- 1Centre de Recherches de Climatologie, UMR 6282 CNRS/UB Biogéosciences, Université Bourgogne-Franche-Comté, Dijon, France
- 2Comité Champagne CIVC, Épernay, France
- 3Department of Viticulture and Enology, California State University, Fresno, Fresno, CA, United States
- 4SEDS, UMR 6282 CNRS/UB Biogéosciences, Université Bourgogne-Franche-Comté, Dijon, France
- 5EGFV, Bordeaux Sciences Agro, INRAE, Univ. Bordeaux, ISVV, Villenave d’Ornon, France
In rainfed agriculture systems, rainfall water management (harvesting, storage, and efficient use) is a key issue. At local scale (i.e., from 100 m to 50 km), the impact of rainfall spatial and temporal variability on crop water availability is seldom addressed. In order to accurately depict the space and time variations of rainfall at local scale, a dense rain-gauges network composed of 45 rain-gauges has been deployed over 28-km2 area, in Burgundy vineyards (North-East France). Rainfall data collected by each rain gauge from 2014 to 2016 were used as input variables in the Lebon et al. (2003) grapevine water balance model. All other climate variables, vineyard, and soil parameters were kept the same for each simulation in order to capture the impact of the sole spatial variability of rainfall on vineyard water status. As rainfall dynamics impact on the vineyard depends on the soil water content, water balance was modeled considering soils with low (50 mm) and medium (150 mm) soil water-holding capacities, representative of the soils of the area. The impact of modeled soil water availability for grapevine was assessed using the water deficit stress index (WDSI), i.e., the relative stomatal conductance. Local rainfall variability throughout the vine vegetative period leads to large variations in WDSI; it varied up to 0.3 within the study area due to because of rainfall spatial variability. Using a set of 34 weather stations at mesoscale level over Burgundy (186 km from North to South), we showed that local rainfall might contribute to change in grapevine water status as large as 50% of the simulated regional water balance spatial variability. Our results indicate that local rainfall and its impacts on agricultural production are probably not sufficiently considered in farming systems, potentially leading to inaccurate water management (cover-crop, irrigation) due to sparse rainfall network.
Introduction
Water management throughout the 21st century is a widely documented and certainly a challenging matter (see for example Clothier et al., 2010). Growing population, changes in food quality, and development of non-feeding agricultural products (such as bioenergy) will undoubtedly lead to increased pressure on natural resources, including water. Crop water consumption is expected to grow from 30 to 53% in 2050, in comparison the early 21st century (de Fraiture and Wichelns, 2010). Therefore, large improvements in both rainfed and irrigated agriculture are required to limit water demand and to provide sufficient food and limit the impacts of crop production on the environment. This can be achieved through adapted and improved agronomical practices as well as regulations imposed by regional and global policies (Howell, 2001; Ward and Michelsen, 2002; Fedoroff et al., 2010).
At the field scale, water can be saved by the use of high water-use efficiency plant material (Condon et al., 2004; Marguerit et al., 2012), soil management techniques (Hatfield et al., 2001), or accurate irrigation systems (Howell, 2001) and planning (Wang et al., 2001; Ali and Talukder, 2008).
For the specific case of rainfed agriculture, water management strongly relies on rainfall water harvesting, water storage, reducing non-productive evaporation, increasing plant water uptake capacity (e.g., with optimum crop geometry, conservation agriculture…), and increasing the water use efficiency of crops (through adapted plant species and varieties) (Rockström et al., 2010; Rossato et al., 2017).
To develop accurate strategies and policies to collect and save rainfall water, fine knowledge of space and time rainfall patterns is necessary. However, even at local scale [i.e., from 100 m to 50 km, according to Oke (1987)], the spatial variability of rainfall can lead to large variations in the spatial distribution of water resources (Finnerty et al., 1997), specifically during convective events (Duncan et al., 1993). Numerous studies have documented the substantial variability of rainfall at local scale (e.g., Berne et al., 2004; Ciach and Krajewski, 2006; Villarini et al., 2008) and its impact on potential rainfall erosivity (Fiener and Auerswald, 2009). Accounting for rainfall spatial variability has been previously suggested as it can be suspected to affect experimental trials results for agriculture (Sivakumar and Hatfield, 1990). We address in this paper the potential impact of rainfall local spatial variability on agriculture, using grapevine as a reference cropping system.
Grapes is a crop for which water management produced a significant body of scientific literature. Grapevine requires limited input of water. When it suffers a moderate water deficit during the fruit development, it produces grapes with high-quality potential for winemaking (Seguin, 1986; van Leeuwen et al., 2009; Acevedo-Opazo et al., 2010). Water status is often considered as a key factor of the so-called “Terroir effect,” a concept that bounds the sensory characteristics of a product to its area of production, because of various factors among which soil and climate conditions (van Leeuwen, 2010). As for other crops, severe water deficit reduces yield (Hardie and Considine, 1976). The environmental and agronomical factors affecting grapevine water status are largely documented (Deloire et al., 2004; Vaudour et al., 2015). At local scale, soil water status variability is addressed mainly through soil, topography, and plant-based studies (André et al., 2012; Bellvert et al., 2013; Bonfante et al., 2015; Brillante et al., 2016a,b). The role of climate variation at local scale on grapevine water status has recently been considered through terrain impact on radiative balance (and therefore vineyard evapotranspiration) and rainfall runoff using water balance modeling (Hofmann et al., 2014).
Local scale weather variability has been increasingly studied lately in vineyards (Quénol, 2014), although mostly focusing on air temperature and its impact on grapevine precocity. Considerable variations in temperature have been recently reported (Quénol and Bonnardot, 2014), up to 300°days (Winkler index) within less than 2-km distance (Bonnefoy et al., 2013). Yet, impact of rainfall spatial variability at this scale on viticulture has not been addressed so far.
The current article explores the potential impact of local rainfall space and time variability on grapevine water availability through the response of a grapevine soil–water balance model to local rainfall variability using a high-density rain-gauges network installed in vineyards located in Burgundy (North-East France). Our research aims at understanding whether or not rainfall local variations play a significant role in grape production in quantity and quality.
Materials and Methods
Study Area and Rain Gauge Networks
The study was conducted over a 28-km2 area located in Burgundy winegrowing region, France (Figure 1). Climate is oceanic with continental and Mediterranean influences (Chabin, 2004). These influences are represented by moderately cold winter and warm summers, with approximately 760 mm of annual precipitation (Dijon data, 1981–2010 normals1). Precipitation is evenly distributed during the year (from 43 to 86 mm each month). During summer, most of the rainfall is brought by thunderstorms, which in hillslope conditions cause a reduced soil water supply compared to recorded rain amount because of their high intensity and the runoff they induce. The terrain of the study area is hilly, due to erosion of a southeast exposed hillside facing a large Plain (Saone Plain) during the Quaternary period. The elevation ranges from 200 to 450 m.
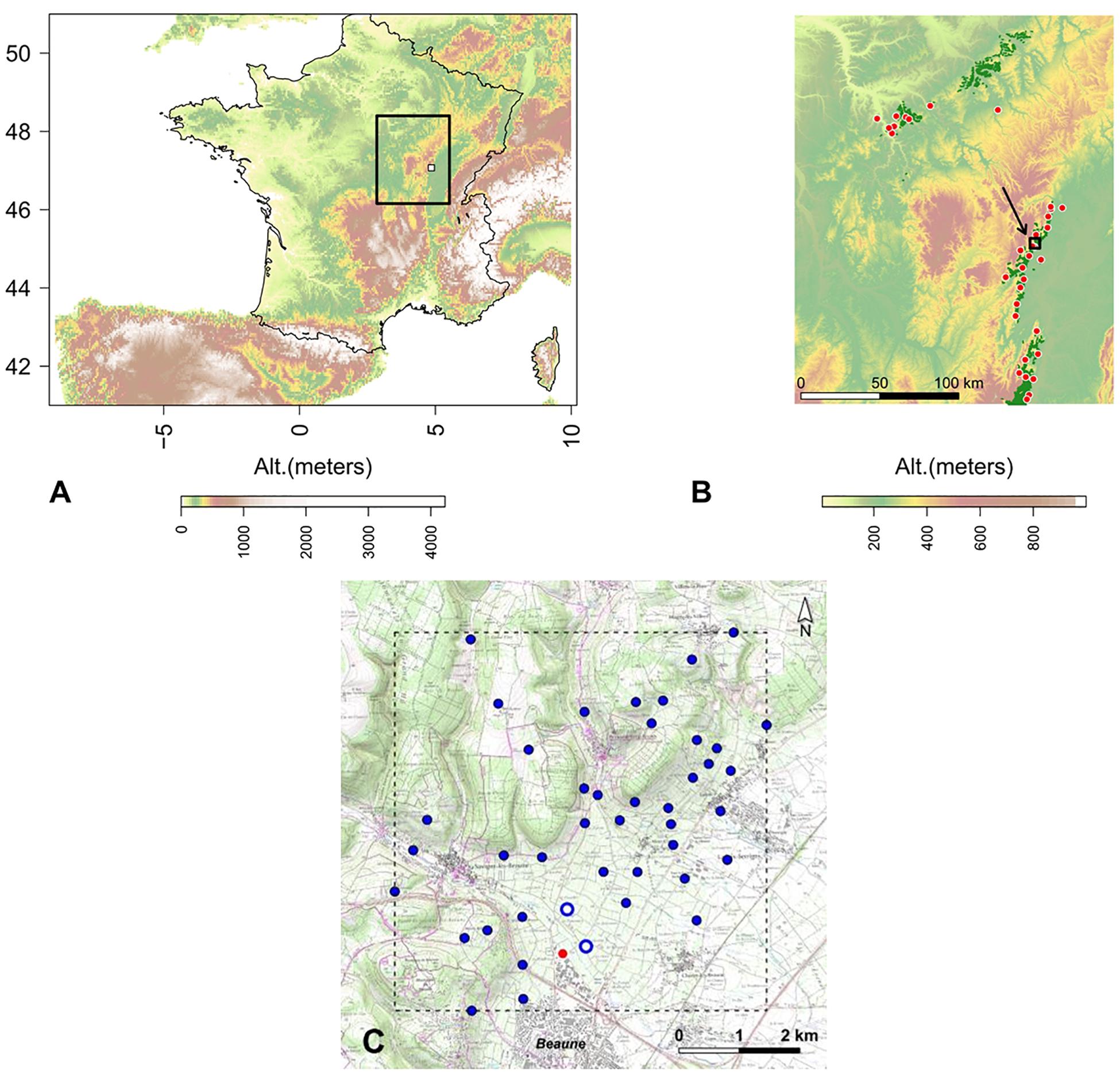
Figure 1. The study area. (A) Burgundy wine region location with coordinates in decimal degrees (black box, zoomed in plot B); (B) Weather stations locations (red dots). Dark-green areas correspond to vineyards. The Hydravitis network location is indicated by a black square pointed by the arrow; (C) the Hydravitis high-resolution rain gauges (blue dots) network. White-filled blue dots correspond to location where two rain gauges were installed aside. The red dot shows the location of the Beaune weather station (Climeo mesoscale network).
