the floodout apex generally experiencing the wettest conditions. Frequency of inundation depends on local rainfall patterns as groundwater contributions, except from lateral seepage, are usually limited.
Discontinuous valley-bottoms have been comprehensively described in a variety of climatic settings. In some settings the formation of fans appears to be linked to the localised effect of resistant lithology, while in others, no geological control was found (Grenfell M. C. et al., 2009; Grenfell et al., 2012, 2014). There is evidence to suggest that at millennial timescales, sediment fill in some valleys is almost completely evacuated by gully incision and widening. In Australia, Fryirs and Brierley (1998) documented three phases of erosion and two phases of infilling in just 6,000 years, suggesting that these wetlands are particularly dynamic. The landscape in such a system may be considered to have two alternate transient states. In one state, the valley is completely eroded with no sediment infill and during this phase, the bedrock valley floor is eroded. In the second state, ongoing erosion upstream produces a pulse of sediment that accumulates on the valley floor, eventually producing a substantial valley fill upon which localised streams and floodouts slowly disperse sediment downstream. It is not known over what time scales these processes naturally operate in different dryland environments, although it is known that human settlement can accelerate incisional processes (Brierley and Fryirs, 1999).
This process was also documented in wetlands dominated by Prionium serratum (Palmiet), where it was found that successive phases of gully incision were initiated in response to gradual steepening by aggradation, and led to a reduction of valley slope (Pulley et al., 2018). In the case of discontinuous valley-bottom wetlands, intrinsic controls on slope and patterns of sedimentation force the system to evolve toward a threshold. Despite evidence for self-organised criticality, these systems are extremely vulnerable to human induced changes to catchment water and sediment supply, typically created through changes in land use or invasion of alien plants (Rebelo et al., 2015). When restoring such wetlands, it is essential that the original cause of change to catchment patterns of sediment and water supply is addressed. Furthermore, interventions must be sensitive to lateral and longitudinal sediment connectivity, as preventing the downstream migration of sediment may cause erosion elsewhere.
Unchannelled Valley-Bottom Wetlands
Unchannelled valley-bottom wetlands are typically well-vegetated wetlands located on the valley floor, characterised by a lack of surficial geomorphic features. Flow across the wetland is diffuse, although there may be localised flow paths within or even underneath vegetation. The lack of channel development, despite a steeper valley slope than most floodplains, is likely related to low stream power, which may be further reduced by the effect of dense vegetation. These wetlands are typically permanent or seasonal, with temporary zones located laterally along the valley side. Water inputs are derived from rainfall, upstream channels and lateral and longitudinal seepage, whether from inter-flow or groundwater sources.
Care should be taken when classifying unchannelled valley-bottom wetlands, as they may actually be blocked-valley wetlands, especially when abutting a large alluvial floodplain, or they may be geochemical depressions. A true unchannelled valley-bottom wetland would be longitudinally connected to downstream systems, and would not be a depression.
As unchannelled valley-bottom wetlands are connected to the drainage network, they must undergo phases of erosion such that the valley floor may be constantly lowered (e.g., Pulley et al., 2018). In terms of long-term geomorphic processes, they must occasionally undergo phases of incision similar to those of discontinuous valley-bottom wetlands. The main contrast appears to be related to local processes of sedimentation. Within unchannelled valley-bottom wetlands, sediment supply appears to be extremely low. Incoming sediment is filtered evenly across the wetland, resulting in a homogenous wetland surface. The lack of localised aggradation processes prevents local steepening and channel formation, allowing patterns of diffuse flow to be preserved. Nevertheless, extremely slow sediment accretion that occurs preferentially toward the wetland head results in gradual steepening of the wetland, until a critical threshold is reached. Under natural conditions, it can be assumed that the complete cycle of aggradation and incision must be slower than that of discontinuous valley-bottom wetlands, where the turnover rate is higher.
These wetlands are extremely vulnerable to interventions that focus flow patterns (e.g., road culverts) and locally increase stream power, causing erosion. In addition, changes to catchment land use may alter water and sediment supply to the wetland, potentially enhancing processes of aggradation (due to increased sediment supply) or causing erosion (due to an increase in peak run off).
Blocked-Valley Wetlands
Blocked-valley wetlands occur along valley floor reaches and can be fairly extensive longitudinally (Figure 5). They are formed along drainage lines where rates of aggradation on one confluent channel exceed that of the other confluent channel, leading to valley impoundment, the impediment of water and sediment flows, and lake or wetland formation. Blocked-valley wetlands may form in tributary or trunk valleys, but in either case the confluent channel driving impoundment has a higher sediment load. This may be due to a larger catchment area, discharge and sediment supply, as is the case when a tributary is blocked by the trunk channel (e.g., Grenfell et al., 2010; Ellery et al., 2012). In other cases the larger sediment load may be attributed to differences in land use, land cover, soil, geology or slope (e.g., Joubert and Ellery, 2013).
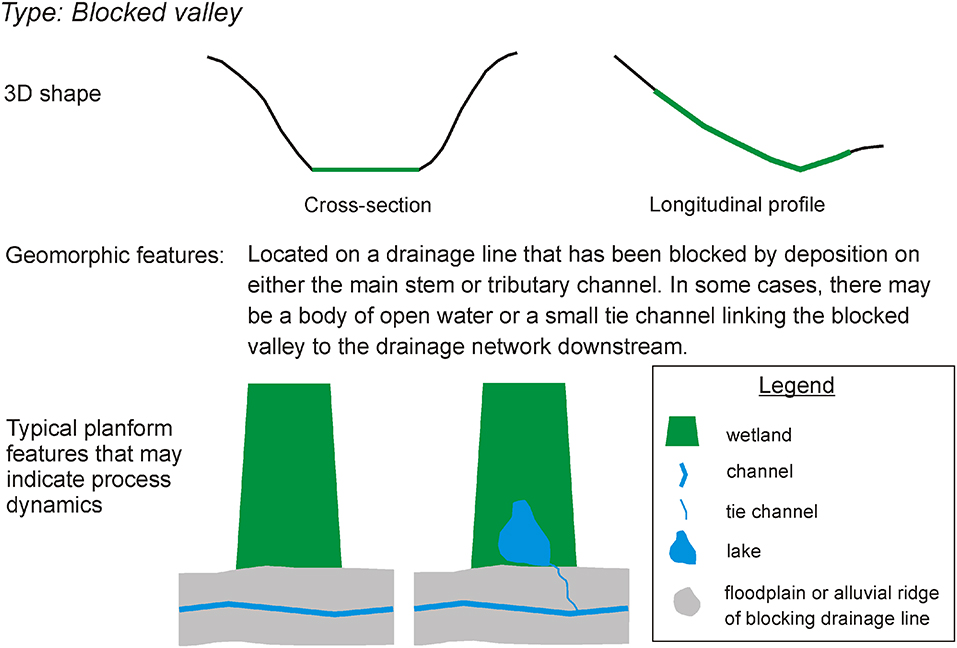
Figure 5. Schematic shape and geomorphic features that are used to classify blocked-valley wetland type, followed by typical planform features that may or may not occur.
Due to their specific landscape setting, blocked-valley wetlands are characterised by particular patterns of sediment accumulation and hydroperiod (Grenfell et al., 2008, 2010). As stream power is reduced by a rising local base level, sediment begins to accumulate in the depression. Sediment typically fines downstream and if the catchment is sufficiently wet, peat may accumulate in the lowermost area of the valley if it is permanently waterlogged (Ellery et al., 2012). Depending on the relative difference in aggradation rate as well as the local climate, the resulting depression may have an area of open water which is too deep for colonisation by emergent macrophytes. Blocked-valley wetlands and lakes occur in dryland settings as well as more humid environments, as typified by Lake Murray on the Strickland River in Papua New Guinea. These wetlands are vulnerable to rapid infilling due to a change in upstream catchment activities (e.g., removal of native vegetation), as well as increases in abstraction of water in the catchment (e.g., plantations) and from the wetland itself for irrigation.
