- 1Department of Soil and Environment, Swedish University of Agricultural Sciences, Uppsala, Sweden
- 2Department of Ecology, Swedish University of Agricultural Sciences, Uppsala, Sweden
Northern peatlands are important carbon (C) reservoirs, storing about one-third of the global terrestrial soil C pool. Anthropogenic influences, such as drainage for agriculture and forestry, lower the originally high groundwater level, leading to peat aeration and decomposition. This is particularly reflected in significant losses of CO2, while fluxes of N2O and CH4 are generally considered of minor importance for the overall greenhouse gas (GHG) balance of cultivated peatlands in Scandinavia. Setting land aside from agricultural production has been proposed as a strategy to reduce GHG emissions from drained peatland, restore natural habitats, and increase C sequestration. However, the evidence for this is rather scarce unless drainage is terminated. In this study, we measured respiration using dark automatic chambers, and CO2, N2O, and CH4 fluxes using manual static chambers, on: 1) cultivated peatland and 2) adjacent set-aside peatland in Central Sweden. The set-aside site was found to be a greater source of respiration than the cultivated site, while higher N2O fluxes and lower CH4 uptake rates were observed for the cultivated site. However, to compare the full GHG balance and assess the abandonment of drained cultivated peatland, additional measures, such as gross primary production (GPP) but also dissolved organic C losses would have to be taken into account.
Introduction
During the Holocene, northern peatlands stored large amounts of carbon (C) (Yu et al., 2009). In a global perspective, they now represent about one-third of the total soil carbon pool (Joosten and Clarke, 2002). Under natural conditions, most peatlands still sequester carbon dioxide (CO2) from the atmosphere (Gorham 1991), but at the same time emit substantial quantities of C as both CO2 and methane (CH4) (Moore and Knowles 1987, 1989). Apart from temperature, the water balance of peat soil is generally regulating decomposition, with decomposition occurring faster in the well-aerated part of the peat above the water table (Silvola et al., 1996; Scanlon and Moore 2000). In several studies, a raised water level has been found to reduce CO2 and nitrous oxide (N2O) fluxes, but to increase the emissions of CH4 (Van de Riet et al., 2013; Regina et al., 2015).
Large areas of peatland are subject to anthropogenic influences and, according to Joosten and Clarke (2002), up to 20% of total global peatland area has been drained for agriculture and forestry. Drained peatlands subside due to oxidation of the organic material, but also due to consolidation, shrinkage, wind and water erosion and compaction (Eggelsman 1972; Heathwaite et al., 1993; Berglund, 1996). Tillage and fertilization can increase decomposition rates caused by higher eration (Glenn et al., 1993; Maljanen et al., 2007). Drainage, in combination with the naturally high organic C concentration in peat soil, leads to increased C losses in the form of CO2 emissions (e.g., Joosten and Clarke, 2002; Lohila et al., 2004; Joosten 2009; Frolking et al., 2011; Salm et al., 2012; Ballantyne et al., 2014). Moreover, these highly elevated CO2 emissions persist for decades after drainage of peat soil (e.g., Koizumi et al., 1999; Lohila et al., 2003). The global potential of peatland restoration in reducing GHG emissions is therefore estimated to be very high, 0.31–3.38 Gt CO2-equivalents according to Leifeld and Menichetti (2018).
In Sweden, peatlands of varying thickness cover approximately 25% of the land area (Fredriksson 1996). Around 8.6% of Swedish agricultural soils are classified as agricultural organic soils (peat and gyttja) (Berglund and Berglund, 2010), which are considered to be ‘hotspots’ of greenhouse gas (GHG) emissions. Despite comprising a small proportion of total agricultural area, cultivated organic soils in Sweden and Finland are considered to be major sources of CO2 and N2O emissions (Kasimir-Klemendtsson et al., 1997; Maljanen et al., 2010). Enhanced nutrient availability through fertilizer application and drainage has been shown to increase N2O emissions (Alm et al., 2007; Teh et al., 2011; van Beek et al., 2011; Salm et al., 2012). Emissions of CH4 have been found to decrease due to soil eration and management, suggesting that cultivated peat soils may even act as CH4 sinks (Kasimir-Klemendtsson et al., 1997; Maljanen et al., 2003; Smith and Conen 2004; Maljanen et al., 2010; Schrier-Uijl et al., 2014; Knox et al., 2015; Wang et al., 2017). Because of their comparatively low fluxes, N2O and CH4 are reported to play only a minor role in the overall GHG balance of cultivated peat soils in Northern Europe (e.g., Maljanen et al., 2004; Grönlund et al., 2006; Berglund and Berglund, 2010; Karki et al., 2015). However, most studies agree that conversion of wetlands for agriculture leads to significant net losses of CO2. Lamb et al. (2016) suggested that land sparing, i.e., setting land aside from agriculture, could have the potential to reduce GHG emissions from agricultural land, through concentrating crop production to intensively managed sites, while restoring natural habitats on the abandoned land and increasing C sequestration. Permanent vegetation on abandoned peatland sites would provide year-round soil cover and enhanced photosynthesis compared with seasonal agricultural crops. This could partially compensate for C losses induced e.g., by the lower water table (Hendriks et al., 2007; Shurpali et al., 2009). However, information about the effects of abandoning or setting aside drained peatlands from agricultural use is scarce. In a study in Newfoundland, Wang et al. (2018) found that an abandoned peatland pasture sequestered more CO2 from the atmosphere than an adjacent undisturbed bog. On including emissions of N2O and CH4, Wang et al. (2020) found that the same abandoned pasture had a cooling effect, primarily resulting from changes in CO2 and CH4, while the bog acted as nearly climate-neutral. Based on these findings, the authors reiterated the suggestion that abandoning cultivated peatland sites could mitigate CO2 emissions. In contrast, Maljanen et al. (2007) found that abandoned organic soils in Finland showed slight net CO2 uptake during the growing season, but were CO2 sources outside that period, and that mean annual net CO2 emissions from those soils were close to those from cultivated cropland. Similarly, Hadden and Grelle (2017) found that a Swedish peatland site under cultivation was a small net C sink, while a previously cultivated site that had been abandoned for 25 years was a C source.
The aim of the present study was to assess the emissions of CO2, N2O, and CH4, from both a cultivated and a set aside organic soil. The study was conducted at two neighboring sites in a peatland area in Central Sweden that was drained in 1878 for agricultural use (Nerman 1898). One of these sites was set aside in the early 1990s, which means that agricultural management has been stopped. However, this did not involve closing the ditches or rising the water table. The other site is still used for agricultural crop production, mainly spring cereals.
Materials and Methods
Study Site
Two experimental sites, cultivated (Cult) and set-aside (SA) were established at the margin of a natural peatland area about 30 km north of Uppsala in Central Sweden (Figure 1). The climate in this area is cold-temperate (Dfb according to the Köppen-Geiger classification) with mean annual temperature of 5.6°C and mean annual precipitation of 544 mm.
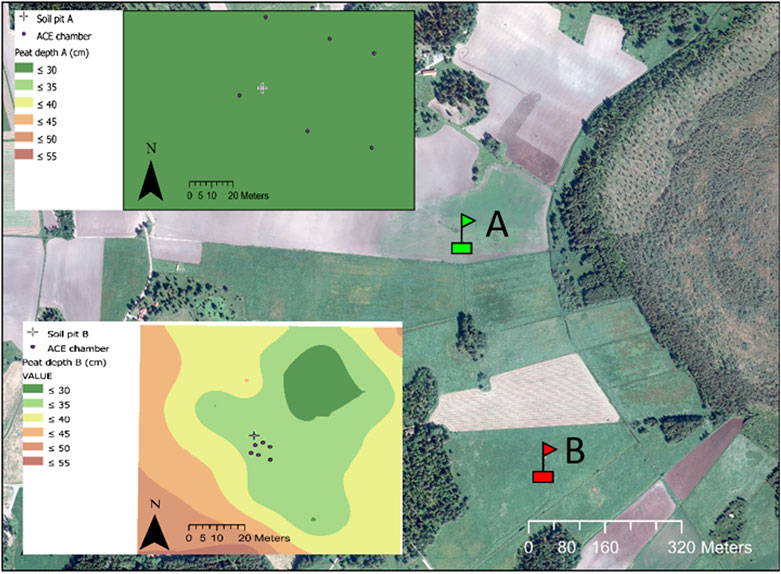
FIGURE 1. Map showing the location of the cultivated peatland site (soil pit A, green flag) and the set-aside site (soil pit B, red flag) and (inserts) showing peat depth and the positions of the soil pits and automatic chambers (ACE) at each site.