In order to capture space and time variability of rainfall, a very dense rain-gauge network composed of 45 tipping-bucket rain gauges (devices called “Rainnew 111,” by Rainwise Inc., Trenton, ME, United States) was installed from 2012 (first tests) to 2014 (final network size). These rain gauges are linked to a Hobo Pendant UA-002-64 event-temperature logger (Onset Computer Corp., Bourn, MA, United States) that records the time of occurrence of bucket tips. The rain gauges, located nearby vineyards, were implemented following the WMO recommendations (World Meteorological Organization, 2008), at a maximum angle of 30° between the top of the gauge to the top of the highest nearest obstacle. In order to assess measurement uncertainty, a pair of two gauges has been installed within a distance of 3 m between each gauge (white filled blue circles on Figure 1C). The resolution (0.258 mm/tip) and the average measurement error (ranging from 0.6 to 4.2%) of the rain gauges have been tested during a preliminary study. Network implementation and control are detailed in Pauthier et al. (2014).
From 2014 to 2016, during the vegetative periods of the vine, rain gauges were controlled every week in order to limit the potential clogging of the rain gauges.
Despite this very frequent maintenance, clogging, battery, or malfunctioning problems appeared on a few rain gauges. Erroneous or missing data were replaced by spatial interpolation using ordinary kriging.
Mesoscale climate variability was assessed using 2014–2016 data from 34 stations of the Climeo weather network (Figure 1B). Climeo is a weather stations network maintained by the union of Burgundy winegrowers and wine merchants (BIVB). It is complemented with stations from the French National Weather service (Meteo–France). All Climeo stations monitor rainfall, air temperature, and humidity 2 m above the ground. Reference evapotranspiration (ET0) was calculated using the Penman–Montheith FAO-56 formula (Allen et al., 1998). Where solar radiation was not available (27 weather stations), it was estimated using the Hargreaves radiation method (Hargreaves and Samani, 1982). Reference evapotranspiration was estimated by means of the Hargreaves temperature formula (Hargreaves and Samani, 1985). It was calibrated to match the Burgundy conditions using Penman–Monteith ET0 as a reference at the seven stations where it was calculated. Hagreaves ET0 was estimated with a root mean squared difference of 0.55 mm in comparison to Penman–Monteith estimates.
Water Balance Modeling
The Lebon model (Lebon et al., 2003) is based on the geometrical canopy model proposed by Riou et al. (1989) for vertical shoot positioned trellises, coupled to a soil–water balance routine accounting separately for grapevine transpiration and bare soil evaporation. This water balance model requires reference evapotranspiration (ET0) and rainfall as water inputs, daily solar radiation for solar radiation interception modeling, and daily air temperature for canopy development modeling (crop coefficient) based on degree days. The canopy expands from budburst to 10 days after flowering (estimated to be the date at which the canopy growth is limited due to vegetation mechanical trimming). The vineyard geometry was set for north to south aligned rows (vertical-shoot-position training), with an interrow distance of 1 m, a maximum canopy height of 0.7 m, a maximum canopy width of 0.35 m, and a minimum canopy porosity (proportion of gaps through the canopy) of 0.25. These parameters were set to match usual canopy geometry in the study area.
Temperature and relative humidity data were collected from a weather station located at Beaune (red dot on Figure 1C). Solar radiation and wind speed data were taken from a weather station located at Volnay (7.5 km south-eastward of the study area, as these variables were not recorded at Beaune station). ET0 was calculated using the Penman–Monteith FAO-56 model (Allen et al., 1998).
For all years from 2014 to 2016, 45 runs of Lebon model were performed, with the same parameters and input climate variables except rainfall. For each run, rainfall data collected from a different rain gauge were used. These 45 runs were performed twice, once for soils with water-holding capacity (WHC) set to 50 mm and once for 150 mm.
For each run, the simulated relative grapevine stomatal conductance, derived from the daily fraction of transpirable soil water (FTSW), was used to evaluate the changes in grapevine water status. The simulate grapevine stomatal conductance ranges from 0 (stomata closed, i.e., no transpiration and extreme water deficit stress) to 1 (stomata fully opened, i.e., maximum transpiration and no water deficit stress). Rather than using the bilinear relationship between FTSW and relative grapevine stomatal conductance, we used the inverse exponential equation proposed by Pieri and Gaudillere (2005). This variable is hereafter referred to as water deficit stress index, using WDSI as an acronym. Water deficit stress index was averaged on three major periods of grapevine and grape development period: from budburst (Bud) to flowering (Flo, i.e., blooming), when grapevine primary shoots and leaves actively develop; from flowering to veraison (Ver), when grape develops; and from veraison (Ver) to harvest (Har), when vegetative growth is very low and the grapes ripen.
The phenological stages have been retrieved from weekly observations of a commercial vineyard of Vitis vinifera cv. Pinot N from a domain located at Aloxe Corton (in the middle of the study area).
It should be specified that Lebon et al. (2003) model has been developed for flat terrain. It assumes 100% infiltration of precipitation. Although an adaptation to slope conditions has been proposed recently by Hofmann et al. (2014), the Lebon model was preferred because the aim of this study is limited to evaluate the sensitivity of vineyard water balance to the sole local rainfall space and time variability only, for two contrasted soil WHC.
The Lebon model was assessed in the study area by means of grapevine water status and soil water status monitoring to evaluate its relevance to simulate vineyard water balance using year 2013 data from a preliminary research (results not shown).
The results of the first experiment (270 water balance simulations using rainfall collected from 45 rain gauges, for 3 years and 2-soil WHC) were compared to those of a second experiment simulating vineyard water balance spatial variability at mesoscale level using Climeo weather data.
In this second experiment, we followed the same scheme as in the first, whereas all climate parameters (and not only rainfall) from each weather station were used as inputs in the water balance model. Indeed, at mesoscale, using all parameters alike but rainfall would have led to unrealistic weather variables combinations for some days (such as rainfall on a sunny day). As for the (local scale) first experiment, simulations were run for two different soil water capacities (i.e., 50 and 150 mm). This led to 204 water balance simulations (34 weather stations × 3 years × 2 soil WHC). Simulated WDSI was also averaged on three periods of the grapevine vegetative cycle mentioned above: Bud to Flo, Flo to Ver, and Ver to Har.
To avoid confusion throughout the text between time and space variability, the equations and acronyms of different metrics used in this article are defined below. They are statistics calculated for a collection of m (total) locations. At each location j, a rain gauge or weather station is located. The variable X is either rainfall (mm) (acronym = R) or WDSI.
The daily (spatial) range of X for a day i:
The daily (spatial) standard deviation of X, for a given day i:
The daily (spatial) standard deviation of X, for a given period (set of days):
Hereafter, a period is a set of days from a phenological stage to another. For example, σFloVer is the standard deviation calculated with the Xj values collected at each j location during the flowering-to-veraison (included) period.
Xperiod, j can be either the sum of each xi precipitation record at location (rain gauge or station) j at day i for a collection of n days corresponding to the period duration, that is:
or the average of each daily Xi,i calculated WDSI for location (rain gauge or weather station) j, on a collection of n days corresponding to the period duration, that is:
The period acronym can either be BudFlo, the budburst to flowering period; FloVer, the flowering to veraison period; or VerHar, the veraison to harvest period.
The δi, σi, and σperiod are metrics of the spatial variability of rainfall or WDSI at local or at mesoscale.
Results
Local Variability of Rainfall
The 3 years exhibited different profiles in climate conditions during the vegetative season (Table 1).
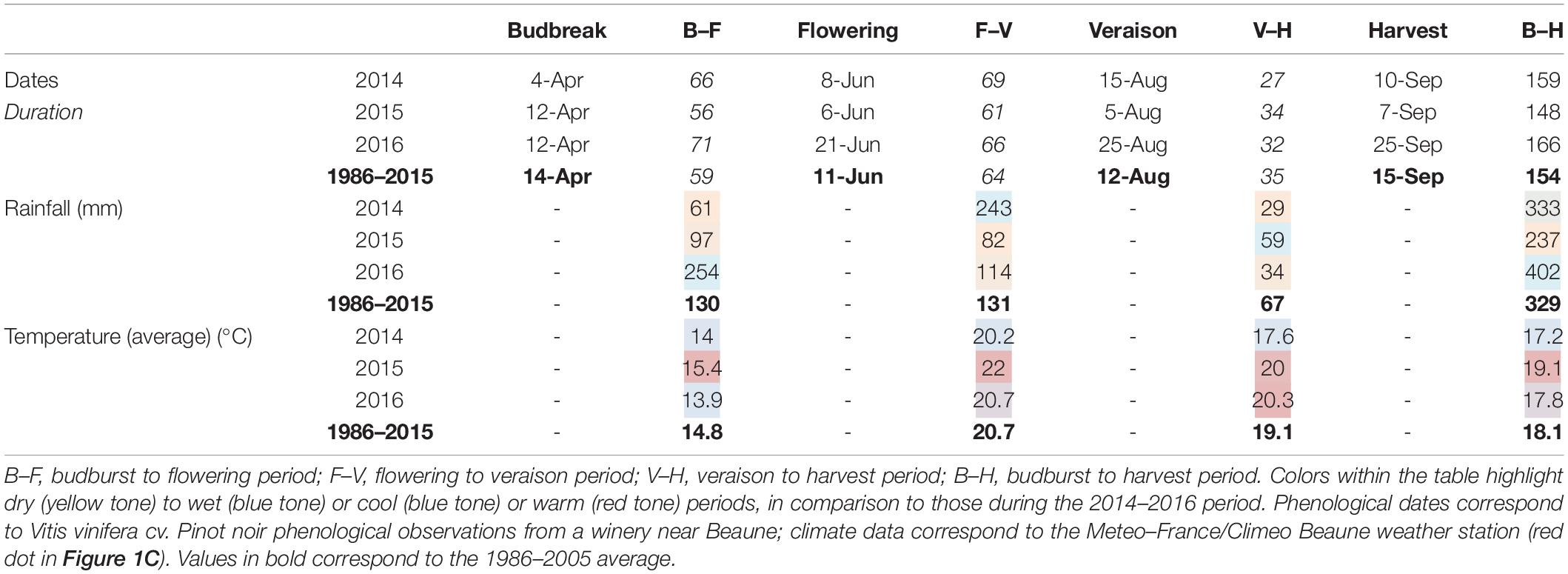
Table 1. Phenological stages and phases (period during two stages) dates, durations, number of days, and corresponding cumulated rainfall and average temperature.