Wetland Plain
Wetland plains, described by Ollis et al. (2015) as “flats,” are extensive systems with no distinct local topography (Figure 6) that typically form on coastal plains. Due to the extremely low gradient, flow is diffuse, and processes of sediment transport are considered minimal. As they are not linked to stream flow, and are not fed by local catchments, water is primarily derived from local rainfall although there is potential for rising primary aquifer levels to locally intersect the land surface. In terms of geomorphic features, wetland flats may be characterised by isolated depressions. There have been no significant publications on geomorphic characteristics of wetland flats in southern Africa, and this description remains largely untested. Wetland plains often occur as part of a complex mosaic of wetlands that include floodplains, fans, depressions, and valley-bottom wetlands. It is likely that due to climatic constraints, wetland plains in drylands are highly variable in extent, and have largely seasonal or temporary hydroperiods. Portions of the Agulhas wetland system surrounding Prinskraal, Western Cape, South Africa are an example of alluvial wetland plains (see Figure 1).
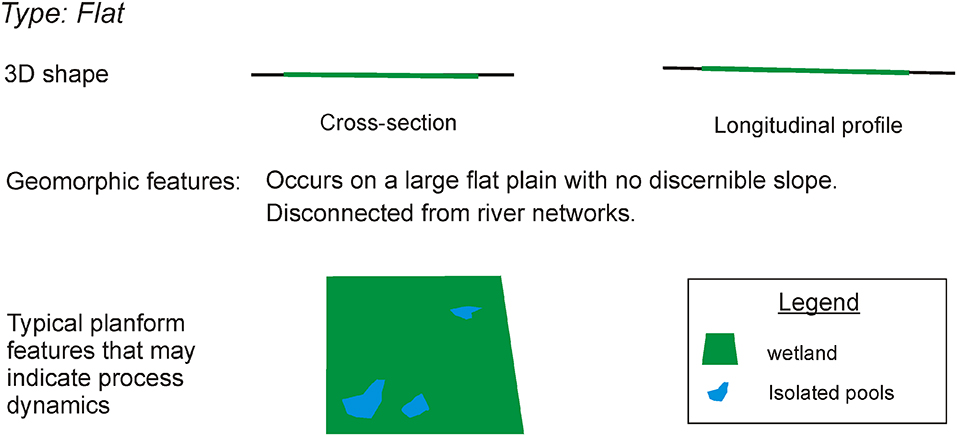
Figure 6. Schematic shape and geomorphic features used to classify a flat type wetland, followed by typical planform features.
Wetland Fan
Alluvial fans form at locations of loss of confinement as a stream discharges onto a receiving basin of very low gradient. Alluvial fans are well-known in arid environments as being generally devoid of hydrophytic vegetation, although the Okavango Delta is strictly an alluvial fan with a large area that is permanently flooded and densely vegetated (McCarthy et al., 1988, 1997, 2002; Stanistreet et al., 1993). In the case of the Okavango Delta, a large river drains a catchment with high rainfall and discharges into a rift valley basin oriented perpendicular to the watercourse in a semi-arid setting (rainfall < 500 mm.a−1). Wetland alluvial fans are fairly common in dry sub-humid and semi-arid areas of South Africa, and vary quite substantially in terms of size. They may also form in a variety of landform contexts, including valleys, plains or hillslopes. Fans are conical shaped sediment deposits that occur when a stream loses confinement at the apex, resulting in adjustments to stream width-depth ratio and concomitant deposition (Figure 7). Loss of confinement is generally controlled by geological factors, such as rifting (McCarthy et al., 1997) or a change in lithology, or may simply be due to a landform transition such as confined tributary valley to unconfined trunk floodplain.
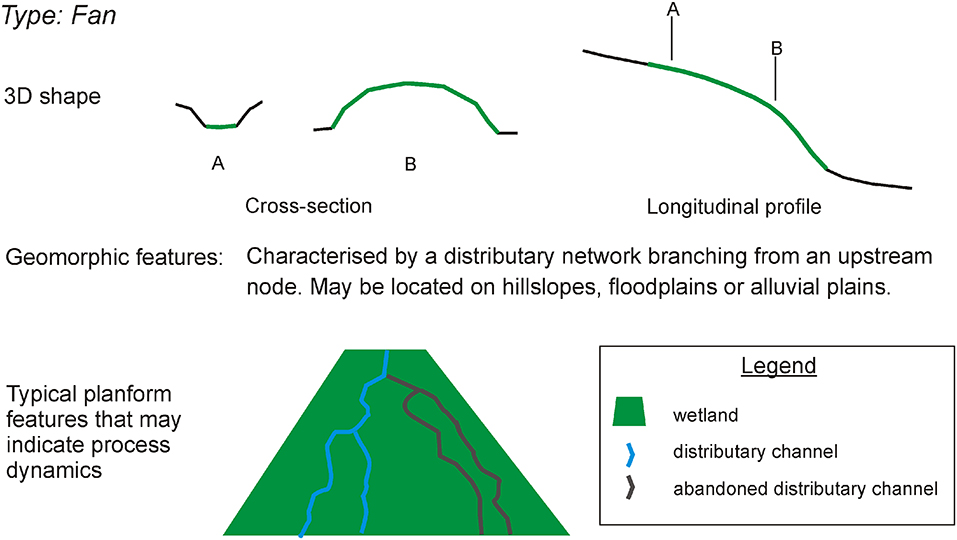
Figure 7. Schematic shape and geomorphic features that are used to classify a fan type wetland, followed by typical planform features that may or may not occur.
Unlike a discontinuous valley-bottom wetland, fans are standalone features and are not associated with a cascading system of floodouts and reforming channels. However, they are similar to floodouts in discontinuous valley-bottom wetlands in terms of sedimentology and hydroperiod. Fans are characterised by sediment fining from the apex to distal reaches, and have a network of distributary channels, some of which may be abandoned and some of which may be morphodynamically active. Fans are dependent on surface water and sediment inputs, and if deprived of these the wetlands may become incised and desiccate. Depending on climatic and/or inflow regime, fans may be permanently wet or, in more arid environments, seasonally or temporarily wet (e.g., Grenfell et al., 2012).
Aeolian Wetlands
Aeolian Depression Wetlands
Aeolian depressions, frequently called pans, form in paleaolacustrine basins, and on palaeodrainage lines, interdunes, and coastal plains (Goudie and Wells, 1995). For pans to persist, the setting of formation must be largely isolated from current fluvial activity, while aeolian sediment sources and fluxes should not be so overwhelming that hollows are rapidly infilled. Most pans are not a product of aeolian deflation alone, but also require the action of salt weathering (Goudie and Wells, 1995).
The majority of depressions that are not located in dune fields form on lithologies that are vulnerable to salt weathering. In general, rocks formed in marine environments are more susceptible as weathering liberates salts which may then exacerbate rock disintegration by crystallisation and hydration. Once the rock has been weathered the individual grains that have been liberated are removed by wind erosion. In southern Africa, animals using the resultant pan as a waterhole can enhance deflation processes (Marshall and Harmse, 1992).
Pans are typically ovoid or cuspate in shape, with a lunette dune downwind of the pan. In areas where salt weathering is important, the pan is typically only seasonally or intermittently inundated. Hydrological inputs are usually derived directly from precipitation. Aeolian depressions are vulnerable to changes in local land use that increase sediment availability and result in infilling. An example of typical aeolian depressions are those of the Northern Cape pan belt, South Africa (Goudie and Wells, 1995).
Geochemical Wetlands
Geochemical Depression Wetlands
Geochemical depression wetlands typically form in valley or plain settings where permanent saturation of underlying bedrock chemically alters and simplifies the mineralogical composition, resulting in a loss of mass and volume. Geochemical depressions may be divided into four sub-types; subsidence depressions formed on igneous rocks, redox depressions formed by redox reactions as a result of repeated cycles of wetting and drying, dissolution depressions formed on karstic rocks such as limestone or dolomite and margin-aggradation depressions formed by precipitation of allochthonously introduced solutes in the pan margin (Table 5). While karstic depression wetlands are dissolution features, igneous depression wetlands are features of hydrolysis, resulting in different characteristic geomorphic and hydrological features. Based on the Ollis et al. (2015) hydrogeomorphic classification, many geochemical depression wetlands would be classified as valley-bottom wetlands due to their appearance in aerial photography. However, their underlying stratigraphy is entirely produced by chemical weathering of bedrock rather than by reworked fluvial sediment, typically covered by a superficial peat deposit if local hydroclimatic conditions allow. Geochemical depression wetlands are particularly vulnerable to changes in catchment hydrology (e.g., increased run-off, reduced infiltration) as saturation is often fundamental to the geochemical processes required for their formation.