The area underwent large-scale drainage during lowering of Lake Tämnaren in 1878 (Nerman 1898), which also affected the two study sites. The cultivated site (60.0835°N, 17.233°E) was cropped with spring cereals during the study period (2013–2016), to produce standing forage for wild birds. The crop was not harvested, but the site was tilled and re-sown every spring. Prior to the study period, annual crops had been grown in rotation with grass leys (4 out of 10 years) supplying forage for dairy cows. Manure was applied and complemented with small amounts of nitrogen fertilizer during that period. The set-aside site (60.079°N, 17.236°E) was established 0.5 km south of the cultivated site and had been set aside (under permanent grassland) for more than 20 years prior to the start of the study. During the study period, the grass growing at the site was occasionally cut once a year and left on the field.
Soil properties were analyzed at the two sites (Table 1). Total nitrogen (Ntot) and total carbon (Ctot) content were determined by dry combustion on a LECO CN-2000 analyzer (St. Joseph, MI, USA). Soil pH and electrical conductivity (EC) were measured at a soil:solution ratio of 1:5 with deionized water. Four replicate undisturbed soil cores (7.2 cm diameter, 10 cm high) from each soil depth were used for determination of dry bulk density and volumetric water content at suction heads of 0.05, 0.3, 0.6, 1.0 and 6.0 m water column (approximately 0.5, 3.0, 6.0, 10.0, and 60.0 kPa). Physical wilting point (water content at 150 m water column) and particle density were determined on disturbed soil samples. Porosity was calculated from particle and dry bulk densities. Shrinkage was not considered when calculating water content at different tensions. Saturated hydraulic conductivity was determined using a constant head method (unit hydraulic gradient) on another set of undisturbed soil cores (4 replicates). Humification degree (H1-H10) of the peat was determined according to von Post (1922). The water table was 0.5 m below the soil surface at both sites at the time of soil sampling in October 2014.
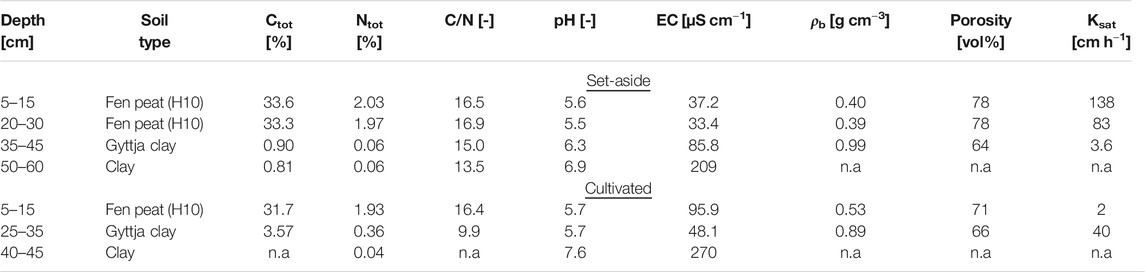
TABLE 1. Soil properties at the set-aside and cultivated study sites. Soil sampling was performed on bare soil at the set-aside site, after removing the grass sward. All values are means (n = 4). H10 = humification degree (von Post, 1922), Ctot = total carbon content, Ntot = total nitrogen content, soil pH was measured at a soil:solution ratio of 1:5 with deionized water, EC = electric conductivity, ρb = dry bulk density, Ksat = saturated hydraulic conductivity, n.a. = no measurements available.
Climate Data
Abiotic variables measured included air temperature, precipitation, and global radiation, which were taken from the nearest official meteorological station (Kerstinbo; 60.270°N, 16.980°E).
Gas Sampling Set-Up
Measurements were performed every year between 2012 and 2016. During that time, gas measurements using dark chambers were taken in 2012, 2013, 2015, and 2016, while CO2 measurements with automatic chambers were taken in 2013, 2014, and 2015. The duration and intensity of measurements varied between the years.
To estimate root respiration at the set-aside site, the grass sward was removed (SA-bare) and the emissions were compared with those from vegetated plots (SA-veg).
Manual Measurements
Manual measurements were made using the closed chamber method (e.g., Flessa et al., 1995) and following Norberg et al. (2016a, 2016b). In brief, at each site six PVC rings with a base area of 0.07 m2 were inserted into the soil to approximately 5 cm depth, to act as anchors for the chambers. To measure soil-atmosphere exchange, opaque chambers (V = 0.009 m3) were set on the rings and sealed with rubber seals. On plots with vegetation, the crop was cut prior to the measurements. Soil and plant (mainly root) respiration was measured in two ways: 1) using a portable infrared analyzer, where measurements were performed every 5 s over a period of 3–5 min (Norberg et al., 2016b); and 2) together with N2O and CH4, using vial sampling and gas chromatography. For days when both methods were used, CO2 fluxes are presented as the mean of both methods. For N2O and CH4, the headspace was sampled by circulating the air between the chamber and 22 ml vials for approximately 30 s (Norberg et al., 2016a). Gas samples were taken at 0, 10, 20, and 30 min after closure, and analyzed in the laboratory using gas chromatography (Perkin Elmer Clarus 500, USA). Gas fluxes were calculated using the HMR approach (Pedersen et al., 2010), which is based on the model of Hutchinson and Mosier (1981). Depending on the trend in the concentration data over time, the fluxes were calculated by either a linear or non-linear function. “No flux” is recommended by the algorithm when the concentration data are noisy and do not show a clear trend. In our dataset, this was the case for 25% of the N2O fluxes and 21% of the CH4 fluxes, and those were set to zero. In total, 18% and 38% of the fluxes were calculated using the non-linear function for N2O and CH4, respectively, while for the remaining fluxes a linear function described the trend best.
Soil moisture (volumetric water content) and soil temperature at 5 cm depth were measured next to each chamber at each measurement event, using a WET sensor (Delta-T) connected to a HH2 moisture meter read-out unit. The air-filled pore space (AFPS) was calculated using the volumetric water content and soil porosity.
Automatic Measurements
Continuous observations of soil and plant respiration (vegetated plots, SA-veg), and of peat oxidation (vegetation cover and roots removed; bare soil, SA-bare), were made using Automatic Soil CO2 Exchange (ACE) stations (ADC BioScientific Limited, Hertfordshire, United Kingdom). Each station consists of an electronic box and a motorized moveable arm that contains an infrared gas analyzer. Besides measurements of soil respiration, the stations were used to record soil temperature at 5 and 10 cm depth (range: −10° to 50°C), and moisture at 5 cm depth measured as voltage (range 0–2 V), which was converted to volumetric water content. The area of soil analyzed was defined by a stainless steel soil collar (23 cm in diameter) which was inserted into the soil to a depth of 5 cm. To perform CO2 measurements, the collar was automatically covered by a sealed lid enclosing a volume of 2.6 L. Internal flux was estimated based on the change in CO2 concentration in the chamber during the closure time, by fitting either a linear or a non-linear function to the concentration data. After measurement, the lid was automatically removed from the collar and the system was left open to the environment. Measurements were performed at hourly intervals. The closure time depended on the change in concentration (maximum 10 μmol m−3), but was never longer than 5 min.