2014 was cooler than average during the vegetative cycle of grapevine, with a wet summer (Table 1 and Figure 2). As the late winter of 2014 was quite warm, budburst occurred rather early (April 4), which, together with a warm period in the first two decades of June, lead to a harvest date on September 10, near the average of the normals (1986–2015; see Table 1). 2015, in contrast, was much warmer and dryer than average, with a little rainfall until harvest but two storms events in mid-June. All phenological stages in 2015 were early, in comparison to 2014 and 2016, from 2 (flowering) to 20 (veraison) days. In 2016, spring rainfall was high. Because of a cool and wet spring, flowering date (June 21) occurred 2 weeks later than in 2014 and 2015. Veraison was also considerably delayed (10 days later than in 2014 and 20 days later than in 2015). A warm spell from late August to early September 2016 allowed to reach maturity on September 25.
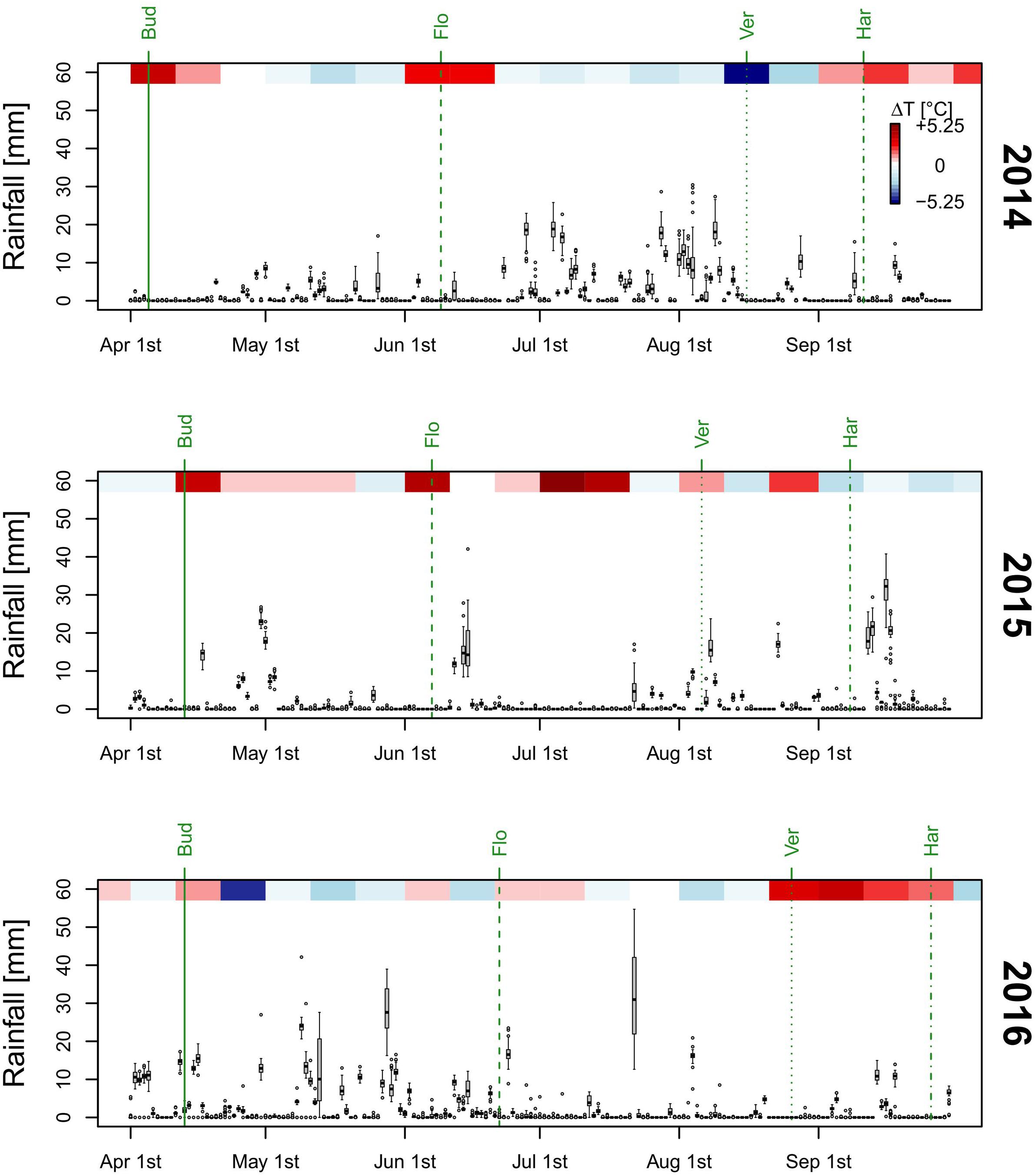
Figure 2. Daily rainfall variability. Each box plot shows the distribution of daily rainfall collected by 45 rain gauges. The colored bar on the top of each plot indicates the anomaly in average temperature [ΔT (°C)] per decade (i.e., 10 days) in comparison to the 1986–2015 decade average, from Beaune weather station (red dot on Figure 1C).
Spatial variability of rainfall at daily time step was largest during heavy daily rainfall events (i.e., showers and storms), with a maximum range on May 9, 2016 (42.1 mm), and on July 22, 2016 (42 mm). Not surprisingly, local variability of rainfall is larger on heavy precipitation events (Figure 3A). For a single day, local daily rainfall can range up to 42 mm.
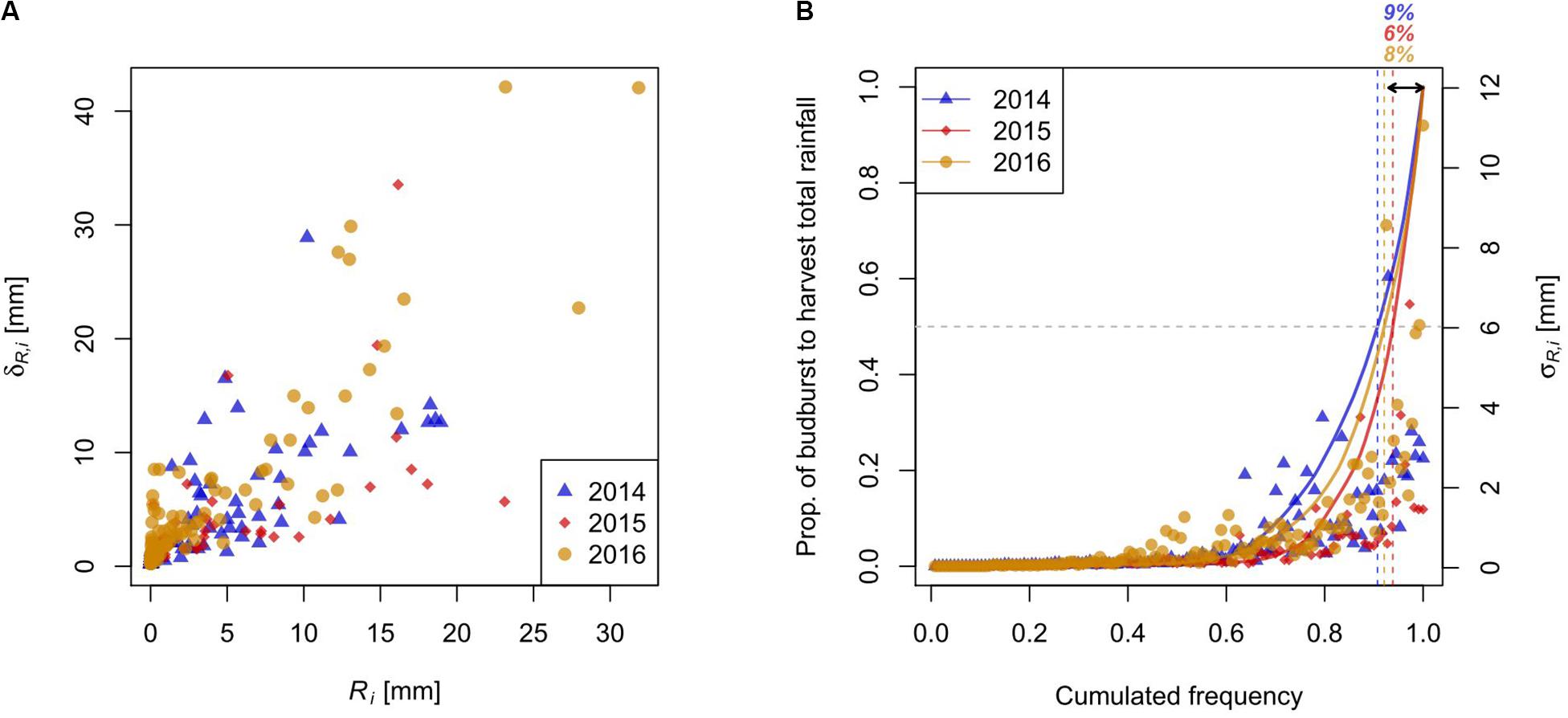
Figure 3. Local rainfall variability at daily time step during the grapevine vegetative period. (A) Spatial precipitation range (δR,i, i.e., max.–min. rainfall collected by the rain gauges on a given day) as function of daily precipitation height (Ri, mm) averages the Hydravitis local scale network. (B) The lines show the proportion of total cumulated rainfall from budburst to harvest as function of the cumulated distribution of each rainy (>0 mm) event (i.e., day), and the dots show the daily standard deviation of rainfall (σR,j, i.e., daily spatial variability). The colored number on the top of the plot indicates the percentage of rainy days (range underlined by arrows) during the budburst to harvest period that bring 50% of the total cumulated rainfall for this period (area delineated by colored dashed vertical lines).