Subsidence Depression Wetlands
Subsidence depression wetlands are generally formed where the underlying bedrock is igneous and has a substantial proportion of feldspar, although they have been recorded on tillite with a high proportion of feldspar. When permanently saturated with slightly acidic water, feldspar minerals are simplified by the loss of metals such as iron, calcium, magnesium, and sodium to form clay minerals such as kaolin. In the case of Edwards et al. (2016), feldspar minerals in a dolerite sill were transformed through hydrolysis to kaolin. The in situ formation of saprolite results in surface sagging, which creates a basin and promotes inundation and wetland formation. Prolonging inundation acts as a positive feedback, as saturation enhances hydrolysis.
These wetlands are surprisingly common on ancient land surfaces such as the African erosion surface in southern Africa, which has weathered for in excess of 60 million years (Holmes and Meadows, 2012). This suggests that prolonged weathering is required for their formation, which is likely to be in the order of 1 mm per 10,000–20,000 years (Edwards et al., 2016). These systems vary considerably in inundation duration, from being permanently to seasonally or ephemerally inundated. In the case of Edwards et al. (2016), prolonged saturation occurred following development of a meandering river floodplain on resistant dolerite. The resultant wetland is therefore characterised by meandering river features such as abandoned meanders. However, due to internal sagging, the river is no longer laterally active. Igneous depression wetlands may therefore have characteristics of depression wetlands (e.g., isolated water bodies) as well as relic features of old landscape processes (e.g., channels). Water inputs are dominated by surface channel flows and diffuse runoff, although seepage might be locally important. Due to the formation of a depression, most of the incoming sediment may be trapped within the wetland during low or mean flows.
Redox Depression Wetlands
In contrast to subsidence depressions, redox depressions are formed in seasonal climates, where there are repeated cycles of wetting and drying. During the wet season, Fe3+ is converted to the more soluble ion Fe2+ by reduction of the underlying soil and bedrock in anaerobic conditions. Vegetation transpiration on the depression edges draws water toward the depression margins, moving the soluble Fe2+ away from the depression centre. During the dry season, the wetland is exposed to oxidising conditions in an aerobic environment. This transforms the ions to insoluble Fe3+, which then comes out of solution on the depression margins, where it builds local relief. Over a prolonged period of time, repeated cycles of wetting and drying result in a loss of volume in the centre of the depression, with concomitant aggradation on the margins.
This process may be fairly common on ancient erosion surfaces that have been exposed to weathering processes for long periods of time. First described by Ellery (2019) for Shadow Vlei, in the Eastern Cape of South Africa, the resultant depression is surrounded by a ferricrete rim that is slightly elevated above the surrounding landscape. The same process can also result in the development of calcrete rims (Alistoun, 2014).
Margin-Aggradation Depression Wetlands
In contrast to redox depression wetlands, the soluble ions responsible for relief building in margin-aggradation depression wetlands are not derived in situ, but are supplied allochthonously by circulating waters rich in solutes. The archetypal example of a margin-aggradation depression is described by McCarthy et al. (1993) for the interior of alluvial islands on the Okavango Delta. Large, deep-rooted trees grow preferentially on the island margins, and are responsible for increasing groundwater salinity, leading to saturation of calcite and silica, by transpiration. Sub-surface saturation results in precipitation of calcite and amorphous silica, leading to vertical expansion on island margins. The creation of topographic relief on the margin creates a central depression, characterised by sparse, salinity tolerant vegetation. On older islands, vegetation in the depression may be replaced with sodium carbonate deposits. In contrast to the long time frames required for the formation of subsidence and redox depressions, numerical modelling suggests that salinisation of the groundwater occurs over timespans between 100 and 200 years, but that aggradation of island margins takes place over much longer timespans. The formation of margin-aggradation depression wetlands requires specific hydroclimatic conditions, such as low annual precipitation to allow local saturation of groundwater, as well as reliable surface water inflows.
Dissolution Depression Wetlands
Dissolution depression wetlands occur in areas underlain by water soluble and porous carbonate rocks and are synonymous with typical karst landscape features such as swallow holes, disappearing streams and solution sinkholes. Wolfe (1996) described two types of dissolution wetlands of karst landscapes, karst pans and compound sinks, which differ in terms of their degree of connection to the groundwater drainage system. Karst pans are very shallow with no direct connection to groundwater. They are formed due to subsidence as the underlying calcareous rocks are dissolved. In some cases, they may be lined with clay, effectively sealing the base of the wetland to groundwater losses (Greear, 1967). In contrast, compound sinks are deeper and linked to the underground drainage network. As a result, sources and patterns of water inputs to karst depressions are highly varied. O'Driscoll and Parizek (2003) found that sinkhole wetlands in Pennsylvania, USA were fed primarily by direct precipitation as well as a perched groundwater table. As a result, dissolution depression wetlands may be seasonal (e.g., Kobza et al., 2004) or permanently inundated. For simplicity, we do not further subdivide dissolution depression wetlands. Dissolution features and the resultant ephemeral wetlands similar to those described above have been described in the Namib by Marker (1982).
Discussion
While the classification is based primarily on research from southern African wetlands, it is clear from the large and growing literature on wetlands in drylands across the globe (see further examples from Africa, Australia and southwest USA in Lisenby et al., 2019) that many of the processes described occur in other dryland environments, as well as in humid environments where wetlands are created by process-form feedbacks. The classification is physics-based and determined by processes of sediment deposition and erosion which apply globally, and it is therefore anticipated that the classification approach presented may be more widely applied and expanded than currently detailed.
Wetland types that have not previously been defined with reference to a clear process-based mode of formation have been excluded (e.g., channelled valley-bottom wetlands, where there is no compelling geomorphic reason to distinguish these features from floodplain wetlands at a high level in classification; Nanson and Croke, 1992), and where necessary, sub-types have been provided such that wetland forming processes may be better contextualised and understood. The advantage of this classification system is that all modes of dryland wetland formation identified in the literature are included. As research advances, additional wetland types may be added in accordance with the discriminatory framework developed. The implications of the genetic classification system for understanding spatial and temporal variability in form and process of wetlands in drylands are discussed in the sections that follow.
Fluvial Wetlands and Alternate Transient States
Dryland wetlands are highly dynamic ecosystems, developing under extreme climatic conditions that are often characterised by alternating phases of floods and droughts. This, in combination with their frequent occurrence on drainage lines, results in wetlands potentially shifting between alternate stable states (e.g., Rountree et al., 2001). While some wetlands may be quite resilient to environmental change (Tooth, 2018), geomorphic processes in others result in alternation from one state to another. When classifying wetlands and developing catchment management plans, it is useful to be aware of the different forms that a wetland may take over time, and to consider the temporal scale at which such change takes place.
Over short time scales (seasons to years), if we consider a non-perennial river that flows seasonally or intermittently, during periods of no flow the river becomes akin to a discontinuous valley-bottom wetland with a series of disconnected pools and reed beds. However, during flood events, the entire floodplain may become a macro channel for flow. There are thus two alternate transient states for this system depending on flow conditions.
On a geomorphological time scale (centuries to millennia), two prevailing geomorphic misconceptions (Schumm, 1994) are frequently applied to dryland wetlands, potentially because they are often viewed exclusively as ecosystems rather than also as geomorphic systems. For many ecologists, wetlands represent a stable habitat that supports obligate species that are adapted to that environment. The assumption is that the particular biophysical conditions that support those species have existed for a very long time. Thus, for many wetland scientists, there is a perception of stability, which supports an underlying view that any adjustment to a wetland ecosystem must have been caused by humans. However, when we consider valley-bottom wetlands as geomorphologists, it is clear that for a drainage line to exist, it cannot always have been a site of aggradation.