Statistical Analysis
The data were analyzed using the R software (R i386 3.3.1, R Core Team 2016). For data processing, the openxlsx package (Schauberger and Walker, 2019) and the ddply function (plyr package, Wickham 2011) were used. Gas fluxes were calculated using the HMR package (Pedersen 2017). Differences between the sites and years were determined by analysis of variance (ANOVA) (Type-III error, car package; Fox and Weisberg 2019) and tested by Honest Significant Difference test (HSD.test in the agricolae package; de Mendiburu 2017). Differences were considered significant at p < 0.05. Diagrams were created using the ggplot2 (Wickham 2009) and cowplot (Wilke 2017) packages. Pearson correlation coefficient (r) was used to determine linear correlations between manually measured gas fluxes and simultaneously measured soil temperature, and between manually measured gas fluxes and the interaction between soil temperature and water content (calculated as soil temperature [°C] x water content [%]). Outlier analysis was carried out using the outliers package (Komsta 2011).
Results and Discussion
The peat layer (average of nine measurements) was shallower at the cultivated site (25 cm) than at the set-aside site (32 cm). The shallower peat layer at the cultivated site may be due to initial differences in peat depth or cultivation, which may have caused erosion and/or faster decomposition of the peat. It can be assumed that the original peat layer, i.e., before drainage of the area in 1878 (Nerman 1898), was much thicker and that the peat surface was at higher elevation. Subsidence rates due to agricultural drainage of peat soils in Nordic conditions can vary from 0.5 cm yr−1 on fields with pasture to 2.5 cm yr−1 on fields with row crops (Berglund 1996). Subsidence rates in this range (1–3 cm yr−1) have been reported by e.g., Armentano (1980). The proportion of subsidence attributable to oxidation is estimated to range between 13 and 90% (Armentano and Menges 1986; Joosten and Clarke, 2002). For Swedish conditions, a value of 35% has been used as an average for all cultivated peat soils (Berglund and Berglund, 2010). Varying subsidence rates of fields under different crops have been taken as an indication that GHG emission rates vary due to the crop grown (Kasimir-Klemendtsson et al., 1997). In the present study, this would mean much higher emissions from the cultivated field compared with the set-aside field with permanent grass vegetation.
Manual Measurements
CO2
The measured CO2 fluxes varied between sites and years. Median fluxes (2012–2016) were significantly higher from SA-veg and SA-bare (743 and 791 mg m−2 h−1, respectively) than from the cultivated site (415 mg m−2 h−1) (Tables 2 and 3). No significant differences between SA-veg and SA-bare were found (Table 3). According to the IPCC (2013) emission factors, CO2 emissions should be much lower from the set-aside site (grassland, drained, boreal: 5.7 t CO2-C ha−1 yr−1) compared with the cultivated site (cropland, drained, boreal: 7.9 t CO2-C ha−1 yr−1), but this was not confirmed by our data.
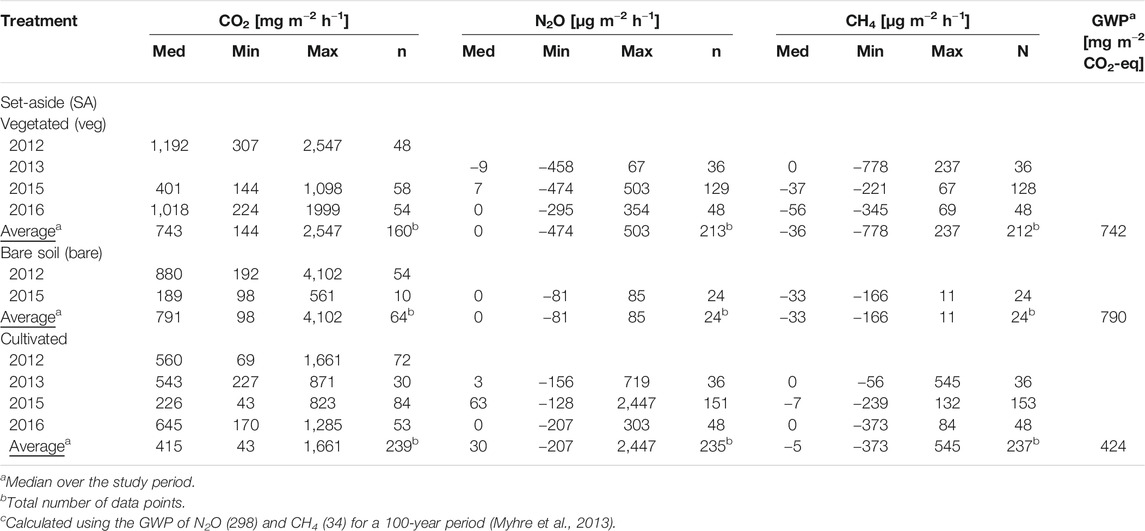
TABLE 2. Ecosystem respiration, nitrous oxide (N2O) and methane (CH4) fluxes measured manually at the set-aside and cultivated sites between 2012 and 2016. Values shown are median (Med), minimum (Min), and maximum (Max). n = number of data points. GWP = Global Warming Potential.
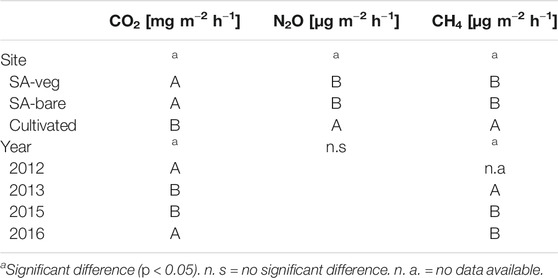
TABLE 3. Differences in greenhouse gas (GHG) fluxes determined by manual measurements between the set-aside (SA) and cultivated sites and between years, based on analysis of variance (ANOVA) and Honest Significant Difference test. Different letters indicate significant differences between sites/years.
Plant-derived CO2, estimated as the difference between CO2 fluxes from simultaneously measured vegetated plots and bare soil, was 37% (Table 4). This is within the range reported by Norberg et al. (2016b) (27%) and Berglund et al. (2011) (27–63%) for different cultivated and non-cultivated organic soils in southern and central Sweden. Berglund et al. (2011) identified seasonal variation in the plant contribution to CO2 emissions, with the lowest contribution in spring (27%) and the highest in autumn (57%). Based on our limited dataset (17 data points in total), average plant-derived CO2 fluxes were lower in summer than in spring and autumn (Table 4). This can be explained by the significantly higher CO2 fluxes from both SA-veg and SA-bare during summer (p < 0.01).
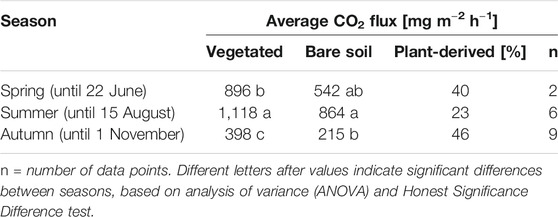
TABLE 4. Season-specific average plant-derived carbon dioxide (CO2) flux [mg m−2 h−1] at the set-aside site in seasons covered by manual measurements in 2012 and 2015.
Soil temperature and soil eration status had a strong impact on formation and release of CO2, and emissions increased with increasing temperature (Figure 2). The correlation with temperature was significant for SA-veg (r = 0.60) and the cultivated site (r = 0.27), but not for SA-bare (r = 0.16). The increase in CO2 fluxes with increasing air-filled pore space (AFPS) in the soil was significant only for the bare soil (r = 0.45) (data not shown). The relationship between CO2 emissions and the interaction of soil temperature and water content (soil temperature x water content) was also significant only for SA-bare (r = -0.31).