In Figure 3B, lines are mapped to the x axis and the left y axis, whereas dots (in different shapes according to the year) are mapped to x axis and right y axis. Lines in Figure 3B show that 50% of the total cumulated rainfall from budburst to harvest (gray dashed horizontal line) is provided by 6% (in 2015) to 9% (in 2014) of the rainy days (colored number on the top of Figure 3B). Dots in Figure 3B show that during these days, the spatial variability is large: standard deviation between all 45 rain gauges is always larger than 1 mm; it is frequently greater than 2 mm and can reach up to 11 mm (in 2016). The fact that a few heavy rainy days, characterized by large local spatial variation, control most of the water input during the grapevine growing season might induce substantial variation in soil–water balance at local scale level.
Water Balance Variability at Local Scale
Figure 4 shows water balance modeling across the rain-gauge locations for all years, under the assumption of 100% precipitation infiltration. In 2014 and 2015, early water deficit was observed, with large spatial differences in WDSI profiles on soils with low WHC (50 mm) in 2014. The frequent rainfall events during summer 2014 reduced water deficit in both low and high WHC soils, where moderate to no water deficit was simulated at harvest. During 2015, after a series of 3 rainy days on June 12 (average = 11.7 mm), 14 (14.8 mm), and 15 (16.16 mm), the absence of rainfall until late July induced moderate to severe water deficit during most of the flowering to veraison period. In August, a series of rainy events brought heterogeneous rainfall in space, maintaining very high stress at a few locations, whereas most of the local area was sufficiently fed to reduce water deficit. Water deficit stress index at harvest was below 0.3 (i.e., moderate to severe) at almost all rain-gauges locations.
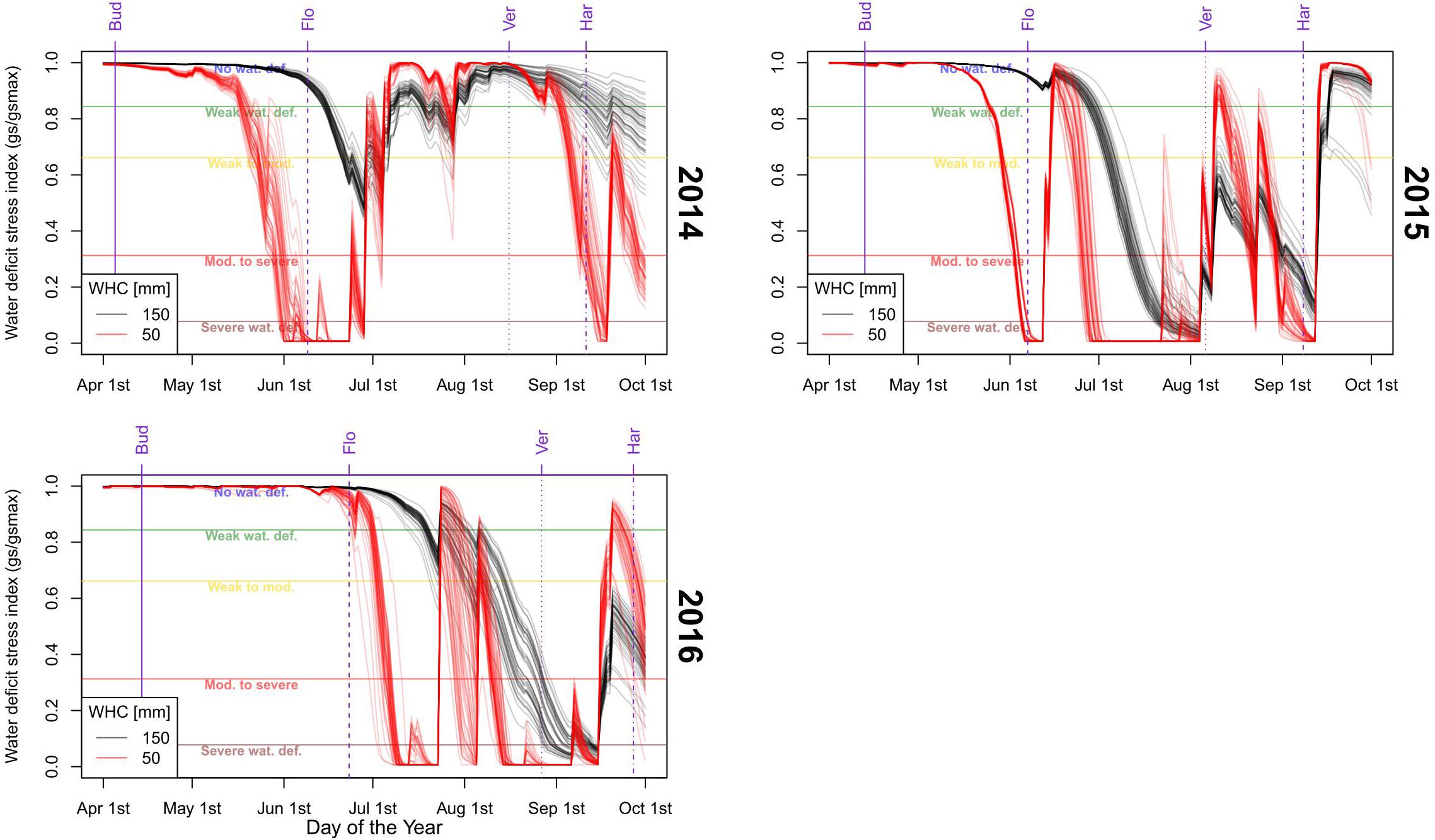
Figure 4. Water deficit stress index daily values for low (50 mm) and high (150 mm) WHC soils. Each line corresponds to a simulation using rainfall value collected at one of the 45 rain gauges of Hydravitis local scale network. Threshold values between each class have been calculated from corresponding predawn leaf water potential values in table 1 of van Leeuwen et al. (2009), using the exponential relationship between fraction of transpirable soil water and predawn leaf water potentiel (Figure 3 in Lebon et al., 2003). The colored horizontal lines indicates the upper limit of water deficit classes as proposed by van Leeuwen et al. (2009).
In 2016, water deficit installed quickly after flowering for 50 mm WHC, whereas it dropped gradually until mid-September to reach severe water deficit in mid-September on soils with moderate water capacity (i.e., WHC = 150 mm). A wet spell from September 15 to 19 reduced simulated water stress until harvest on September 25.
The spatial structure in grapevine water deficit stress is not maintained in time within the same year. That is, during a given year, areas with the lowest WDSI can change. In 2015, the highest simulated water deficit caused by rainfall spatial variability was located in the northern hilly part of the study area during the flowering to veraison period (Figure 5, top-right corner), whereas it was located on the southeastern part of the study area during the veraison-to-harvest period (simulations for a 50-mm WHC soil). In 2016, the location where the lowest flowering-to-veraison average WDSI (0.17) was calculated is at the northeast part of the study area, whereas it is found in the southwestern part of the study area for veraison to harvest (lowest WDSI = 0.19).
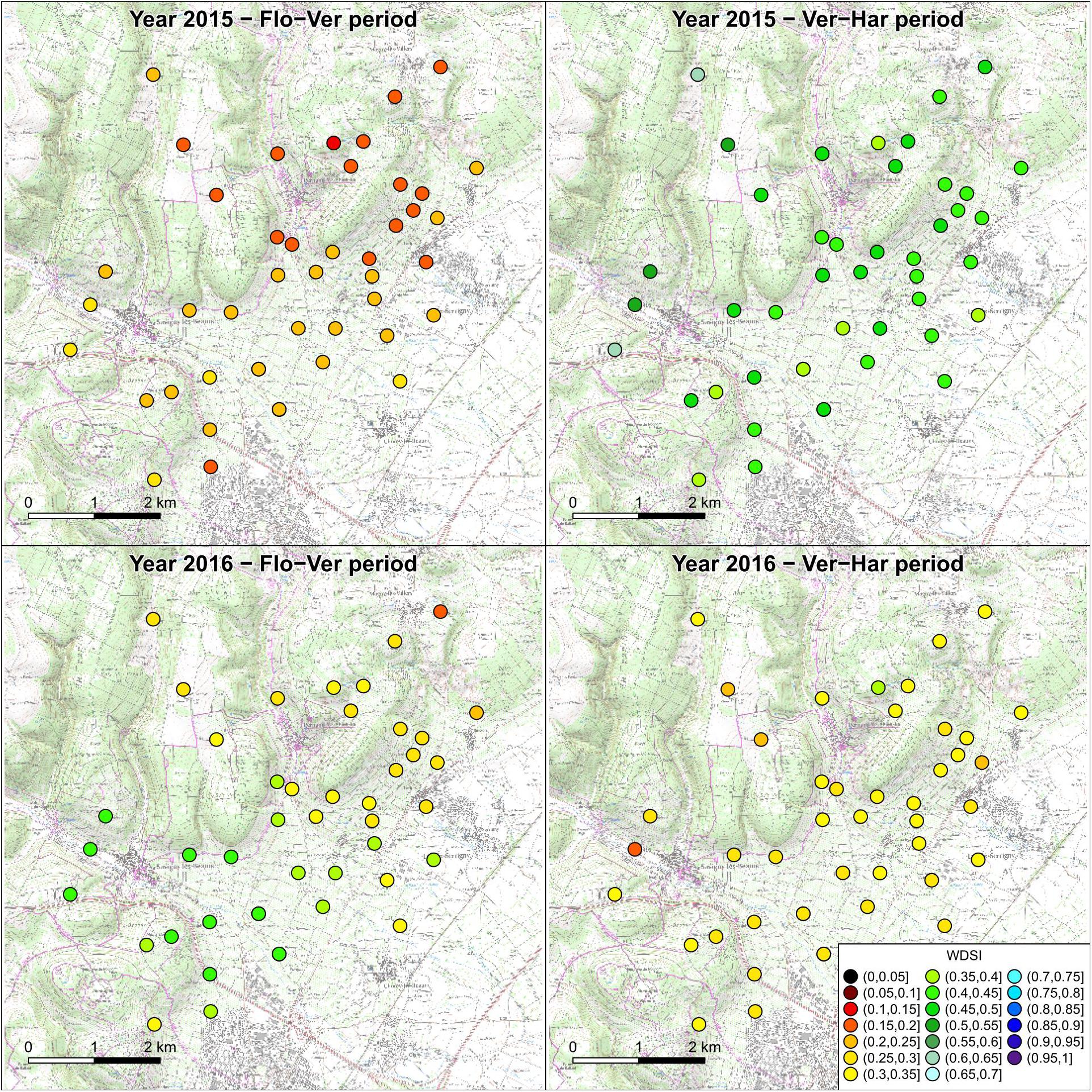
Figure 5. Maps of the average WDSI calculated using a 50-mm WHC soil during two grapevine development stages (Flo–Ver, flowering to veraison; Ver–Har, veraison to harvest) in 2015 and 2016. Each point corresponds to a rain gauge of the Hydravitis local scale network.