Contrastingly, once a wetland has entered a state of erosion, whether anthropogenically-forced or otherwise, there is a perception that the adjustment will never cease, and that the wetland will not recover unless intervention occurs. This is (Schumm, 1979) misperception of instability. However, cut and fill landscapes are driven by geomorphic threshold behaviour, where self-reinforcing, continuous but gradual changes (e.g., in longitudinal slope, associated with ongoing aggradation) force an abrupt switch in system behaviour (to incision; Ellery et al., 2016). Aggradational processes within valley-bottom wetlands result in oversteepening over the long term, and once a geomorphic threshold is reached, they begin to erode. When the gullies are completely connected, the valley fill is completely or partially evacuated. The sudden availability of sediment overwhelms the drainage line downstream, causing aggradation, the effects of which cascade up- and downstream (Figure 8). The valley-bottom wetland therefore oscillates between gullied and wetland states (Grenfell M. C. et al., 2009; Ellery et al., 2016; Pulley et al., 2018). While natural aggradation can force the system toward a critical threshold, external factors that enhance aggradation within the wetland, or increase the frequency and magnitude of catchment runoff supply, can artificially accelerate the process.
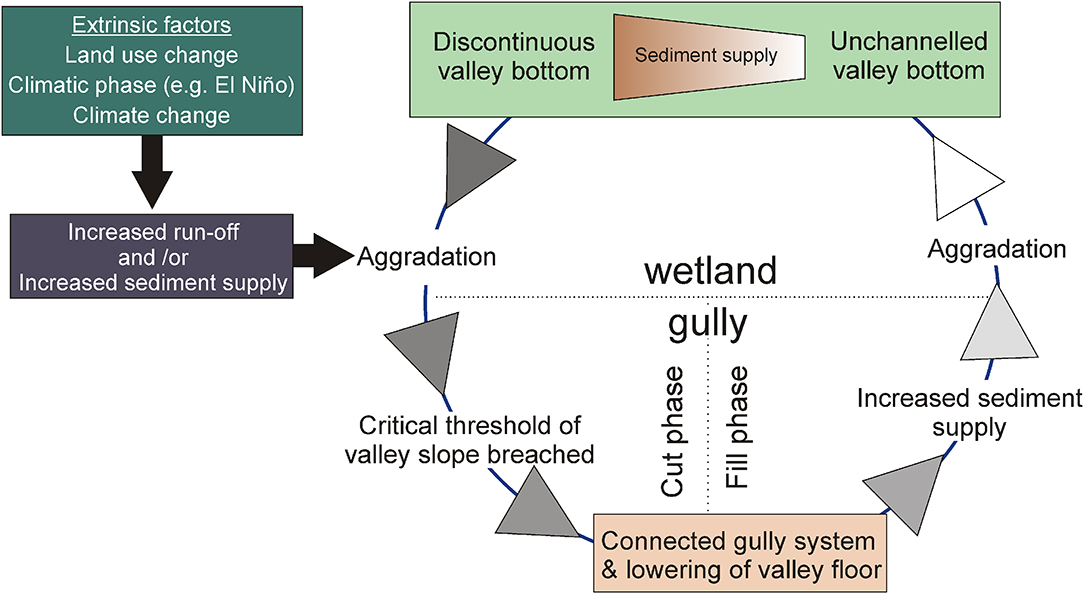
Figure 8. In an example of self-organised criticality, aggradation in valley-bottom wetlands results in valley steepening. Ongoing aggradation eventually results in incision once a particular valley slope threshold is breached. The release of valley fill sediment through erosion causes aggradation downstream, eventually resulting in the formation of new valley-bottom wetlands. Whether the wetland is a discontinuous or unchannelled valley-bottom wetland may be related to catchment sediment supply during the fill phase, with discontinuous systems being characterised by a larger sediment supply.
Integrating Spatial and Temporal Process Scales
Due to the wide variety of spatial scales at which geomorphic processes occur, it is possible for wetland types to be spatially nested within each other. This does not undermine the concept that it is the mode of formation of each wetland that is important, but rather supports the system-scale view that processes may operate at different spatial scales (Fryirs et al., 2018). For example, the Okavango Delta is a large wetland system that has formed on a graben structure in Botswana. The system as a whole may be classified as an alluvial fan as it has formed as a result of loss of confinement (Figure 9A). However, if we consider the wetland at finer resolution, we may also characterise different types of floodplain which have formed due to variations in flow and sediment transport (Figures 9B,C). On the distal reaches of the alluvial fan, geomorphic processes are extremely different, and geochemical depression wetlands occur due to evapotranspiration and subsequent solute enrichment (Figure 9D). Understanding and illustrating the nested hierarchies of process and form that characterise many large wetland systems provides essential insight into wetland structure, function and dynamics, but is also an essential tool for communicating this insight in management initiatives (Dollar et al., 2007; Fryirs et al., 2018).
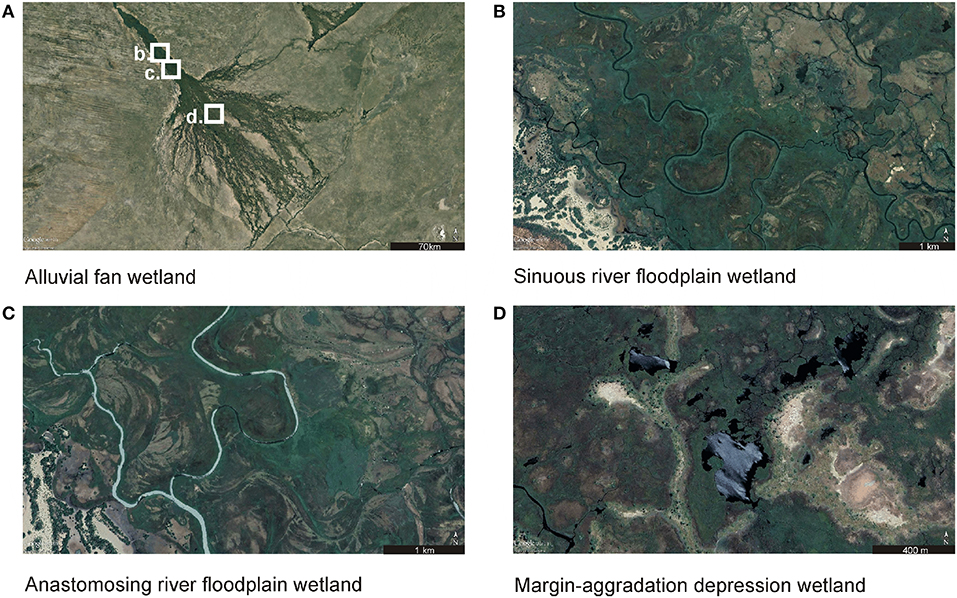
Figure 9. Wetland types and their associated processes and dynamics may be nested. For example, the Okavango Delta is an alluvial fan in a tectonic setting (A). On the panhandle, different types of floodplain wetlands occur due to downstream variation in sediment supply and discharge regime (B,C). In the fan region, tree-fringed geochemical depressions occur (D). All images from Google Earth.
Similarly, geomorphic processes may operate at varied temporal scales. In floodplains, an individual flood event may shape an in-channel bar or set of bars, while long term channel migration and development of an alluvial ridge may occur within decades or centuries (Figure 10). Geomorphic diversity and variation in wetland habitat in such systems arises over time through the interplay of processes that occur relatively gradually and continuously (e.g., channel lateral migration toward a backswamp environment), and processes that occur abruptly, though perhaps after a lengthy period of initial development (e.g., intersection of meander necks forming an oxbow lake, or avulsion following headward incision that intersects a low point in the channel upstream). Morphological changes in alluvial fans may also be fairly abrupt, with channels switching course following natural aggradation. Where material fluxes are more diffuse, geomorphic processes tend to occur more slowly. For instance, many aeolian and geochemical depressions in southern Africa are located on ancient erosional surfaces. The formation of these wetlands potentially takes place over tens-to-hundreds of thousands of years. Understanding the typical timescales over which different features within wetlands are formed is critical to our interpretations of change, and to distinguishing natural changes from those that may have been anthropogenically accelerated. It is also critical to understanding the natural recovery potential of wetlands, in determining the time required for natural recovery to progress in a particular geomorphic setting if/once the cause of degradation has been removed.