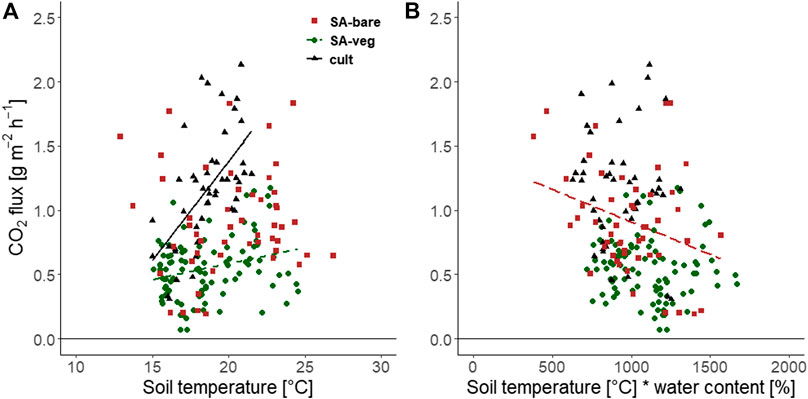
FIGURE 2. Carbon dioxide (CO2) flux [g m−2 h−2] as a function of (A) soil temperature at 5 cm depth and (B) the interaction between soil temperature and volumetric water content, at the set-aside site (SA-bare (▪), SA-veg (○)) and the cultivated site (cult, ▲). Significant correlations (p < 0.05) are shown by the respective regression line.
N2O
Median fluxes of N2O from the cultivated site (30 μg m−2 h−1) were significantly higher than from the set-aside site (Table 2). The fluxes from SA-veg were on average negative in 2013, but were positive in 2015 and zero in 2016. The bare soil (SA-bare) showed zero N2O fluxes on average. This was mainly due to zero fluxes during summer and autumn, while fluxes were negative during spring (−39 μg m−2 h−1; Table 5). In SA-veg, the N2O fluxes were similar in spring and autumn (7 and 5 μg m−2 h−1, respectively) and were slightly, but not significantly, higher in summer (14 μg m−2 h−1). At the cultivated site, N2O fluxes were not significantly different during spring and summer (141 and 288 μg m−2 h−1, respectively), but were significantly lower (p < 0.05) in autumn (27 μg m−2 h−1).
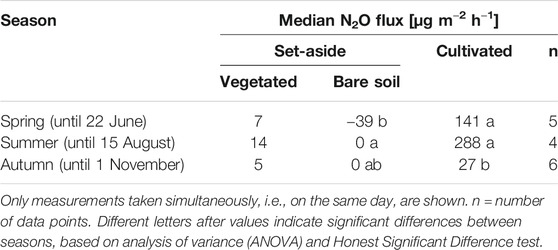
TABLE 5. Season-specific median nitrous oxide (N2O) flux [µg m−2 h−1] from the set-aside site (SA-bare and SA-veg) and the cultivated site.
In general, N2O fluxes showed large temporal and spatial variation and both consumption and emission were observed at all sites, irrespective of the vegetation. Absence of plants could be assumed to result in more available nitrogen (N) and, consequently, higher N2O emissions, due to lack of N uptake by plant roots. However, we found that vegetated plots acted as N2O sources, while fluxes were on average negative for bare soil plots (Table 2). Johansson et al. (2011) found a negative correlation between N2O consumption and nitrate (NO3−) concentration, suggesting that consumption is linked to NO3− deficiency (lack of electron acceptors) and that denitrifying bacteria use N2O as a substitute. Although it can be assumed that denitrification was the main process for N2O production at all sites in this study, the negative fluxes found for SA-bare indicate reduction of N2O to molecular nitrogen (N2) in the final step of the denitrification process. Based on our dataset, decreasing soil moisture, and thus increasing AFPS, significantly decreased N2O emissions at the cultivated site (r = −0.25). Furthermore, we found no relationship with soil temperature or with the interaction between soil temperature and soil moisture at either SA-veg or the cultivated site, which suggests that other factors influence the formation and emission of N2O from the soil (Figure 3). Although crop management was quite extensive at the cultivated site, its history of manure application and the consequences of tillage in late spring, i.e., before the measurements started, might still be visible in the observed N2O emissions.
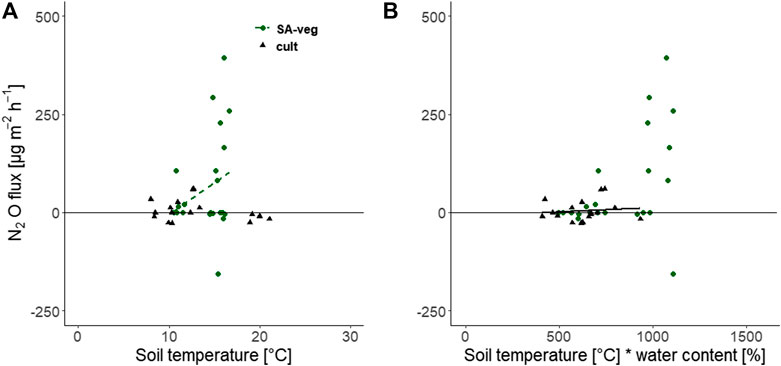
FIGURE 3. Nitrous oxide (N2O) flux [µg m−2 h−1] as a function of (A) soil temperature at 5 cm depth and (B) the interaction between soil temperature and volumetric water content, at the set-aside site (SA-veg (○)) and the cultivated site (cult, ▲). No significant relationship was found.
CH4
Both the cultivated and the set-aside site were found to be a sink for CH4 and the latter acted as a sink irrespective of the vegetation (Table 2). In 2013, average fluxes were zero from both the set-aside and the cultivated site. In contrast, there was net uptake of CH4 in 2015 on both sites and in 2016 on the set-aside site. Small and mostly negative fluxes from drained peat soils have been observed in many studies in Sweden (e.g., Kasimir-Klemedtsson et al., 2009; Berglund and Berglund, 2010), Finland (e.g., Nykänen et al., 1995; Maljanen et al., 2003), Canada (Glenn et al., 1993), and Estonia (Salm et al., 2012).
In the present study, CH4 uptake rates were significantly higher at the set-aside site (36 μg m−2 h−1) than at the cultivated site (5 μg m−2 h−1). Although CH4 uptake by SA-bare (33 μg m−2 h−1) was on average only slightly lower than that by SA-veg, the latter showed greater variation, with both high emissions (237 μg m−2 h−1) and high uptake rates (778 μg m−2 h−1). The function of all sites as zero emissions or even a net sink for CH4 is likely to be related to the shallow peat layer, soil drainage, and the associated lowering of the water table. In the presence of O2, CH4 is consumed by methanotrophs for energy production and growth (Lai 2009). Methanotrophic activity in peatland soils is highest at shallower depths and in the boundary between the aerobic and anaerobic zones, where the ratio of CH4 to O2 is optimal (Dedysh 2002; Lai 2009). Consequently, rising water levels, e.g., as a consequence of poor drainage, have been found to transform peat soils from a net sink into a net source of CH4 (e.g., Regina et al., 2007, 2015). The peat soil at the set-aside site was less compacted than that at the cultivated site, with lower dry bulk density and higher porosity and saturated hydraulic conductivity (Table 1). This difference in aeration and oxidizing conditions may explain the higher uptake of CH4 at the set-aside site compared with the cultivated site.
We found no significant correlation between CH4 flux and soil temperature (Figure 4A). However, CH4 fluxes decreased with increasing AFPS in both SA-bare and the cultivated site (data not shown), but this relationship was not significant at either of the sites. Similarly, Kasimir-Klemedtsson et al. (2009) found positive CH4 fluxes in wetter areas, and CH4 uptake in drier areas, of a cultivated Histosol in southern Sweden. In agreement with Kasimir-Klemedtsson et al. (2009), we did not find a significant correlation with soil temperature or with the interaction between soil temperature and water content. Lack of correlation between CH4 flux and soil parameters may be explained by low emissions levels and fluctuations around zero (Kasimir-Klemedtsson et al., 2009).
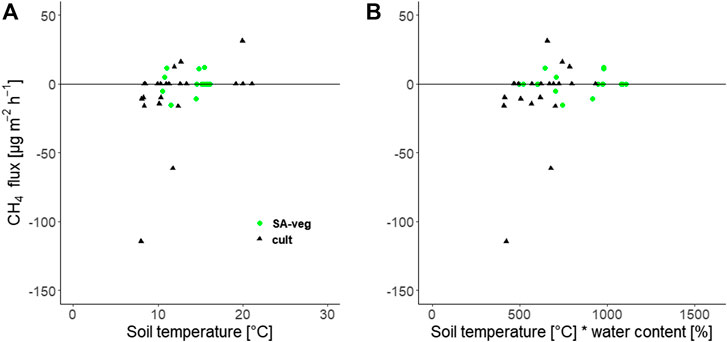
FIGURE 4. Methane (CH4) flux [µg m−2 h−1] as a function of (A) soil temperature at 5 cm depth and (B) the interaction between soil temperature and volumetric water content, at the set-aside site (SA-veg (○)) and the cultivated site (▲). No significant relationship was found.