Local to Mesoscale Water Balance Variability
Water deficit stress index spatial variability at local scale (induced by changes in rainfall spatial distribution) was compared to climate-induced WDSI spatial variability at meso-scale, i.e., the Burgundy wine–producing region, using Climeo weather stations network. At local scale, the Hydravitis network captures rainfall variability over 6.3 (from north to south) and 6.1 (from west to east) km. In contrast, the Climeo network captures climate variable from 186 (from north to south) to 116 (west to east) km.
Figure 6 presents the distributions of WDSI averaged over three grapevine development periods: from budburst to flowering, from flowering to veraison, and from veraison to harvest. From budburst to floraison, WDSI remained high, so that little spatial variability was observed, either at local or at mesoscale, as soil water content remained close to the soil WHC, thus maintaining WDSI close to its maximum value. In 2014, however, weak water deficit was simulated at both local and mesoscale level for soil with low WHC (i.e., 50 mm). From flowering to veraison, water deficit was larger on low WHC soils, which was not the case during veraison to harvest, when scarce rainy events refill most of the soil WHC in low WHC soils.
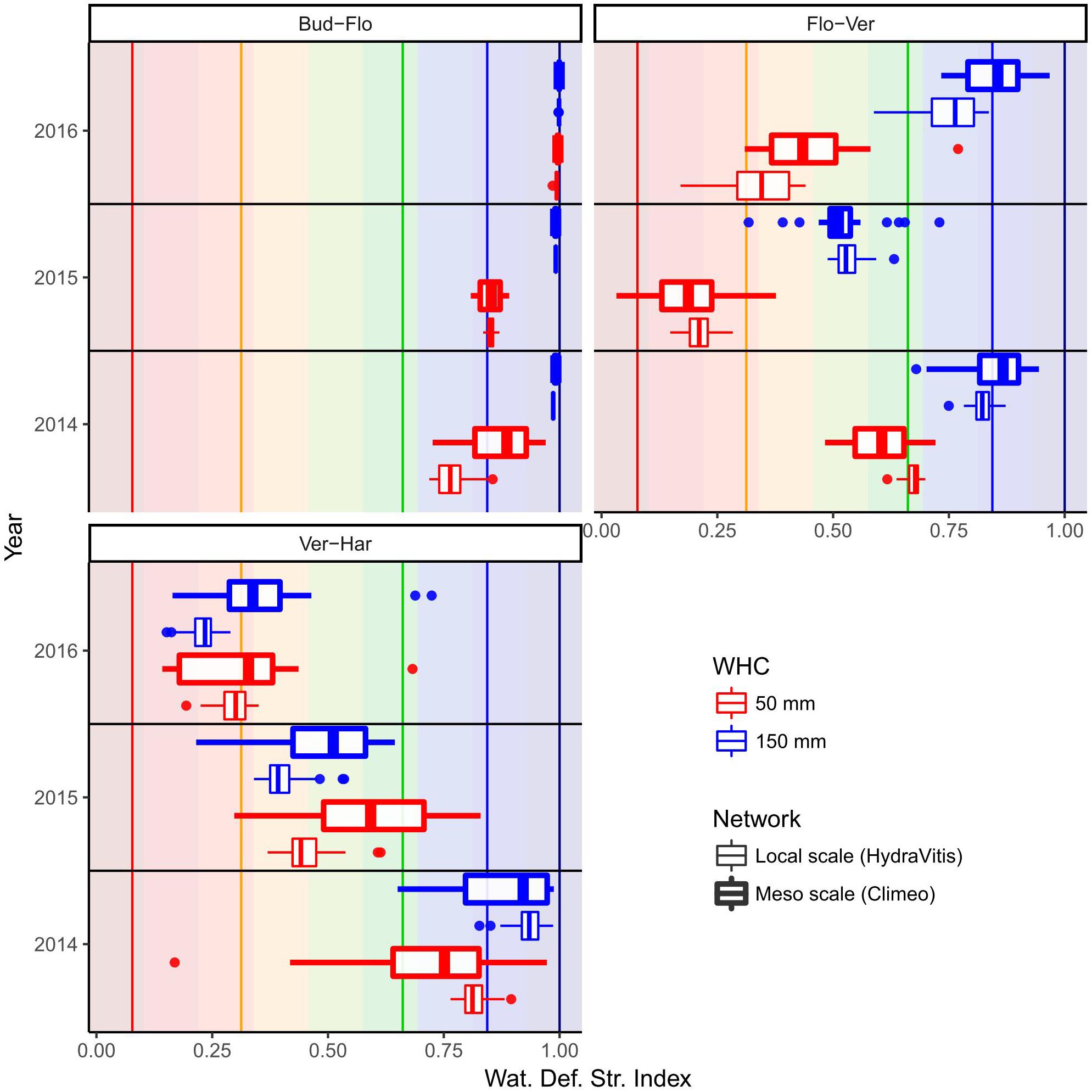
Figure 6. Comparison of local to mesoscale variability of simulated WDSI daily averages during three development stages (Bud–Flo, budburst to flowering; Flo–Ver, flowering to veraison; Ver–Har, veraison to harvest), for soils with low (50 mm) and high (150 mm) soil water contents (WHC). Note that local variability of WSDI resulted from simulations using different rainfall data at each location, whereas all other climate-related input (relative humidity, air temperature, wind speed, and solar radiation) came from the same location. For mesoscale variability, simulations were made using different climate related input for each location.
Moderate to severe water deficits were observed during the flowering-to-veraison period in 2015 and from veraison to harvest in 2016.
At mesoscale level, larger spatial variability was observed during the veraison-to-harvest period than during other periods, in all 3 years studied. Spatial standard deviation (σWDSI, period) ranged from 0.1034 (year 2015, soil WHC = 150 mm) to 0.1835 (year 2014, soil WHC = 50 mm, Table 2). In 2014, climate-based water deficit simulations suggested that grapevine water stress ranged from severe to moderate water deficit up to no water deficit, following the classification proposed by van Leeuwen et al. (2009).
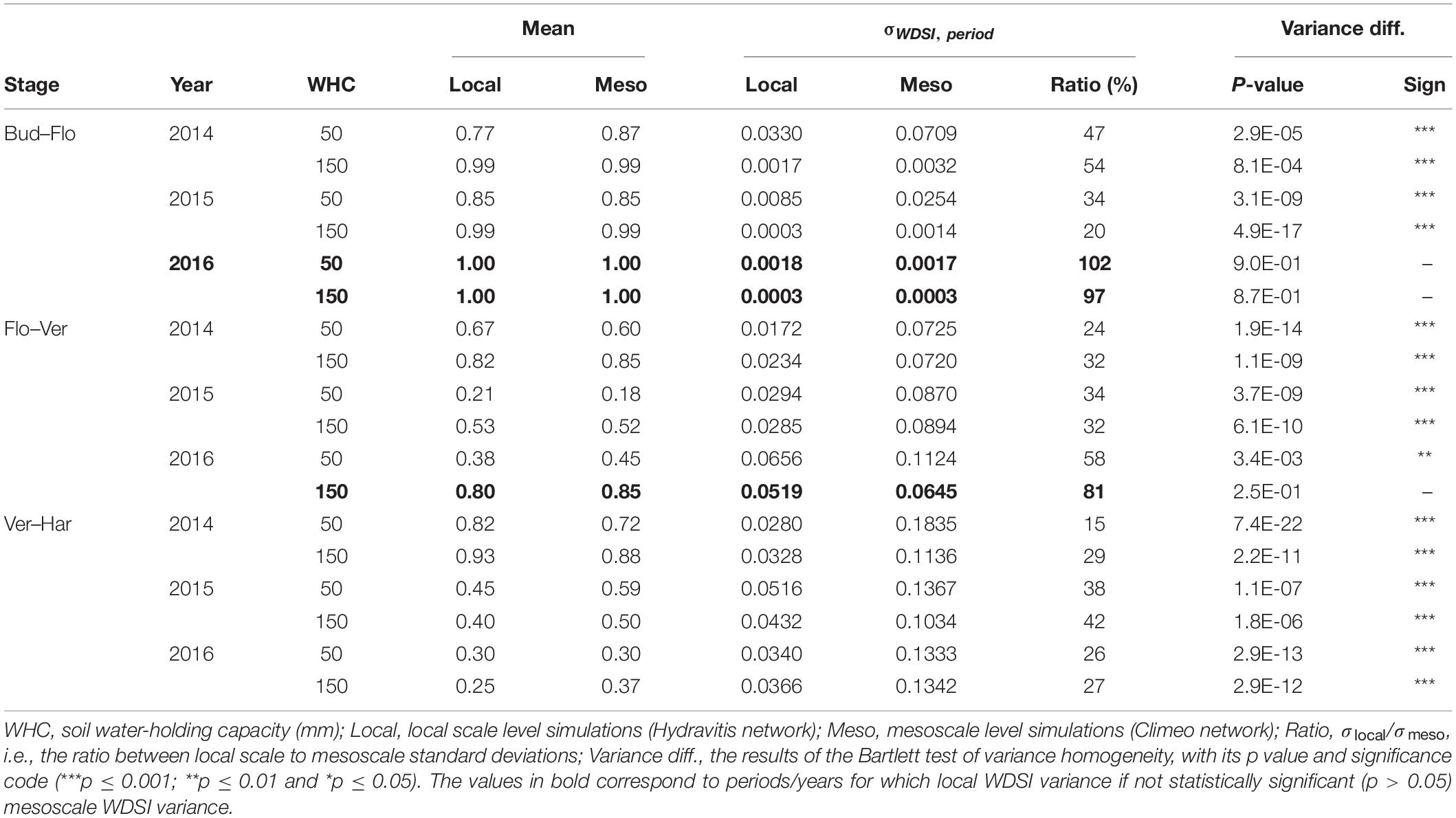
Table 2. Average (mean) and standard deviations (σWDSI, period) of mean WDSI during three development periods of grapevine, in 2014, 2015, and 2016.