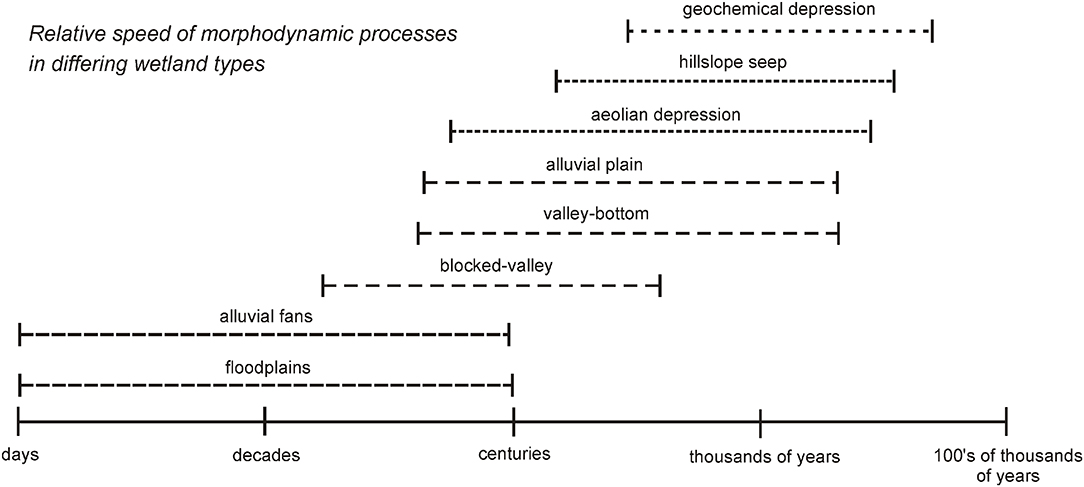
Figure 10. A comparison of relative speed of typical morphodynamic processes in different wetland types.
Implications for Understanding Geomorphology, Sediment Fluxes, and Ecosystem Service Provision
A re-envisioning of what constitutes a functional wetland unit and acknowledgment of system-scale linkages between wetlands and catchments is essential for successful wetland restoration in dryland environments. In the genetic classification system presented here, the wetland is an integrated system with characteristic local fluxes of water and sediment. A wetland classified according to its mode of formation may encompass several hydrological zones, although the wetland type and sub-type may provide an indicator of the typical spatial arrangement and temporality of those zones. This is in contrast to Semeniuk and Semeniuk (1995) and Ollis et al. (2015), where the functional unit is a combination of a hydrogeomorphic descriptor or type and the hydrological regime. The main aim of the genetic classification system is to provide insight into characteristic patterns of landform development, which generally arise through sediment entrainment, transport and deposition.
For dryland wetlands, understanding the manner in which sediment moves through the landscape is essential. This often requires a catchment scale understanding of sources of sediment supply, and the likely impact of changes in land use or treatment. Changes in run-off, either through physical abstraction, land use change or alien plant infestation, may also influence river capacity and competence, either increasing it if flood peaks are enhanced, or reducing it if abstraction rates are high. The proposed genetic classification of wetlands acknowledges that the maintenance of sediment supply may be as important as water supply in these wetlands, and is essential for maintaining biodiversity and ecological processes (Wohl et al., 2015).
Processes of sediment transport and deposition, their magnitude, and their temporal and spatial scale have implications for two tasks common to wetland practitioners; (a) planning of wetland restoration projects, and (b) assessments of wetland ecosystem services. When considering the rehabilitation of an eroded wetland at the functional unit scale that has previously been used in South Africa, it is perhaps easy to overlook geomorphic processes that occur throughout a drainage line, and which are vital for maintaining and creating wetlands downstream. At a local scale, preventing further headward erosion and encouraging the deposition of sediment is useful as it allows wetland processes on site to be maintained. However, for downstream systems, dependent on an upstream sediment supply, artificially reducing longitudinal connectivity (i.e., through a weir or other barrier to sediment movement) can be catastrophic (e.g., Kondolf et al., 2006). In a wetland that is actively aggrading, such as in some floodplains and all valley-bottom wetlands, a reduction in sediment supply may result in erosion, effectively reversing the system into a cutting cycle. It is vital that drainage line wetlands are considered holistically in terms of both water and sediment connectivity such that meeting the immediate aims of local restoration does not cause harm downstream in the future. Even in systems which have been subject to aggradation as a result of anthropogenically-enhanced catchment sediment supply, have crossed a threshold slope (Ellery et al., 2016), and are undergoing incision, it must be acknowledged that this represents a system level reorganisation of material that will in time self-organise wetland development through the drainage network. In this case, the forced retention of sediment at discrete points in the network using engineering structures is in fact working against these natural processes. Greater attention should be paid to identifying the cause of degradation, and the persistence of degrading forces within the system, so that restoration efforts can work with natural processes to enhance natural recovery.
Since the genetic classification system for palustrine wetlands in dryland settings offers an improved conceptualisation of sediment movement, it is anticipated that there will be an improvement in understanding of the provision of ecosystem services, especially with regards to services associated with sediment trapping, assimilation of nutrients, and the removal of contaminants and toxicants. For instance, floodplain wetlands are often considered excellent at sediment trapping (e.g., Kotze et al., 2009), but for many dryland wetlands, inundation of the floodplain due to overbank flooding may be infrequent. As such, in dry years, floodplain wetlands which experience some lateral migration but limited overbank connectivity are likely to be net exporters of sediment. Furthermore, the vertical growth (i.e., depth of sediment storage) of the floodplain is limited by processes that can result in a net return of sediment from the floodplain to the channel (described by Lauer and Parker, 2008), either through scour during extreme floods, bank slumping or meander migration. Further research is required in dryland floodplain settings to determine actual rates of sediment trapping efficiency. In contrast, blocked-valley wetlands are extremely effective at trapping sediment and associated contaminants, as the entire sediment supply is likely to be trapped, while water may still seep down valley. The classification system also offers an enhanced understanding of sorting processes that lead to spatial variation in sediment and nutrient dispersal within wetlands. Nutrient/toxicant uptake in wetlands (especially the sediment associated/adsorbed component) depends as much on their input spatial distribution, as it does on the biogeochemistry of plant or bacterial uptake processes, and this input spatial distribution is conditioned by advective and diffusive geomorphic transport processes (e.g., material fining with increasing distance from a channel margin or terminus).
Conclusion
The proposed genetic classification system offers a complementary approach to several existing vegetation-based or hydrogeomorphic classification systems when applied for the purpose of wetland management or rehabilitation planning. The classification structure lends itself to additions that could expand the classification globally and ensures that when applied consistently, a wetland may fall into only one class. However, it is flexible in that when considered at increasing resolution, additional wetland types may be nested within larger systems.
The main conceptual difference from existing classification systems is that the characteristics of the sediment flux are explicitly included, which results in a classification system that is truly responsive and inclusive of geomorphic processes responsible for the formation and ongoing existence of wetlands in drylands. It encourages holistic consideration of wetland systems and recognises that fluxes in sediment and water occur both longitudinally and laterally within wetlands, and that this is important for the long-term viability of wetlands when comparing management or intervention options.
Furthermore, by more accurately detailing geomorphic processes occurring within wetlands, our ability to predict changes associated with altering sediment or water flux in the catchment and within the wetland is improved. It is anticipated that application of the genetic classification approach will encourage a landscape approach to wetland management, where wetlands are understood to be components of an integrated catchment. This is especially important in dryland settings as the frequent occurrence of wetlands on drainage lines makes them vulnerable to changes in catchment land use that impact upon downstream flood peaks and/or sediment supply. It is hoped that use of the genetic classification system will provide information required for the development of catchment management plans that are suited to the range of wetlands occurring in the landscape, as well as restoration strategies that are sensitive to geomorphic processes.
Author Contributions
SG conceived and developed the classification system and wrote the paper. MG and WE contributed to the classification system and co-wrote the paper. NJ contributed toward the hillslope seeps section of the paper. DW critiqued the classification system and contributed to writing of the paper.
Funding
This research of SG was supported in part by the National Research Foundation of South Africa (Grant number: 118603).
Conflict of Interest
The authors declare that the research was conducted in the absence of any commercial or financial relationships that could be construed as a potential conflict of interest.
References
Aalto, R., Maurice-Bourgoin, L., Dunne, T., Montgomery, D. R., Nittrouer, C. A., and Guyot, J. L. (2003). Episodic sediment accumulation on Amazonian flood plains influenced by El Nino/Southern Oscillation. Nature 425, 493–497. doi: 10.1038/nature02002
Alberta Environment and Sustainable Resource Development (2015). Albert Wetland Classification System. Edmonton, AB: Water Policy Branch, Policy and Planning Division.