Using the eddy covariance technique at the set-aside site, Hadden and Grelle (2017) observed higher CH4 uptake rates during the summer months, while spring and winter usually had small but positive fluxes. In general, those authors found that CH4 exchange rates were close to zero for air temperatures below freezing, and that higher soil temperatures led to higher uptake rates. On comparing manual measurements made simultaneously in all study plots, we did not find any significant differences between the seasons (Table 6). However, uptake rates at the set-aside site were on average highest in autumn (irrespective of vegetation), while uptake rates at the cultivated site were highest in spring and similar in summer and autumn (Table 6).
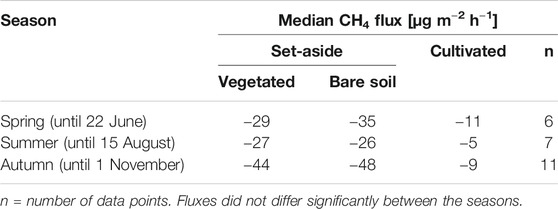
TABLE 6. Season-specific median methane (CH4) flux [µg m−2 h−1] from the set-aside site (bare soil and vegetated) and the cultivated site.
Automatic Chambers
Respiration measurements using automatic chambers were made between 2013 and 2015. Comparing the three years based on the month of July, which was covered in all three years, the average temperature was higher in 2014 (20°C) than in 2013 (17°C) and 2015 (15°C). However, the summers in 2013 and 2014 were rather dry, with rainfall in July of 25 and 16 mm, respectively. In contrast, 2015 was characterized by a wetter summer, with 105 mm of rain in July (Figure 5).
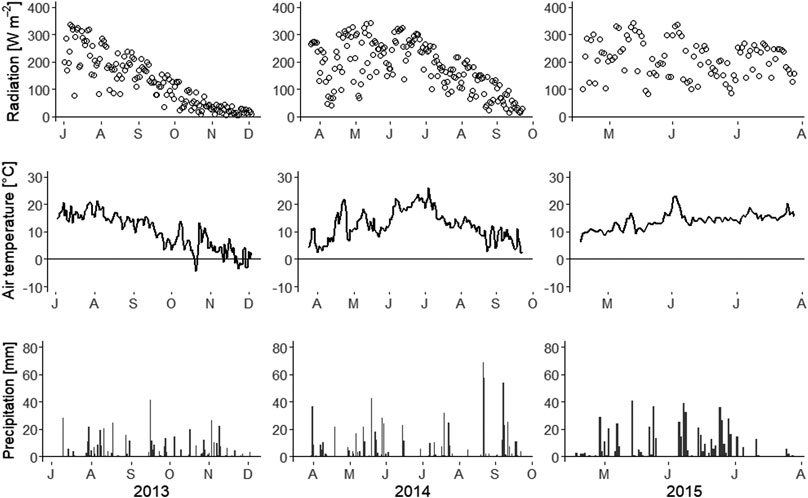
FIGURE 5. Daily global radiation (top), air temperature (middle), and precipitation (bottom) in 2013, 2014, and 2015, measured at the nearest official meteorological station (Kerstinbo) to the study site.
Measured CO2 followed a seasonal trend, with higher emissions during the summer months and decreasing emissions as winter approached (Figure 6).
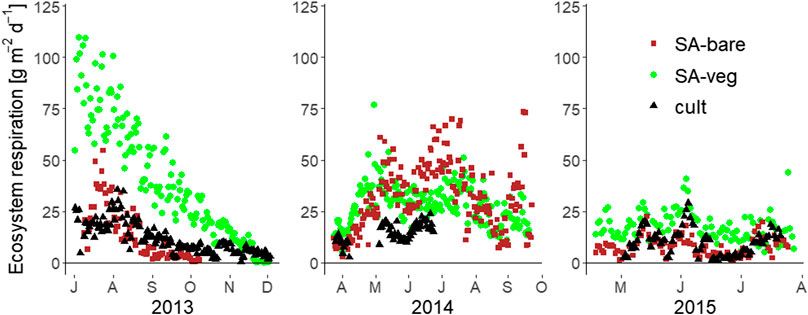
FIGURE 6. Daily ecosystem respiration [g m−2] measured by automatic chambers at the set-aside site (SA-bare and SA-veg) and the cultivated site (cult) in July-December 2013, April-October 2014, and May-August 2015.
In July, CO2 fluxes were higher from the vegetated set-aside plots than from the bare set-aside plots or the cultivated site (Table 7). One explanation for this difference might be higher C availability at the set-aside site due to the thicker peat layer, slightly higher soil C content, and greater biomass of vegetation compared with the cultivated site (Hadden and Grelle 2017).
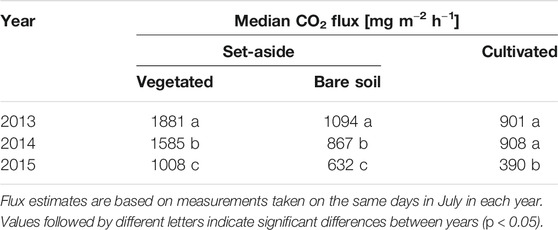
TABLE 7. Daily ecosystem respiration [mg m−2 h−1] in July, determined using automatic chambers, in 2013 (n = 23), 2014 (n = 24), and 2015 (n = 30).
Soil respiration from the bare soil plots (859 mg m−2 h−1) was around 55% of ecosystem respiration (vegetated plots, 1,575 mg m−2 h−1) when averaged over all three years. In individual years, soil respiration was 45% (2013), 52% (2014), and 37% (2015) of ecosystem respiration. These estimates are lower than the values obtained using the manual chambers, according to which soil respiration accounted for 63% of ecosystem respiration (total CO2 flux). Similar estimates (62%) were made by Lohila et al. (2003) for a Finnish peat soil in the middle of the summer. Measurements made within controlled lysimeter experiments with ryegrass on a similar peat soil estimated the contribution of soil respiration to total summer-time CO2 emissions to be 46% (Berglund et al., 2011). Our values are also slightly lower than estimates made in other studies using eddy covariance and chambers, according to which soil respiration accounted for 68–80% of total ecosystem respiration (Goulden et al., 1996; Law et al., 1999; Granier et al., 2000; Davidson et al., 2002). Generally, these rates typically vary throughout the year and can be expected to be lower during the growing season and higher during the non-growing season. For example, Bolstad et al. (2004) found that soil respiration was more than 60% of total ecosystem respiration during the growing season and more than 90% during the non-growing season. However, these estimates relate to forests and forest soils, respectively. The proportion of bare soil respiration estimated in this study (55%) relates to the growing season and the month of July. Moreover, it only relates to the set-aside site, since based on the data available we were unable to make an estimate for the cultivated site. The generally lower respiration observed at the cultivated site might be due to e.g., the shallower peat layer and lower root biomass, leading to less heterotrophic and autotrophic respiration, respectively.
As expected, the variation in ecosystem respiration over single days was rather moderate, but average fluxes increased slightly throughout the morning and decreased toward the evening. In general, measured fluxes were higher at higher soil temperatures (Figures 7A,B). No such clear correlation was seen for changes in AFPS (Figures 7C,D). The fluxes determined using the automatic chambers are in good agreement with the respective fluxes captured by eddy covariance at the same two sites and over the same period (Hadden and Grelle 2017; data not shown).