In general, WDSI spatial variations were larger at mesoscale than at local scale (Figure 6). To compare spatial variation within each development period at both spatial scales, we calculated the ratio between local and mesoscale WDSI standard deviations, i.e., (local σperiod)/(mesoscale σperiod) in Table 2. In all cases (i.e., year per period per WHC) but one, this ratio is lower than one, indicating that mesoscale climate variability induces more variation in WDSI than local scale rainfall does (Table 2). In three cases, however, the difference in WDSI variance was not significant (Bartlett variance comparison test, at α = 0.05): during the budburst-to-flowering period for both WHC soils in year 2016 and from flowering to veraison for the 150-mm WHC soil in year 2016. The difference in standard deviation for the 2016 budburst-to-veraison period, although significant, is almost null, because WDSI was close to one at all locations and at both local and mesoscale. From flowering to veraison during 2016 for a 150-mm WHC soils, the standard deviation was 0.0519 at local scale and 0.0645 at mesoscale level. The local scale spatial variability was as large as 81% of the mesoscale spatial variability during this period (see the “ratio%” column in Table 2).
Discussion
This article measured the spatial variability of rainfall and estimated the relevance of this phenomenon on the grapevine water status during 3 years. Variation in rainfall was measured to be as large as 43 mm in a single rain event. To evaluate the potential physiological significance of the rainfall variability on grapevine water status, the model developed by Lebon et al. (2003) was used, because it is commonly used in viticulture, and it has shown its efficacy in many viticultural conditions (e.g., Pellegrino et al., 2006; Celette et al., 2010). It is simple to use and produce meaningful outputs to both grape growers and researchers. This model does not use an absolute relationships to link soil water status and grapevine water deficit. In the model, plant water deficit stress depends on the water availability relative to the soil WHC, that is, the FTSW. Water deficit stress index is related to FTSW through an inverse exponential function that led to a rapid decrease in WDSI when FTSW is below 0.4 (Pieri and Gaudillere, 2005). This can sometimes bring to counterintuitive results, when water available to plants is low, rainfall events might be sufficient to refill most of the soil capacity on low WHC soils, whereas they might refill only partly the soil water capacity of higher WHC soils. In this case, the model will simulate higher plant water stress for high WHC soil than for low WHC soil, because in the first case the FTSW would be higher. For example, in 2015, the average WDSI was 0.02 on August 3 for 50-mm SHC soils (2 days before veraison, see Figure 4), whereas it was slightly higher on 150 SHC soils (average WDSI = 0.04). On August 4, 9 mm of rainfall refilled about 17% of the 50-mm WHC soil, whereas only 6% of the 150-mm WHC soil was brought by this rainfall amount. Consequently, the WDSI of the 50-mm WHC soil was, respectively, more than twice as high (0.58) than the 150-mm WHC soil WDSI (0.27). While these situations are not frequent, in the 3 years studied here, they finally led to higher simulated water stress on 150-mm WHC than on the 50-mm WHC soil from veraison to harvest in 2015 and 2016 (at local scale level). This model is constantly under development, it has been improved by Celette et al. (2010) to account for the presence of cover crop and then by Hofmann et al. (2014) to account for slope effect on vineyard radiative balance. However, the relationships between soil water availability and plant water status have never been modified from the one in the original model. In our opinion, a better understanding of the grapevine soil–water relationships is needed and deserves further investigations from soil scientists and plant ecophysiologists.
Variability in vineyard water status can be caused by many factors: soil, terrain, plant material, training system, soil management, and climate. This article makes abstraction of all other factors except soil WHC, to concentrate on the effect of rainfall variability on plant water status. The soil WHCs that were used in this study (50 and 150 mm) are comparable to the upper and lower limits of WHC range previously measured (from 42 to 176 mm) in Burgundy vineyards, at nearby locations (Curmi et al., 2012).
Our results show that at local scale the difference in plant water status caused by the physical environment is related more to soil variability than to rainfall variability. As shown in Figure 4, differences in WDSI are greater between the two soil scenarios than for a given WHC because of differences in rainfall. Differences in soils affect not only the magnitude of the water stress but also the time of occurrence. However, differences in water status between the soil scenarios depend on the meteorological conditions, and they manifest only when rainfall is limited, and differences are not observable at the beginning of the season. Within the study area (see Figure 1C), Brillante et al. (2016a) monitored two grapevine cv Chardonnay grafted on SO4 rootstock subplots, 40 m apart on the same slope, in the same commercial vineyard plot. Stem water potential (Ψstem) measured from two consecutive summers differed from 0.2 MPa on average (with maximum of 0.4 MPa) to 0.1 MPa between the two locations and was largely correlated to soil water content variations. Differences were most probably the result of changes in soil and subsoil characteristics (e.g., depth, texture, gravel content…) or/and rainfall runoff, and probably little affected by changes in rainfall inputs, as both locations compared in their study were 40 m apart. Rainfall spatial variability at local scale level might either enhance or buffer the soil-induced differences on observed grapevine water status. In an empirical model developed in the study area, Brillante et al. (2016b) showed that amounts of rainfall lower than 10 mm in the previous 7 days would linearly reduce Ψstem of 0.02 MPa per mm (average across soil and weather conditions).
The water balance sensitivity was tested at mesoscale level using all climate parameters variations between weather stations, and not rainfall only, contrarily to the local scale water balance modeling experiment. It would have been unrealistic to simulate water balance modeling over the whole Burgundy wine region using the same climate data but rainfall from each weather station, as nonsense data combination would have occurred, such as rain in sunny weather (i.e., high solar radiation and evapotranspiration) conditions. Consequently, mesoscale water balance modeling simulates changes in both grapevine development timing (leaf area and solar radiation interception by rows) and evapotranspiration, whereas these major components of the water balance are kept even at local scale. Spatial variation of rainfall at local scale level induced changes in WDSI, as large as 15–102% of the climate-induced spatial variability at mesoscale level. During the flowering-to-veraison period in 2016, simulated WDSI was as variable in space at local scale as at regional levels.
Within vine growing regions of similar size (about 28 km2, i.e., 2,800 ha), changes in soil management, plant material, training systems, terrain and climate parameters might also either cumulate or compensate the potential changes in vineyard water status as simulated in our study. Lopes et al. (2011) compared the consequences of soil tillage and permanent resident vegetation coverage on soil and grapevine cv. Tempranillo water status in Alentejo (southern Portugal) wine region. Significant differences in FTSW and predawn leaf water potential (ΨPD) were observed within the two soil management systems. ΨPD commonly differed from about 0.1 MPa between the two compared systems, where the soil tillage showed most of the time lower water deficit. Celette (2007) observed up to about 0.3 MPa differences in ΨPD when comparing several interrow grass cover to bare soil on 5- to 7-year-old grapevine cv. Aranel grated with Fercal rootstock. Marguerit et al. (2012) studied the control of scion (cv. Cabernet-Sauvignon) water use from 138 V. vinifera × Vitis riparia rootstock genotypes. While metrics are not comparable to our study, they showed that rootstock material can strongly affect water status of grapevine. For example, scion relative transpiration rate (i.e., WDSI) can show difference up 0.63, depending on the rootstock used. Training systems, through changes in microclimate and incoming solar radiation interception by grapevine (Pieri and Gaudillère, 2003), can considerably affect vineyard transpiration and water use efficiency (Baeza et al., 2005; Reynolds and Heuvel, 2009).
In our study, we focused mainly on rainfall daily depth without accounting for rainfall intensity, which can dramatically affect the water input in soil, depending on runoff.
Runoff is wrongfully often not accounted in water balance modeling.
Yet, it can strongly affect soil water refill. Gaudin et al. (2010) showed that off-season soil water refilling might not be achieved according to rainfall intensity, topography, and soil management (bare soil or interrow grass cover). Biddoccu et al. (2017) observed a reduction of vineyard soil surface runoff of 63% when soil was covered with grass, in comparison to conventional tillage, leading to changes in soil water content. Besides, as runoff is also related to soil permeability; thus, humidity and rainfall spatial variation might affect soil water status through changes in runoff, depending on soil surface texture, structure, and humidity.
Runoff depends on terrain characteristics, among which slope intensity and position. However, slope impact on runoff is not straightforward, as various factor can interact and modify the impact of slope on runoff (Fang et al., 2008). Under intense rainfall, runoff measurements based on simulated rainfall in Mediterranean vineyards showed no impact concerning the slope position on runoff (Cerdà and Rodrigo-Comino, 2020). As runoff is very sensitive to various parameters, its simulation is unsatisfactory when broadly estimated (Chahinian et al., 2005). The data set used in this study could be used in further work to better assess, through hydrological modeling, the potential impact of local rainfall variability on runoff and erosion. Indeed, erosion is a major physical process that leads to land degradation, and soil preservation is a key element of Sustainable Development Goals (Keesstra et al., 2018). As often planted in slopes, grape is a crop for which soil erosion is a major concern (Rodrigo-Comino et al., 2018) and can be considerable in steep slope cool climate grape growing areas such as Burgundy and Germany (Quiquerez et al., 2014; Rodrigo Comino et al., 2016).