Alistoun, J. (2014). The origin of endorheic pans on the African erosion surface north of Grahamstown, South Africa (MSc thesis). Grahamstown: Rhodes University.
Braudrick, C. A., Dietrich, W. E., Leverich, G. T., and Sklar, L. S. (2009). Experimental evidence for the conditions necessary to sustain meandering in coarse-bedded rivers. Proc. Natl. Acad. Sci. U.S.A. 106, 16936–16941. doi: 10.1073/pnas.0909417106
Brierley, G. J., and Fryirs, K. (1999). Tributary–trunk stream relations in a cut-and-fill landscape: a case study from Wolumla catchment, New South Wales, Australia. Geomorphology 28, 61–73. doi: 10.1016/S0169-555X(98)00103-2
Brinson, M. M. (1993). “A hydrogeomorphic classification for wetlands,” in Wetlands Research Program Technical Report WRP-DE-4 (Greenville, SC: US Army Corps of Engineers; East Carolina University).
Buffington, J. M., and Montgomery, D. R. (2013). “Geomorphic classifications of rivers,” in Treatise on Geomorphology, Fluvial Geomorphology, Vol. 9, eds J. Shroder and E. Wohl (San Diego, CA: Academic Press), 730–767.
Chorley, R. J., and Kennedy, B. A. (1971). Physical Geography: A Systems Approach. London: Prentice Hall), 370.
Church, M. (2006). Bed material transport and the morphology of alluvial river channels. Annu. Rev. Earth Planet. Sci. 34, 325–354. doi: 10.1146/annurev.earth.33.092203.122721
Cowardin, L. M., Carter, V, Golet, F. C., and Laroe, E. T. (1979). Classification of Wetlands and Deepwater Habitats of the United States. Washington, DC: US Department of the Interior, US Fish and Wildlife Service.
Cowardin, L. M., and Golet, F. C. (1995). US fish and wildlife service 1979 wetland classification: a review. Vegetatio 118, 139–152. doi: 10.1007/BF00045196
Dollar, E. S. J., James, C. S., Rogers, K. H., and Thoms, M. C. (2007). A framework for interdisciplinary understanding of rivers as ecosystems. Geomorphology 89, 147–162. doi: 10.1016/j.geomorph.2006.07.022
Edwards, R. J., Ellery, W. N., and Dunlevey, J. (2016). The role of the in situ weathering of dolerite on the formation of a peatland: the origin and evolution of Dartmoor Vlei in the KwaZulu-Natal Midlands, South Africa. Catena 143, 232–243. doi: 10.1016/j.catena.2016.04.016
Ellery, S. (2019). The origin of bedrock depression wetlands in the southern Cape of South Africa: a changing perspective (MSc thesis). Grahamstown: Rhodes University, South Africa.
Ellery, W. N., Grenfell, M. C., Grenfell, S. E., Kotze, D. C., McCarthy, T., Tooth, S., et al. (2009). WET-Origins: Controls on the Distribution and Dynamics of Wetlands in South Africa. WRC Report TT 334/09. Pretoria: Water Research Commission.
Ellery, W. N., Grenfell, S. E., Grenfell, M. C., Humphries, M. S., Barnes, K., Dahlberg, A., et al. (2012). Peat formation in the context of the development of the Mkuze floodplain on the coastal plain of Maputaland, South Africa. Geomorphology 141, 11–20. doi: 10.1016/j.geomorph.2011.11.009
Ellery, W. N., Grenfell, S. E., Grenfell, M. C., Powell, R., Kotze, D. C., Marren, P. M., et al. (2016). “Wetlands in southern Africa,” in Quaternary Environmental Change in Southern Africa: Physical and Human dimensions, eds J. Knight and S. Grab (Cambridge: Cambridge University Press).
Ewart-Smith, J. L., Ollis, D. J., Day, J. A., and Malan, H. L. (2006). National Wetland Inventory: Development of a Wetland Classification System for South Africa. WRC Report No. KV 174/06. Pretoria: Water Research Commission.
Federal Geographic Data Committee (2013). Classification of Wetlands and Deepwater Habitats of the United States. FGDC-STD-004-2013, 2nd Edn. Washington, DC: Wetlands Subcommittee; Federal Geographic Data Committee; U.S. Fish and Wildlife Service.
Finlayson, C. M., and van der Valk, A. G. (1995). Wetland classification and inventory: a summary. Vegetatio 118, 185–192. doi: 10.1007/978-94-011-0427-2_15
Fryirs, K., and Brierley, G. (1998). The character and age structure of valley fills in upper Wolumla Creek catchment, south coast, New South Wales, Australia. Earth Surf. Proc. Land. 23, 271–287. doi: 10.1002/(SICI)1096-9837(199803)23:3<271::AID-ESP867>3.0.CO;2-5
Fryirs, K. A., Ralph, T. J., Larkin, Z. T., Tooth, S., Humphries, M., McCarthy, T., et al. (2018). A nested hierarchical perspective to enhance interpretations and communication in fluvial geomorphology for use in water resources management: Lessons from the Okavango Delta, Botswana. Geogr. J. 184, 192–207. doi: 10.1111/geoj.12250
Gell, P., Fluin, J., Tibby, J., Hancock, G., Harrison, J., Zawadzki, A., et al. (2009). Anthropogenic acceleration of sediment accretion in lowland floodplain wetlands, Murray–Darling Basin, Australia. Geomorphology 108, 122–126. doi: 10.1016/j.geomorph.2007.12.020
Gibling, M, R., Nanson, G, C., and Maroulis, J. C. (1998). Anastomosing river sedimentation in the Channel Country of central Australia. Sedimentology 45, 595–619. doi: 10.1046/j.1365-3091.1998.00163.x
Goudie, A. S., and Wells, G. L. (1995). The nature, distribution and formation of pans in arid zones. Earth Sci. Rev. 38, 1–69. doi: 10.1016/0012-8252(94)00066-6
Greear, P. F. (1967). Composition, diversity and structure of the vegetation of some natural ponds in Georgia (PhD dissertation). University of Georgia, Athens, GA, USA.
Grenfell, M. C., Ellery, W., and Grenfell, S. E. (2008). Tributary valley impoundment by trunk river floodplain development: a case study from the KwaZulu-Natal Drakensberg foothills, eastern South Africa. Earth Surf. Proc. Land. 33, 2029–2044. doi: 10.1002/esp.1652
Grenfell, M. C., Ellery, W. N., and Grenfell, S. E. (2009). Valley morphology and sediment cascades within a wetland system in the KwaZulu-Natal Drakensberg Foothills, Eastern South Africa. Catena 78, 20–35. doi: 10.1016/j.catena.2009.02.004
Grenfell, S. E., and Ellery, W. N. (2009). Hydrology, sediment transport dynamics and geomorphology of a variable flow river: the Mfolozi River, South Africa. Water SA 35, 271–282. doi: 10.4314/wsa.v35i3.76764
Grenfell, S. E., Ellery, W. N., and Grenfell, M. C. (2009). Geomorphology and dynamics of the Mfolozi River floodplain, KwaZulu-Natal, South Africa. Geomorphology 107, 226–240. doi: 10.1016/j.geomorph.2008.12.011
Grenfell, S. E., Ellery, W. N., Grenfell, M. C., Ramsay, L. F., and Flügel, T. J. (2010). Sedimentary facies and geomorphic evolution of a blocked-valley lake: Lake Futululu, northern Kwazulu-Natal, South Africa. Sedimentology 57, 1159–1174. doi: 10.1111/j.1365-3091.2009.01141.x
Grenfell, S. E., Grenfell, M. C., Rowntree, K. M., and Ellery, W. N. (2014). Fluvial connectivity and climate: a comparison of channel pattern and process in two climatically contrasting fluvial sedimentary systems in South Africa. Geomorphology 205, 142–154. doi: 10.1016/j.geomorph.2012.05.010
Grenfell, S. E., Rowntree, K. M., and Grenfell, M. C. (2012). Morphodynamics of a gully and floodout system in the Sneeuberg Mountains of the semi-arid Karoo, South Africa: implications for local landscape connectivity. Catena 89, 8–21. doi: 10.1016/j.catena.2011.09.007
Harvey, A. M. (2001). Coupling between hillslopes and channels in upland fluvial systems: implications for landscape sensitivity, illustrated from the Howgill Fells, northwest England. Catena 42, 225–250. doi: 10.1016/S0341-8162(00)00139-9
Holmes, P., and Meadows, M. (2012). Southern African Geomorphology: Recent Trends and New Directions. Bloemfontein: SUN MeDIA.