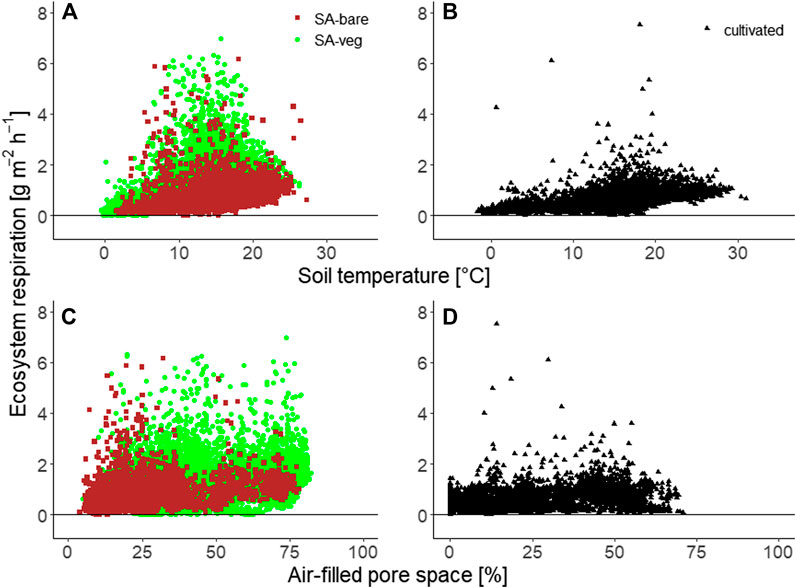
FIGURE 7. Ecosystem respiration [g m−2 h−1] measured by automatic chambers at (A,C) the set-aside site and (B,D) the cultivated site, as a function of (A,B) soil temperature at 5 cm depth and (C,D) air-filled pore space.
Considering both environmental factors simultaneously revealed that higher fluxes generally occurred at higher soil temperatures (Figure 8). At all sites, this was also related to higher AFPS but, due to the magnitude of the emissions, this effect was less visible for the cultivated site. The highest average emissions over the respective bins of 2°C soil temperature and 5% APFS were observed at the vegetated set-aside site (SA-veg), for soil temperatures around 20°C and AFPS between 65 and 75%.
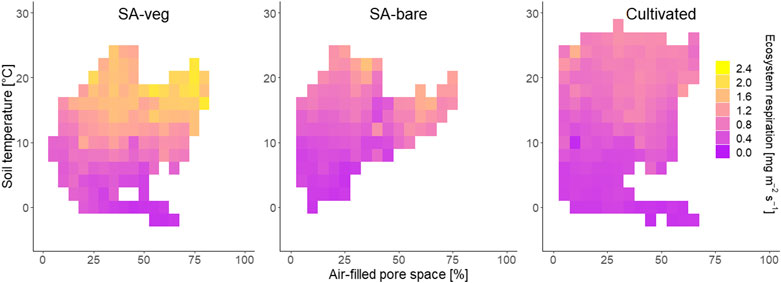
FIGURE 8. Ecosystem respiration [g m−2 h−1] as a function of both soil temperature [°C] and air-filled pore space [AFPS, %]. Individual squares represent increments of 2°C and 5% AFPS, and colors represent the median CO2 fluxes.
Greenhouse Gas Budget
Based on the flux data obtained in this study, we were unable to compare the full GHG budget of the two sites, since our measurements did not include GPP, C losses from water and discharge. In a comprehensive study on an abandoned peat meadow in the The Netherlands, Hendriks et al. (2007) found the area to be a small C sink after rewetting (−86 g CO2-eq. m−2 yr−1). Although we found significant differences for N2O and CH4 between the sites, the fluxes were generally low. Despite higher global warming potential (GWP) compared with CO2, neither N2O nor CH4 is expected to change the picture of the set-aside site as a larger source of respiration than the cultivated site. Based on the results obtained using manual chambers, the contribution of N2O to GWP at the sites (expressed as CO2-equivalents; Table 2) was 0.27% from the bare set-aside soil and 4.33% from the cultivated site, while for CH4 the contribution was negative for all plots (−0.14, −0.18, and −0.10% from SA-bare, SA-veg, and the cultivated site, respectively). However, N2O emissions in particular were influenced by the vegetation cover and could be higher when considering the whole year. Our results therefore support the conclusion reached in previous studies that N2O and CH4 are of minor importance for the GHG balance of cultivated peat soils.
Conclusion
Based on measurements obtained using manual and automatic chambers, ecosystem respiration from drained peat soil was higher at a set-aside site than at a continuously cultivated site. The cultivated site was a greater source of N2O and a smaller sink for CH4 compared with the set-aside site, but this had only a small effect on the GHG balance derived in this study. However, in order to compare the full GHG balance, more continuous measurements are needed and GPP, C losses through water and discharge should be taken into account.
Data Availability Statement
The original contributions presented in the study are included in the article/Supplementary Material, further inquiries can be directed to the corresponding author.
Author Contributions
ÖB planned and set up the experiment and collected the data. KM analysed the data and provided a first draft of the manuscript. ÖB and TK reviewed and edited the manuscript.
Funding
This study was funded by the Swedish Research Council (Formas) under contract no. 214-2010-769 and by the Faculty of Natural Resources and Agricultural Sciences at SLU.
Conflict of Interest
The authors declare that the research was conducted in the absence of any commercial or financial relationships that could be construed as a potential conflict of interest.
Acknowledgments
The authors would like to thank Kerstin Berglund for her fundamental help in setting up the experiment and her helpful comments on the manuscript.
References
Alm, J., Shurpali, N. J., Minkkinen, K., Aro, L., Hytönen, J., Laurila, T., et al. (2007). Emission factors and their uncertainty for the exchange of CO2, CH4 and N2O in Finnish managed peatlands. Boreal Environ. Res. 12, 191–209.
Armentano, T. V. (1980). Drainage of organic soils as a factor in the world carbon cycle. Bioscience 30 (12), 825–830. doi:10.2307/1308375
Armentano, T. V., and Menges, E. S. (1986). Patterns of change in the carbon balance of organic soil-wetlands of the temperate zone. J. Ecol. 74, 755–774. doi:10.2307/2260396
Ballantyne, D. M., Hribljan, J. A., Pypker, T. G., and Chimner, R. A. (2014). Long-term water table manipulations alter peatland gaseous carbon fluxes in Northern Michigan. Wetl. Ecol. Manag. 22, 35–47. doi:10.1007/s11273-013-9320-8
Berglund, K. (1996). Cultivated organic soils in Sweden: properties and amelioration. PhD thesis. Uppsala, Sweden: Sveriges lantbruksuniversitet, 39.
Berglund, Ö., and Berglund, K. (2011). Influence of water table level and soil properties on emissions of greenhouse gases from cultivated peat soil. Soil Biol. Biochem. 43, 923–931. doi:10.1016/j.soilbio.2011.01.002
Berglund, Ö., Berglund, K., and Klemedtsson, L. (2011). Plant-derived CO2flux from cultivated peat soils. Acta Agric. Scand. Sect. B Soil Plant Sci. 61 (6), 508–513. doi:10.1080/09064710.2010.51021
Bolstad, P. V., Davis, K. J., Martin, J., Cook, B. D., and Wang, W. (2004). Component and whole-system respiration fluxes in northern deciduous forests. Tree Physiol. 24, 493–504. doi:10.1093/treephys/24.5.493
Davidson, E. A., Savage, K., Verchot, L. V., and Navarro, R. (2002). Minimizing artifacts and biases in chamber-based measurements of soil respiration. Agric. For. Meteorol. 113, 21–37. doi:10.1016/s0168-1923(02)00100-4
de Mendiburu, F. (2017). Agricolae: statistical procedures for agricultural research. R package version 1.2-8. Available at: https://CRAN.R-project.org/package=agricolae.
Dedysh, S. N. (2002). Methanotrophic bacteria of acidic sphagnum peat bogs. Microbiology 71 (6), 638–650. doi:10.1023/a:1021467520274
Flessa, H., Dörsch, P., and Beese, F. (1995). Seasonal variation of N2O and CH4 fluxes in differently managed arable soils in Southern Germany. J. Geophys. Res. 100, 23115–23124.