Over the study area of our research, terrain might also greatly affect water balance by modifying solar incoming radiation and evapotranspiration. The Lebon et al. (2003) water balance model was strongly enhanced by Hofmann et al. (2014) to account for slope effect on vineyard radiative balance. Their research showed good agreement between simulated and measured FTSW on three plots planted with cv Riesling over the same hillslope in Germany (Rüdesheim region). It suggests that solar radiation partitioning from interrow and row, together with modification in potential (or reference) evapotranspiration by terrain solar radiation interception, soil characteristics, soil management, and training systems variations, influences the changes in grapevine water dynamics. Unfortunately, the study does not compare the relative contribution of these factors to differences in vineyard water status between the validation plots.
Terrain, soil, training systems, and plant material are rather stable through time. When studying a wine producing region, potential water status of grapevine is therefore inferred through these vineyard characteristics. Our simulations indicate that the impact of these vineyard characteristics on plant water status can differ according to local rainfall variations in time and space. According to the water balance modeling performed in our study, a lower soil water capacity can lead to, in a counterintuitive manner, lower water deficit. Water balance simulation in 2015 shows a lower WDSI (i.e., higher water deficit related stress) on 150-mm WHC soils than on 50-mm WHC soils from veraison to harvest (Figure 6, bottom), as rainy events refilled most of the WHC on low WHC soils, whereasthe FTSW remained low on high WHC soils. In the study area, rainfall spatial distribution strongly changes over time. During the three vintages during which rainfall spatial distribution was monitored, no location was preferentially wetter than others were. Consequently, rainfall modifies the zoning of lower or higher water deficit in the study area through time, so that even if several parameters favor a specific water status (e.g., water deficit promoting factors such as low WHC soils, steep slope, considerable leaf area…), the rather odd spatial distribution of rainfall might partially change the spatial distribution of grapevine water deficit at local scale level.
For viticulture, soil is often considered as the sole environmental component that explains grape water status variations and thus the major factor of terroir. Our results show that when comparing spatial structure of grapevine water status at local scale, one should account for the impact of rainfall local variability.
Conclusion
This article shows that spatial variability in rainfall could probably affect grapevine physiology independently from soil or other factors. Differences in rainfall did not have specific spatiotemporal structures in the years and at the scale of this study. Further investigations and sensitivity tests of grapevine water balance modeling to climate, plant material, soil physicochemical properties, soil management, training system, and terrain to better represent the viticultural system diversity would probably be of great interest. It would provide useful contribution to achieve a better understanding of plant water status response to the environment.
To the best of our knowledge, no studies reporting the impact of rainfall spatial variation at local scale on crops has been reported. The issues of rainfall spatial variability impact on small catchment in agriculture are numerous: plant protection, crop quality, mechanization, and soil conservation. The progress in high-resolution radar-aided precipitation detection makes it an interesting source of data to address agricultural water management at local scale level.
Data Availability Statement
The datasets generated for this study are available on request to the corresponding author.
Author Contributions
BP collected and prepared the data. BB and BP analyzed the data. BB wrote the first draft. BP, BB, LB, OM, JL, CV, TC, and YR finalized the manuscript. All authors contributed to the article and approved the submitted version.
Funding
This research was financially supported by Conseil Regional de Bourgogne Franche-Comt and the Bureau Interprofessionnel des Vins de Bourgogne (BIVB). This research was also financially supported by the European Fund FEDER.
Conflict of Interest
The authors declare that the research was conducted in the absence of any commercial or financial relationships that could be construed as a potential conflict of interest.
Footnotes
References
Acevedo-Opazo, C., Ortega-Farias, S., and Fuentes, S. (2010). Effects of grapevine (Vitis vinifera L.) water status on water consumption, vegetative growth and grape quality: an irrigation scheduling application to achieve regulated deficit irrigation. Agric. Water Manag. 97, 956–964. doi: 10.1016/j.agwat.2010.01.025
Ali, M. H., and Talukder, M. S. U. (2008). Increasing water productivity in crop production—A synthesis. Agric. Water Manag. 95, 1201–1213. doi: 10.1016/j.agwat.2008.06.008
Allen, R. G., Pereira, L. S., Raes, D., and Smith, M. (1998). Crop Evapotranspiration: guidelines for Computing Crop Water Requirements. Rome: FAO.
André, F., van Leeuwen, C., Saussez, S., Van Durmen, R., Bogaert, P., Moghadas, D., et al. (2012). High-resolution imaging of a vineyard in south of France using ground-penetrating radar, electromagnetic induction and electrical resistivity tomography. J. Appl. Geophys. 78, 113–122. doi: 10.1016/j.jappgeo.2011.08.002
Baeza, P., Ruiz, C., Cuevas, E., Sotés, V., and Lissarrague, J.-R. (2005). Ecophysiological and agronomic response of tempranillo grapevines to four training systems. Am. J. Enol. Vitic. 56, 129–138.
Bellvert, J., Zarco-Tejada, P. J., Girona, J., and Fereres, E. (2013). Mapping crop water stress index in a ‘Pinot-noir’ vineyard: comparing ground measurements with thermal remote sensing imagery from an unmanned aerial vehicle. Precis. Agric. 15, 361–376. doi: 10.1007/s11119-013-9334-5
Berne, A., Delrieu, G., Creutin, J.-D., and Obled, C. (2004). Temporal and spatial resolution of rainfall measurements required for urban hydrology. J. Hydrol. 299, 166–179. doi: 10.1016/j.jhydrol.2004.08.002
Biddoccu, M., Ferraris, S., Pitacco, A., and Cavallo, E. (2017). Temporal variability of soil management effects on soil hydrological properties, runoff and erosion at the field scale in a hillslope vineyard. North-West Italy. Soil Tillage Res. 165, 46–58. doi: 10.1016/j.still.2016.07.017
Bonfante, A., Agrillo, A., Albrizio, R., Basile, A., Buonomo, R., De Mascellis, R., et al. (2015). Functional homogeneous zones (fHZs) in viticultural zoning procedure: an Italian case study on Aglianico vine. Soil 1, 427–441. doi: 10.5194/soil-1-427-2015
Bonnefoy, C., Quenol, H., Bonnardot, V., Barbeau, G., Madelin, M., Planchon, O., et al. (2013). Temporal and spatial analyses of temperature in a French wine-producing area: the Loire Valley. Int. J. Climatol. 33, 1849–1862. doi: 10.1002/joc.3552
Brillante, L., Bois, B., Lévêque, J., and Mathieu, O. (2016a). Variations in soil-water use by grapevine according to plant water status and soil physical-chemical characteristics—A 3D spatio-temporal analysis. Eur. J. Agron. 77, 122–135. doi: 10.1016/j.eja.2016.04.004
Brillante, L., Mathieu, O., Lévêque, J., and Bois, B. (2016b). Ecophysiological modeling of grapevine water stress in burgundy terroirs by a machine-learning approach. Front. Plant Sci 7:796. doi: 10.3389/fpls.2016.00796
Celette, F. (2007). Dynamique des Fonctionnements Hydrique et azoté dans une vigne Enherbée sous le Climat Méditerranéen. Available at: http://www.agro-montpellier.fr/theses/extranet/07-0009_Celette.pdf (accessed December 11, 2016).
Celette, F., Ripoche, A., and Gary, C. (2010). WaLIS—A simple model to simulate water partitioning in a crop association: The example of an intercropped vineyard. Agric. Water Manag. 97, 1749–1759. doi: 10.1016/j.agwat.2010.06.008
Cerdà, A., and Rodrigo-Comino, J. (2020). Is the hillslope position relevant for runoff and soil loss activation under high rainfall conditions in vineyards? Ecohydrol. Hydrobiol. 20, 59–72. doi: 10.1016/j.ecohyd.2019.05.006
Chabin, J.-P. (2004). L’excellence aux limites… ou le paradoxe des vignobles septentrionaux fran ais d’après l’exemple côte-d’orien. Revue Géographique de l’Est 44, 9–16.
Chahinian, N., Moussa, R., Andrieux, P., and Voltz, M. (2005). Comparison of infiltration models to simulate flood events at the field scale. J. Hydrol. 306, 191–214. doi: 10.1016/j.jhydrol.2004.09.009
Ciach, G. J., and Krajewski, W. F. (2006). Analysis and modeling of spatial correlation structure in small-scale rainfall in Central Oklahoma. Adv. Water Resour. 29, 1450–1463. doi: 10.1016/j.advwatres.2005.11.003
Clothier, B., Dierickx, W., Oster, J. D., Perry, C. J., and Wichelns, D. (2010). Investing in water for food, ecosystems, and livelihoods. Compr. Assess. Water Manag. Agric. 97, 493–494. doi: 10.1016/j.agwat.2009.11.001
Condon, A. G., Richards, R. A., Rebetzke, G. J., and Farquhar, G. D. (2004). Breeding for high water-use efficiency. J. Exp. Bot. 55, 2447–2460. doi: 10.1093/jxb/erh277
Curmi, P., Ayachi, S., Taoutaou, S., Louamba, J., Brayer, J.-M., Guerinot, M., et al. (2012). Characterization of Water Reserve of the Burgundy vineyard soils in the Monitoring of Grape Maturation and for Understanding the Epidemiology of Wood Diseases. Dijon: Université de Bourgogne, 435–437.