Jaeger, K. L., Montgomery, D. R., and Bolton, S. M. (2007). Channel and perennial flow initiation in headwater streams: management implications of variability in source-area size. Environ. Manag. 40, 775–786. doi: 10.1007/s00267-005-0311-2
Jerolmack, D. J., and Mohrig, D. (2007). Conditions for branching in depositional rivers. Geology 35, 463–466. doi: 10.1130/G23308A.1
Job, N. M., and Le Roux, P. A. L. (2018). Preliminary Guidelines to Apply Hydropedology in Support of Wetland Assessment and Reserve Determination. WRC Report No K5-2548. Pretoria: Water Research Commission.
Joubert, R., and Ellery, W. N. (2013). Controls on the formation of Wakkerstroom Vlei, Mpumalanga province, South Africa. Afr. J. Aquat. Sci. 38, 135–151. doi: 10.2989/16085914.2012.762897
Keen-Zebert, A., Tooth, S., Rodnight, H., Duller, G. A. T., Roberts, H. M., and Grenfell, M. (2013). Late quaternary floodplain reworking and the preservation of alluvial sedimentary archives in unconfined and confined river valleys in the eastern interior of South Africa. Geomorphology 185, 54–66. doi: 10.1016/j.geomorph.2012.12.004
Kleinhans, M. G. (2010). Sorting out river channel patterns. Prog. Phys. Geogr. 34, 287–326. doi: 10.1177/0309133310365300
Kleinhans, M. G., and Van den Berg, J. H. (2011). River channel and bar patterns explained and predicted by an empirical and a physics-based method. Earth Surf. Proc. Land. 36, 721–738. doi: 10.1002/esp.2090
Kobza, R. M., Trexler, J. C., Loftus, W. F., and Perry, S. A. (2004). Community structure of fishes inhabiting aquatic refuges in a threatened Karst wetland and its implications for ecosystem management. Biol. Conserv. 116, 153–165. doi: 10.1016/S0006-3207(03)00186-1
Kondolf, G. M., Boulton, A. J., O'daniel, S., Poole, G. C., Rahel, F. J., Stanley, E. H., et al. (2006). Process-based ecological river restoration: visualizing three-dimensional connectivity and dynamic vectors to recover lost linkages. Ecol. Soc. 11, 1–16. doi: 10.5751/ES-01747-110205
Kondolf, G. M., and Piégay, H. (2016). Tools in Fluvial Geomorphology, 2nd Edn. Chichester: John Wiley & Sons), 568.
Kotze, D., Marneweck, G., Batchelor, A., Lindley, D., and Collins, N. (2009). WET-Ecoservices: A Technique for Rapidly Assessing Ecosystem Services Supplied by wetlands. WRC Report TT 339/09. Pretoria: Water Research Commission.
Kotze, D. C., Highes, J. C., Breen, C. M., and Klug, J. R. (1994). The Development of a Wetland Classification System for KwaZulu-Natal, South Africa. WRC Report No. 501/4/94. Pretoria: Water Research Commission.
Kuenene, B. T., Van Huysteen, C. W., and Le Roux, P. A. L. (2013). Selected soil properties as indicators of soil water regime in the Cathedral Peak VI catchment of KwaZulu-Natal, South Africa. S. Afr. J. Plant Soil 30, 1–6. doi: 10.1080/02571862.2013.767387
Kuenene, B. T., Van Huysteen, C. W., Le Roux, P. A. L., Hensley, M., and Everson, C. S. (2011). Facilitating interpretation of Cathedral Peak VI catchment hydrograph using soil drainage curves. S. Afr. J. Geol. 114, 525–534. doi: 10.2113/gssajg.114.3-4.525
Larkin, Z. T., Ralph, T. J., Tooth, S., and McCarthy, T. S. (2017). The interplay between extrinsic and intrinsic controls in determining floodplain wetland characteristics in the South African drylands. Earth Surf. Proc. Land. 42, 1092–1109. doi: 10.1002/esp.4075
Lauer, J. W., and Parker, G. (2008). Net local removal of floodplain sediment by river meander migration. Geomorphology 96, 123–149. doi: 10.1016/j.geomorph.2007.08.003
Lidzhegu, Z. (2019). A classification of large wetlands in Africa's elevated drylands based on their formation, structure and hydrological functioning using Earth Observation and GIS (Unpublished PhD thesis), Grahamstown: Rhodes University, South Africa.
Lisenby, P. E., Tooth, S., and Ralph, T. J. (2019). Product vs. process? The role of geomorphology in wetland characterization. Sci. Total Environ. 663, 980–991. doi: 10.1016/j.scitotenv.2019.01.399
Macklin, M, G., and Lewin, J. (2003). River sediments, great floods and centennial-scale Holocene climate change. J. Quat. Sci. 18, 101–105. doi: 10.1002/jqs.751
Makaske, B., Smith, D. G., and Berendsen, H. J. (2002). Avulsions, channel evolution and floodplain sedimentation rates of the anastomosing upper Columbia River, British Columbia, Canada. Sedimentology 49, 1049–1071. doi: 10.1046/j.1365-3091.2002.00489.x
Marshall, T., and Harmse, J. T. (1992). A review of the origin and propagation of pans. S. Afr. Geogr. 19, 9–21.
McCarthy, T. S., Barry, M., Bloem, A., Ellery, W. N., Heister, H., Merry, C. L., et al. (1997). The gradient of the Okavango fan, Botswana, and its sedimentological and tectonic implications. J. Afr. Earth Sci. 24, 65–78.
McCarthy, T. S., Ellery, W. N., and Ellery, K. (1993). Vegetation induced subsurface precipitation of carbonate as an aggradational process in the permanent swamps of the Okavango (delta) fan, Botswana. Chem. Geol. 107, 111–131. doi: 10.1016/0009-2541(93)90105-R
McCarthy, T. S., Smith, N. D., Ellery, W. N., and Gumbricht, T. (2002). “The Okavango Delta – semi-arid alluvial fan sedimentation related to incipient rifting,” in Sedimentation in Continental Rifts, Vol. 73, eds R. W. Renaut and G. M. Ashley (Tulsa, OK: Society for Sedimentary Geology), 179–193. doi: 10.2110/pec.02.73.0179
McCarthy, T. S., Stanistreet, I. G., Cairncross, B., Ellery, W. N., Ellery, K., Oelofse, R., et al. (1988). Incremental aggradation on the Okavango Delta-fan, Botswana. Geomorphology 1, 267–278. doi: 10.1016/0169-555X(88)90017-7
McCarthy, T. S., Tooth, S., Jacobs, Z., Rowberry, M. D., Thompson, M., Brandt, D., et al. (2011). The origin and development of the Nyl River floodplain wetland, Limpopo Province, South Africa: trunk–tributary river interactions in a dryland setting. S. Afr. Geogr. J. 93, 172–190. doi: 10.1080/03736245.2011.619324
Montgomery, D. R., and Dietrich, W. E. (1988). Where do channels begin? Nature 336, 232–234. doi: 10.1038/336232a0
Nanson, G. C., and Croke, J. C. (1992). A genetic classification of floodplains. Geomorphology 4, 459–486. doi: 10.1016/0169-555X(92)90039-Q
Nanson, G. C., and Knighton, A. D. (1996). Anabranching rivers: their cause, character and classification. Earth Surf. Proc. Land. 21, 217–239. doi: 10.1002/(SICI)1096-9837(199603)21:3<217::AID-ESP611>3.0.CO;2-U
O'Driscoll, M. A., and Parizek, R. R. (2003). The hydrologic catchment area of a chain of karst wetlands in central Pennsylvania, USA. Wetlands 23, 171–179. doi: 10.1672/0277-5212(2003)023[0171:THCAOA]2.0.CO;2
Ollis, D. J., Ewart-Smith, J. L., Day, J. A., Job, N. M., Macfarlane, D. M., Snaddon, C. D., et al. (2015). The development of a classification system for inland aquatic ecosystems in South Africa. Water SA 41, 727–745. doi: 10.4314/wsa.v41i5.16
Parker, G. (1976). On the cause and characteristic scales of meandering and braiding in rivers. J. Fluid Mech. 76, 457–479.