Fox, J., and Weisberg, S. (2019). An R companion to applied regression. 3rd Edn. Thousand Oaks, CA: Sage.
Fredriksson, D. (1996). “Peat resources in Sweden,” in Global peat resources. Editor E. Lappalainen (London, United Kingdom: International Peat Society), 137–144.
Frolking, S., Talbot, J., Jones, M. C., Treat, C. C., Kauffman, J. B., Tuittila, E.-S., et al. (2011). Peatlands in the Earth's 21st century climate system. Environ. Rev. 19, 371–396. doi:10.1139/a11-014
Glenn, S., Heyes, A., and Moore, T. (1993). Carbon dioxide and methane fluxes from drained peat soils, southern Quebec. Global Biogeochem. Cycles. 7 (2), 247–257. doi:10.1029/93gb00469
Gorham, E. (1991). Northern peatlands: role in the carbon cycle and probable responses to climatic warming. Ecol. Appl. 1 (2), 182–195. doi:10.2307/1941811
Goulden, M. L., Munger, J. W., Fan, S.-M., Daube, B. C., and Wofsy, S. C. (1996). Measurements of carbon sequestration by long-term eddy covariance: methods and a critical evaluation of accuracy. Global Change Biol. 2, 169–182. doi:10.1111/j.1365-2486.1996.tb00070.x
Granier, A., Ceschia, E., Damesin, C., Dufrêne, E., Epron, D., Gross, P., et al. (2000). The carbon balance of a young Beech forest. Funct. Ecol. 14, 312–325. doi:10.1046/j.1365-2435.2000.00434.x
Grönlund, A., Sveistrup, T. E., Sövik, A. K., Rasse, D. P., and Klöve, B. (2006). Degradation of cultivated peat soils in Northern Norway based on field scale CO2, N2O and CH4 emission measurements. Arch Acker Pfl Boden. 52 (2), 149–159. doi:10.1080/03650340600581968
Hadden, D., and Grelle, A. (2017). The impact of cultivation on CO 2 and CH 4 fluxes over organic soils in Sweden. Agric. For. Meteorol. 243, 1–8. doi:10.1016/j.agrformet.2017.05.002
Heathwaite, A. L., Eggelsman, R., and Göttlich, K. H. (1993). “Ecohydrology, mire drainage and mire conservation,” in Mires: processes, exploitation and conservation. Editor A. L. Heathwaite (Chichester, United Kingdom: John Wiley & Sons), 417–484.
Hendriks, D. M. D., van Huissteden, J., Dolman, A. J., and van der Molen, M. K. (2007). The full greenhouse gas balance of an abandoned peat meadow. Biogeosciences 4, 411–424. doi:10.5194/bg-4-411-2007
Hutchinson, G. L., and Mosier, A. R. (1981). Improved soil cover method for field measurement of nitrous oxide fluxes. Soil Sci. Soc. Am. J. 45, 311–316. doi:10.2135/sssaj1981.03615995004500020017
IPCC (2013). Drained inland organic soils. Available at: https://www.ipcc-nggip.iges.or.jp/public/wetlands/pdf/Wetlands_separate_files/WS_Chp2_Drained_Inland_Organic_Soils.pdf.
Johansson, A. E., Klemedtsson, Å. K., Klemedtsson, L., and Svensson, B. H. (2011). Nitrous oxide exchanges with the atmosphere of a constructed wetland treating wastewater. Tellus B 55 (3), 737–750. doi:10.3402/tellusb.v55i3.16363
Joosten, H., and Clarke, D. (2002). Wise use of mires and peatlands – background and principles including a framework for decision-making. London, United Kingdom: International Mire Conservation Group and International Peat Society.
Joosten, H. (2009). The global peatland CO2 picture: peatland status and drainage related emissions in all countries of the world. Wageningen, The Netherlands: Report for Wetlands International.
Karki, S., Elsgaard, L., Kandel, T. P., and Lærke, P. E. (2015). Full GHG balance of a drained fen peatland cropped to spring barley and reed canary grass using comparative assessment of CO2 fluxes. Environ. Monit. Assess. 187, 62. doi:10.1007/s10661-014-4259-7
Kasimir-Klemedtsson, Å., Klemedtsson, L., Berglund, K., Martikainen, P., Silvola, J., and Oenema, O. (1997). Greenhouse gas emissions from farmed organic soils: a review. Soil Use Manag. 13, 245–250. doi:10.1111/j.1475-2743.1997.tb00595.x
Kasimir-Klemedtsson, Å., Weslien, P., and Klemedtsson, L. (2009). Methane and nitrous oxide fluxes from a farmed Swedish Histosol. Eur. J. Soil Sci. 60, 321–331. doi:10.1111/j.1365-2389.2009.01124.x
Knox, S. H., Sturtevant, C., Matthes, J. H., Koteen, L., Verfaillie, J., and Baldocchi, D. (2015). Agricultural peatland restoration: effects of land-use change on greenhouse gas (CO2 and CH4) fluxes in the Sacramento-San Joaquin Delta. Global Change Biol. 21, 750–765. doi:10.1111/gcb.12745
Koizumi, H., Kontturi, M., Mariko, S., Nakadai, T., Bekku, Y., and Mela, T. (1999). Soil respiration in three soil types in agricultural ecosystems in Finland. Acta Agric. Scand. Sect. B Soil Plant Sci. 49, 65–74. doi:10.1080/09064719950135560
Komsta (2011). Outliers: test for outliers. Available at: http://komsta.net.
Lai, D. Y. F. (2009). Methane dynamics in northern peatlands: a review. Pedosphere 19 (4), 409–421. doi:10.1016/s1002-0160(09)00003-4
Lamb, A., Green, R., Bateman, I., Broadmeadow, M., Bruce, T., Burney, J., et al. 2016). The potential for land sparing to offset greenhouse gas emissions from agriculture. Nat. Clim. Change. 6, 488–492. doi:10.1038/NCLIMATE2910
Law, B. E., Ryan, M. G., and Anthoni, P. M. (1999). Seasonal and annual respiration of a ponderosa pine ecosystem. Global Change Biol. 5, 169–182. doi:10.1046/j.1365-2486.1999.00214.x
Leifeld, J., and Menichetti, L. (2018). The underappreciated potential of peatlands in global climate change mitigation strategies. Nat. Commun. 9 (1), 1071. doi:10.1038/s41467-018-03406-6
Lohila, A., Aurela, M., Regina, K., and Laurila, T. (2003). Soil and total ecosystem respiration in agricultural fields: effect of soil and crop type. Plant Soil. 251, 303–317. doi:10.1023/a:1023004205844
Lohila, A., Aurela, M., Tuovinen, J-P., and Laurila, T. (2004). Annual CO2exchange of a peat field growing spring barley or perennial forage grass. J. Geophys. Res. 109, D18116. doi:10.1029/2004JD004715
Maljanen, M., Hyvtönen, J., Mäkiranta, P., Alm, J., Minkkinen, K., Laine, J., et al. (2007). Greenhouse gas emissions from cultivated and abandoned organic croplands in Finland. Boreal Environ. Res. 12, 133–140.
Maljanen, M., Komulainen, V.-M., Hytönen, J., Martikainen, P. J., and Laine, J. (2004). Carbon dioxide, nitrous oxide and methane dynamics in boreal organic agricultural soils with different soil characteristics. Soil Biol. Biochem. 36, 1801–1808. doi:10.1016/j.soilbio.2004.05.003
Maljanen, M., Liikanen, A., Silvola, J., and Martikainen, P. J. (2003). Methane fluxes on agricultural and forested boreal organic soils. Soil Use Manag. 19, 73–79. doi:10.1079/SUM2002171
Maljanen, M., Sigurdsson, B. D., Guðmundsson, J., Óskarsson, H., Huttunen, J. T., and Martikainen, P. J. (2010). Greenhouse gas balances of managed peatlands in the Nordic countries - present knowledge and gaps. Biogeosciences 7, 2711–2738. doi:10.5194/bg-7-2711-2010
Moore, T. R., and Knowles, R. (1987). Methane and carbon dioxide evolution from subarctic fens. Can. J. Soil Sci. 67, 77–81. doi:10.4141/cjss87-007
Moore, T. R., and Knowles, R. (1989). The influence of water table levels on methane and carbon dioxide emissions from peatland soils. Can. J. Soil Sci. 69, 33–38. doi:10.4141/cjss89-004
Myhre, G., Shindell, D., Bréon, F-M., Collins, W., Fuglestvedt, J., Huang, J., et al. (2013). “Anthropogenic and natural radiative forcing,” in Climate change 2013: the physical science basis. Contribution of working group I to the fifth assessment report of the intergovernmental panel on climate change. Editors T. F. Stocker et al. (Cambridge, MA: Cambridge University Press).