de Fraiture, C., and Wichelns, D. (2010). Satisfying future water demands for agriculture. Compr. Assess. Water Manag. Agric. 97, 502–511. doi: 10.1016/j.agwat.2009.08.008
Deloire, A., Carbonneau, A., Wang, Z., and Ojeda, H. (2004). Vine and water: a short review. OENO One 38, 1–13. doi: 10.20870/oeno-one.2004.38.1.932
Duncan, M. R., Austin, B., Fabry, F., and Austin, G. L. (1993). The effect of gauge sampling density on the accuracy of streamflow prediction for rural catchments. J. Hydrol. 142, 445–476. doi: 10.1016/0022-1694(93)90023-3
Fang, H. Y., Cai, Q. G., Chen, H., and Li, Q. Y. (2008). Effect of rainfall regime and slope on runoff in a gullied loess region on the loess plateau in China. Environ. Manage. 42, 402–411. doi: 10.1007/s00267-008-9122-6
Fedoroff, N. V., Battisti, D. S., Beachy, R. N., Cooper, P. J. M., Fischhoff, D. A., Hodges, C. N., et al. (2010). Radically rethinking agriculture for the 21st Century. Science 327, 833–834. doi: 10.1126/science.1186834
Fiener, P., and Auerswald, K. (2009). Spatial variability of rainfall on a sub-kilometre scale. Earth Surf. Process. Landf. 34, 848–859. doi: 10.1002/esp.1779
Finnerty, B. D., Smith, M. B., Seo, D.-J., Koren, V., and Moglen, G. E. (1997). Space-time scale sensitivity of the Sacramento model to radar-gage precipitation inputs. J. Hydrol. 203, 21–38. doi: 10.1016/S0022-1694(97)00083-8
Gaudin, R., Celette, F., and Gary, C. (2010). Contribution of runoff to incomplete off season soil water refilling in a Mediterranean vineyard. Agric. Water Manag. 97, 1534–1540. doi: 10.1016/j.agwat.2010.05.007
Hardie, W. J., and Considine, J. A. (1976). Response of grapes to water-deficit stress in particular stages of development. Am. J. Enol. Vitic. 27, 55–61.
Hargreaves, G. H., and Samani, Z. A. (1982). Estimating potential evapotranspiration. J. Irrig. Drain. Div. 108, 225–230.
Hargreaves, G. H., and Samani, Z. A. (1985). Reference crop evapotranspiration from temperature. Appl. Eng. Agric. 1, 96–99. doi: 10.13031/2013.26773
Hatfield, J. L., Sauer, T. J., and Prueger, J. H. (2001). managing soils to achieve greater water use efficiency. Agron. J. 93, 271–280. doi: 10.2134/agronj2001.932271x
Hofmann, M., Lux, R., and Schultz, H. R. (2014). Constructing a framework for risk analyses of climate change effects on the water budget of differently sloped vineyards with a numeric simulation using the Monte Carlo method coupled to a water balance model. Front. Plant Sci. 5:645. doi: 10.3389/fpls.2014.00645
Howell, T. A. (2001). Enhancing water use efficiency in irrigated agriculture. Agron. J. 93, 281–289. doi: 10.2134/agronj2001.932281x
Keesstra, S., Mol, G., De Leeuw, J., Okx, J., Molenaar, C., De Cleen, M., et al. (2018). Soil-related sustainable development goals: four concepts to make land degradation neutrality and restoration work. Land 7:133. doi: 10.3390/land7040133
Lebon, E., Dumas, V., Pieri, P., and Schultz, H. R. (2003). Modelling the seasonal dynamics of the soil water balance of vineyards. Funct. Plant Biol. 30, 699–710.
Lopes, C. M., Santos, T. P., Monteiro, A., Rodrigues, M. L., Costa, J. M., and Chaves, M. M. (2011). Combining cover cropping with deficit irrigation in a Mediterranean low vigor vineyard. Sci. Hortic. 129, 603–612. doi: 10.1016/j.scienta.2011.04.033
Marguerit, E., Brendel, O., Lebon, E., Van Leeuwen, C., and Ollat, N. (2012). Rootstock control of scion transpiration and its acclimation to water deficit are controlled by different genes. New Phytol. 194, 416–429. doi: 10.1111/j.1469-8137.2012.04059.x
Pauthier, B., Bois, B., Castel, T., and Richard, Y. (2014). Note technique d’implantation d’un réseau de pluviomètres en terrain viticole sur la côte de Beaune (France). Climatologie 11, 34–46. doi: 10.4267/climatologie.570
Pellegrino, A., Gozé, E., Lebon, E., and Wery, J. (2006). A model-based diagnosis tool to evaluate the water stress experienced by grapevine in field sites. Eur. J. Agron. 25, 49–59. doi: 10.1016/j.eja.2006.03.003
Pieri, P., and Gaudillère, J. P. (2003). Sensitivity to training system parameters and soil surface albedo of solar radiation intercepted by vine rows. VITIS - J. Grapevine Res. 42:77.
Pieri, P., and Gaudillere, J.-P. (2005). “Vines water stress derived from a soil water balance model - sensitivity to soil and training system parameters,” in Proceedings of the XIV International GESCO Viticulture Congress, (Geisenheim: Groupe d’Etude des Systemes de COnduite de la vigne), 457–463.
Quénol, H., and Bonnardot, V. (2014). A multi-scale climatic analysis of viticultural terroirs in the context of climate change: the “TERADCLIM” project. J. Int. Sci. Vigne Vin. (Special Laccave) 23–32.
Quiquerez, A., Chevigny, E., Allemand, P., Curmi, P., Petit, C., and Grandjean, P. (2014). Assessing the impact of soil surface characteristics on vineyard erosion from very high spatial resolution aerial images (Côte de Beaune, Burgundy, France). Catena 116, 163–172. doi: 10.1016/j.catena.2013.12.002
Reynolds, A. G., and Heuvel, J. E. V. (2009). Influence of grapevine training systems on vine growth and fruit composition: a review. Am. J. Enol. Vitic. 60, 251–268.
Riou, C., Valancogne, C., and Pieri, P. (1989). Un modele simple d’interception du rayonnement solaire par la vigne. Verification experimentale. Agronomie 9, 441–450. doi: 10.1051/agro:19890502
Rockström, J., Karlberg, L., Wani, S. P., Barron, J., Hatibu, N., Oweis, T., et al. (2010). Managing water in rainfed agriculture—The need for a paradigm shift. Compr. Assess. Water Manag. Agric. 97, 543–550. doi: 10.1016/j.agwat.2009.09.009
Rodrigo Comino, J., Quiquerez, A., Follain, S., Raclot, D., Le Bissonnais, Y., Casalí, J., et al. (2016). Soil erosion in sloping vineyards assessed by using botanical indicators and sediment collectors in the Ruwer-Mosel valley. Agric. Ecosyst. Environ. 233, 158–170. doi: 10.1016/j.agee.2016.09.009
Rodrigo-Comino, J., Keesstra, S., and Cerdà, A. (2018). Soil erosion as an environmental concern in vineyards: the case study of celler del roure, eastern spain, by means of rainfall simulation experiments. Beverages 4:31. doi: 10.3390/beverages4020031
Rossato, L., Alvalá, R. C., dos, S., Marengo, J. A., Zeri, M., Cunha, A. P. M., et al. (2017). Impact of soil moisture on crop yields over brazilian semiarid. Front. Environ. Sci. 5:73. doi: 10.3389/fenvs.2017.00073
Seguin, G. (1986). “Terroirs” and pedology of vinegrowing. Experientia 42, 861–873. doi: 10.1007/bf01941763
Sivakumar, M. V. K., and Hatfield, J. L. (1990). Spatial variability of rainfall at an experimental station in Niger, West Africa. Theor. Appl. Climatol. 42, 33–39. doi: 10.1007/BF00865524
van Leeuwen, C. (2010). “9 - Terroir: the effect of the physical environment on vine growth, grape ripening and wine sensory attributes,” in Managing Wine Quality Woodhead Publishing Series in Food Science, Technology and Nutrition, ed. A. G. Reynolds, (Sawston: Woodhead Publishing), 273–315. doi: 10.1533/9781845699284.3.273
van Leeuwen, C., Tregoat, O., Chone, X., Bois, B., Pernet, D., and Gaudillère, J. P. (2009). Vine water status is a key factor in grapevine ripening and vintage quality for red Bordeaux wine. How can it be assessed for vineyard management process? J. Int. Sci. Vigne Vin 43, 121–134.
Vaudour, E., Costantini, E., Jones, G. V., and Mocali, S. (2015). An overview of the recent approaches to terroir functional modelling, footprinting and zoning. Soil 1, 287–312. doi: 10.5194/soil-1-287-2015
Villarini, G., Mandapaka, P. V., Krajewski, W. F., and Moore, R. J. (2008). Rainfall and sampling uncertainties: a rain gauge perspective. J. Geophys. Res. Atmospheres 113:D11102. doi: 10.1029/2007JD009214
Wang, H., Zhang, L., Dawes, W. R., and Liu, C. (2001). Improving water use efficiency of irrigated crops in the North China Plain — measurements and modelling. Agric. Water Manag. 48, 151–167. doi: 10.1016/S0378-3774(00)00118-9
Ward, F. A., and Michelsen, A. (2002). The economic value of water in agriculture: concepts and policy applications. Water Policy 4, 423–446. doi: 10.1016/S1366-7017(02)00039-9
Keywords: water balance, grapevine, rainfall, local scale, Burgundy, terroir
Citation: Bois B, Pauthier B, Brillante L, Mathieu O, Leveque J, Van Leeuwen C, Castel T and Richard Y (2020) Sensitivity of Grapevine Soil–Water Balance to Rainfall Spatial Variability at Local Scale Level. Front. Environ. Sci. 8:110. doi: 10.3389/fenvs.2020.00110
Received: 01 December 2019; Accepted: 26 June 2020;
Published: 04 August 2020.
Edited by:
Pascal Boivin, University of Applied Sciences and Arts Western Switzerland, SwitzerlandReviewed by:
Zamir Libohova, United States Department of Agriculture (USDA), United StatesArtemi Cerdà, University of Valencia, Spain
Copyright © 2020 Bois, Pauthier, Brillante, Mathieu, Leveque, Van Leeuwen, Castel and Richard. This is an open-access article distributed under the terms of the Creative Commons Attribution License (CC BY). The use, distribution or reproduction in other forums is permitted, provided the original author(s) and the copyright owner(s) are credited and that the original publication in this journal is cited, in accordance with accepted academic practice. No use, distribution or reproduction is permitted which does not comply with these terms.
*Correspondence: Benjamin Bois, YmVuamFtaW4uYm9pc0B1LWJvdXJnb2duZS5mcg==