Pulley, S., Ellery, W. N., Lagesse, J. V., Schlegel, P. K., and Mcnamara, S. J. (2018). Gully erosion as a mechanism for wetland formation: an examination of two contrasting landscapes. Land Degrad. Dev. 29, 1756–1767. doi: 10.1002/ldr.2972
Rebelo, A. J., Le Maitre, D. C., Esler, K. J., and Cowling, R. M. (2015). Hydrological responses of a valley-bottom wetland to land-use/land-cover change in a South African catchment: making a case for wetland restoration. Restor. Ecol. 23, 829–841. doi: 10.1111/rec.12251
Rountree, M. W., Heritage, G. L., and Rogers, K. H. (2001). In-Channel Metamorphosis in a Semiarid, Mixed Bedrock/Alluvial River System: Implications for Instream Flow Requirements (Birminghamn: IAHS Publishers), 266, 113–123.
Schulze, R. E. (1997). South African Atlas of Agrohydrology and Climatology. WRC Report TT82/96. Pretoria: Water Research Commission.
Schumm, S. A. (1979). Geomorphic thresholds: the concept and its applications. Trans. Inst. Br. Geogr. 4, 485–515. doi: 10.2307/622211
Schumm, S. A. (1994). Erroneous perceptions of fluvial hazards. Geomorphology 10, 129–138. doi: 10.1016/0169-555X(94)90012-4
Scott, D. A., and Jones, T. A. (1995). Classification and inventory of wetlands: a global overview. Vegetatio 118, 3–16. doi: 10.1007/978-94-011-0427-2_2
Semeniuk, C. A., and Semeniuk, V. (1995). A geomorphic approach to global classification for inland wetlands. Vegetatio 118, 103–124. doi: 10.1007/BF00045193
Sieben, E. J., Khubeka, S. P., Sithole, S., Job, N. M., and Kotze, D. C. (2018). The classification of wetlands: integration of top-down and bottom-up approaches and their significance for ecosystem service determination. Wetlands Ecol. Manage. 26, 441–458. doi: 10.1007/s11273-017-9585-4
Sieben, E. J. J., Ellery, W. N., Kotze, D. C., and Rountree, M. (2011). Hierarchical spatial organization and prioritization of wetlands: a conceptual model for wetland rehabilitation in South Africa. Wetlands Ecol. Manage. 19, 209–222. doi: 10.1007/s11273-011-9212-8
Simenstad, C., Reed, D., and Ford, M. (2006). When is restoration not?: Incorporating landscape-scale processes to restore self-sustaining ecosystems in coastal wetland restoration. Ecol. Eng. 26, 27–39. doi: 10.1016/j.ecoleng.2005.09.007
Smith, N. D., McCarthy, T. S., Ellery, W. N., Merry, C. L., and Rütther, H. (1997). Avulsion and anastomosis in the panhandle region of the Okavango Fan, Botswana. Geomorphology 20, 49–65. doi: 10.1016/S0169-555X(96)00051-7
Stanistreet, I. G., Cairncross, B., and McCarthy, T. S. (1993). “Low sinuosity and meandering bedload rivers of the Okavango Fan: channel confinement by vegetated levées without fine sediment,” in Current Research in Fluvial Sedimentology. Sedimentary Geology, Vol. 85, ed C. R. Fielding, 135–156. doi: 10.1016/0037-0738(93)90079-K
Tanner, J. L., Smith, C., Ellery, W. N., and Schlegel, P. (2018). Determining the Hydrological Functioning of the Palmiet Wetlands in the Eastern and Western Cape of South Africa. WRC Report No K5-2548. Pretoria: Water Research Commission.
Thoms, M. C. (2006). Variability in riverine ecosystems. River Res. Appl. 22, 115–121. doi: 10.1002/rra.900
Tooth, S. (2018). The geomorphology of wetlands in drylands: resilience, nonresilience, or…? Geomorphology 305, 33–48. doi: 10.1016/j.geomorph.2017.10.017
Tooth, S., Brandt, D., Hancox, P. J., and McCarthy, T. S. (2004). Geological controls on alluvial river behaviour: a comparative study of three rivers on the South African Highveld. J. Afr. Earth Sci. 38, 79–97. doi: 10.1016/j.jafrearsci.2003.08.003
Tooth, S., Ellery, W. N., Grenfell, M. C., Thomas, A., Kotze, D., and Ralph, T. (2015). 10 Reasons Why the Geomorphology of Wetlands Is Important. Wetlands in Drylands Research Network. Available online at: http://wetlandsindrylands.net/wp-content/uploads/2015/10/10-Reasons-Geomorphology-of-Wetlands-NEAR-FINAL-FULL-COLOUR.pdf (accessed January 23, 2019).
Tooth, S., McCarthy, T., Rodnight, H., Keen-Zebert, A., Rowberry, M., and Brandt, D. (2014). Late holocene development of a major fluvial discontinuity in floodplain wetlands of the Blood River, eastern South Africa. Geomorphology 205, 128–141. doi: 10.1016/j.geomorph.2011.12.045
Tooth, S., and McCarthy, T. S. (2004). Anabranching in mixed bedrock-alluvial rivers: the example of the Orange River above Augrabies Falls, Northern Cape Province, South Africa. Geomorphology 57, 235–262. doi: 10.1016/S0169-555X(03)00105-3
Tooth, S., and McCarthy, T. S. (2007). Wetlands in drylands: geomorphological and sedimentological characteristics, with emphasis on examples from southern Africa. Prog.Phys. Geogr. 31, 3–41. doi: 10.1177/0309133307073879
Tooth, S., McCarthy, T. S., Brandt, D., Hancox, P. J., and Morris, R. (2002). Geological controls on the formation of alluvial meanders and floodplain wetlands: the example of the Klip River, eastern Free State, South Africa. Earth Surf. Proc. Land. 27, 797–815. doi: 10.1002/esp.353
Tooth, S., Rodnight, H., Duller, G. A. T., McCarthy, T. S., Marren, P. M., and Brandt, D. (2007). Chronology and controls of avulsion along a mixed bedrock-alluvial river. Geol. Soc. Am. Bull. 119, 452–461. doi: 10.1130/B26032.1
UNEP (1997) United Nations Environment Programme World Atlas of Desertification 2nd Edn. London: UNEP.
Van Tol, J. J., Le Roux, P. A. L., Hensley, M., and Lorentz, S. A. (2011). Soil indicators of hillslope hydrology in Bedford catchment. S. Afr. J. Plant Soil 27, 242–251. doi: 10.1080/02571862.2010.10639993
Wohl, E., Bledsoe, B. P., Jacobson, R. B., Poff, N. L., Rathburn, S. L., Walters, D. M., et al. (2015). The natural sediment regime in rivers: broadening the foundation for ecosystem management. Bioscience 65, 358–371. doi: 10.1093/biosci/biv002
Keywords: wetland geomorphology, wetland classification, wetland restoration, wetland management, drylands
Citation: Grenfell S, Grenfell M, Ellery W, Job N and Walters D (2019) A Genetic Geomorphic Classification System for Southern African Palustrine Wetlands: Global Implications for the Management of Wetlands in Drylands. Front. Environ. Sci. 7:174. doi: 10.3389/fenvs.2019.00174
Received: 08 May 2019; Accepted: 16 October 2019;
Published: 05 November 2019.
Edited by:
Anne Alje Van Dam, IHE Delft Institute for Water Education, NetherlandsReviewed by:
Stefanos Xenarios, Nazarbayev University, KazakhstanGordon Randy Milton, Nova Scotia Department of Natural Resources, Canada
Copyright © 2019 Grenfell, Grenfell, Ellery, Job and Walters. This is an open-access article distributed under the terms of the Creative Commons Attribution License (CC BY). The use, distribution or reproduction in other forums is permitted, provided the original author(s) and the copyright owner(s) are credited and that the original publication in this journal is cited, in accordance with accepted academic practice. No use, distribution or reproduction is permitted which does not comply with these terms.
*Correspondence: Suzanne Grenfell, c2dyZW5mZWxsQHN1bi5hYy56YQ==