Norberg, L., Berglund, Ö., and Berglund, K. (2016a). Nitrous oxide and methane fluxes during the growing season from cultivated peat soils, peaty marl and gyttja clay under different cropping systems. Acta Agric. Scand. Sect. B Soil Plant Sci. 66 (7), 602–612. doi:10.1080/09064710.2016.1205126
Norberg, L., Berglund, Ö., and Berglund, K. (2016b). Seasonal CO2 emission under different cropping systems on Histosols in southern Sweden. Geoderma Regional. 7, 338–345. doi:10.1016/j.geodrs.2016.06.005
Nykänen, H., Alm, J., Lang, K., Silvola, J., and Martikainen, P. J. (1995). Emissions of CH4, N2O and CO2 from a virgin fen and a fen drained for grassland in Finland. J. Biogeogr. 22, 351–357. doi:10.2307/2845930
Pedersen, A. R. (2017). HMR: flux estimation with static chamber data. R package version 0.4.2. Available at: https://CRAN.R-project.org/package=HMR.
Pedersen, A. R., Petersen, S. O., and Schelde, K. (2010). A comprehensive approach to soil-atmosphere trace-gas flux estimation with static chambers. Eur. J. Soil Sci., 61, 888. doi:10.1111/j.1365-2389.2010.01291.x
R Core Team (2016). R: a language and environment for statistical computing. Vienna, Austria: R Foundation for Statistical Computing.
Regina, K., Pihlatie, M., Esala, M., and Alakukku, L. (2007). Methane fluxes on boreal arable soils. Agric. Ecosyst. Environ. 119, 346–352. doi:10.1016/j.agee.2006.08.002
Regina, K., Sheehy, J., and Myllys, M. (2015). Mitigating greenhouse gas fluxes from cultivated organic soils with raised water table. Mitig. Adapt. Strategies Glob. Change. 20, 1529–1544. doi:10.1007/s11027-014-9559-2
Salm, J.-O., Maddison, M., Tammik, S., Soosaar, K., Truu, J., and Mander, Ü. (2012). Emissions of CO2, CH4 and N2O from undisturbed, drained and mined peatlands in Estonia. Hydrobiologia 692, 41–55. doi:10.1008/s10750-011-0934-7
Scanlon, D., and Moore, T. (2000). Carbon dioxide production from peatland soil profiles: the influence of temperature, oxic/anoxic conditions and substrate. Soil Sci. 165 (2), 153–160. doi:10.1097/00010694-200002000-00006
Schauberger, P., and Walker, A. (2019). openxlsx: Read, Write and Edit xlsx Files. R package version 4.1.4. Available at: https://CRAN-R-project.org/package=openxlsx
Schrier-Uijl, A. P., Kroon, P. S., Hendriks, D. M. D., Hensen, A., Van Huissteden, J., Berendse, F., et al. (2014). Agricultural peatlands: towards a greenhouse gas sink - a synthesis of a Dutch landscape study. Biogeosciences 11, 4559–4576. doi:10.5194/bg-11-4559-2014
Shurpali, N. J., Hyvönen, N. P., Huttunen, J., Clement, R. J., Reichstein, M., Nykänen, H., et al. (2009). Cultivation of a perennial grass for bioenergy on a boreal organic soil - carbon sink or source? GCB Bioenergy 1, 35–50. doi:10.1111/j.1757-1707.2009.01003.x
Silvola, J., Alm, J., Ahlholm, U., Nykänen, H., and Martikainen, P. J. (1996). CO2 fluxes from peat in boreal mires under varying temperature and moisture conditions. J. Ecol. 84, 219–228. doi:10.2307/2261357
Smith, K. A., and Conen, F. 2004). Impacts of land management on fluxes of trace greenhouse gases. Soil Use Manag. 20, 255–263. doi:10.1079/SUM2004238
Teh, Y. A., Silver, W. L., Sonnentag, O., Detto, M., Kelly, M., and Baldocchi, D. D. (2011). Large greenhouse gas emissions from a temperate peatland pasture. Ecosystems 14, 311–325. doi:10.1007/s10021-011-9411-4
van Beek, C. L., Pleijter, M., and Kuikman, P. J. (2011). Nitrous oxide emissions from fertilized and unfertilized grasslands on peat soil. Nutrient Cycl. Agroecosyst 89, 453–461. doi:10.1007/s10705-010-9408-y
van de Riet, B. P., Hefting, M. M., and Verhoeven, J. T. A. (2013). Rewetting drained peat meadows: risks and benefits in terms of nutrient release and greenhouse gas exchange. Water Air Soil Pollut. 1440, 224–133. doi:10.1007/s11270-013-1140-5
von Post, L. (1922). Sveriges Geologiska Undersöknings torvinventering och några av dess hittills vunna resultat. Svenska Mosskulturföreningens tidskrift. 36, 1–27.
Wang, M., Wu, J., Lafleur, P. M., and Luan, J. (2020). Investigation of the climatological impacts of agricultural management and abandonment on a boreal bog in western Newfoundland, Canada. Sci. Total Environ. 711, 134632. doi:10.1016/j.scitotenv.2019.134632
Wang, M., Wu, J., Lafleur, P. M., Luan, J., Chen, H., and Zhu, X. (2018). Can abandoned peatland pasture sequestrate more carbon dioxide from the atmosphere than an adjacent pristine bog in Newfoundland, Canada? Agric. For. Meteorol. 248, 91–108. doi:10.1016/j.agrformet.2017.09.010
Wang, M., Wu, J., Luan, J., Lafleur, P., Chen, H., and Zhu, X. (2017). Near-zero methane emission from an abandoned boreal peatland pasture based on eddy covariance measurements. PloS One 12, e0189692. doi:10.1371/journal.pone.0189692
Wickham, H. (2011). The split-apply-combine strategy for data analysis. J. Stat. Software 40 (1), 1–29. doi:10.18637/jss.v040.i01
Wilke, C. O. (2017). Cowplot: streamlined plot theme and plot annotations for ‘ggplot2’. R package version 0.8.0. Available at: https://CRAN.R-project.org/package=cowplot.
Keywords: drained cultivated peatlands, setting land aside, greenhouse gas, chamber measurements, mitigation action, data assimilation
Citation: Berglund Ö, Kätterer T and Meurer KHE (2021) Emissions of CO2, N2O and CH4 From Cultivated and Set Aside Drained Peatland in Central Sweden. Front. Environ. Sci. 9:630721. doi: 10.3389/fenvs.2021.630721
Received: 19 November 2020; Accepted: 21 January 2021;
Published: 05 March 2021.
Edited by:
Annalea Lohila, University of Helsinki, FinlandReviewed by:
Paavo Ojanen, University of Helsinki, FinlandMartin Maddison, University of Tartu, Estonia
Copyright © 2021 Berglund, Kätterer and Meurer. This is an open-access article distributed under the terms of the Creative Commons Attribution License (CC BY). The use, distribution or reproduction in other forums is permitted, provided the original author(s) and the copyright owner(s) are credited and that the original publication in this journal is cited, in accordance with accepted academic practice. No use, distribution or reproduction is permitted which does not comply with these terms.
*Correspondence: Katharina H. E. Meurer, a2F0aGFyaW5hLm1ldXJlckBzbHUuc2U=