- 1Department of Crop Sciences, University of Illinois at Urbana-Champaign, Champaign, IL, United States
- 2Department of Civil and Environmental Engineering, University of Illinois at Urbana-Champaign, Champaign, IL, United States
- 3Department of Agricultural and Biological Engineering, University of Illinois at Urbana-Champaign, Champaign, IL, United States
Two common tree species, ash (Fraxinus sp.) and oak (Quercus sp.), could provide readily available media for denitrifying bioreactors that use wood-based carbon for biological nitrate treatment. However, it is not known if the wood from Emerald Ash Borer-killed (EAB-killed) ash trees is an effective carbon source for nitrate removal compared to other wood species or if the high-tannin nature of oak inhibits denitrification potential. This lab-scale study showed that EAB-killed ash woodchips did not significantly differ in nitrate removal or denitrification potential compared to a commercially available blend of hardwood chips. However, neither treatment performed as well as oak woodchips in these metrics. Use of high-tannin oak in bioreactors is currently restricted by a federal standard in the United States. Ash woodchips beneficially exhibited the lowest nitrous oxide production potential, and their dissolved phosphorus leaching fell within the range of other woodchip types. Emerald ash borer-killed ash wood could be an effective source for denitrifying bioreactors located within affected regions and oak woodchips merit additional investigation for the application of denitrifying bioreactors.
Introduction
Denitrifying bioreactors using a wood-based carbon source provide practical biological treatment for nitrate (NO3–) in wastewaters and agricultural effluents (Schipper et al., 2010). Such bioreactors consist of a trench (generally on the order of 15–30 m long x 1.8–7.6 m wide x 1 m deep) filled with woodchips through which nitrate-laden waters are routed. The wood provides the electron donor (organic carbon) for the process of denitrification (i.e., NO3– conversion to nitrogen gas) and a colonization surface for the denitrifying microorganisms. One of the most common applications of these woodchip bioreactors is the treatment of subsurface tile drainage water in the US Midwest, a region home to many ash and oak trees. Bioreactor installation costs are generally $7,000–$20,000 with the woodchip costs often accounting for more than 30% of the total (Christianson et al., 2012). Use of commonly available woodchips from widespread tree species could help reduce a barrier to bioreactor implementation, which is important considering the voluntary (i.e., non-regulatory) nature of agricultural water quality improvement in the Midwest.
Several foundational studies in woodchip bioreactor literature compared wood media for suitability (Gibert et al., 2008; Cameron and Schipper, 2010; Healy et al., 2012), with a more recent meta-analysis reporting no difference in nitrogen removal by softwoods vs. hardwoods (Addy et al., 2016). However, the ultimate use of a given wood in this practical on-farm treatment technology will be determined by the local context. For example, Cameron and Schipper (2010) studied eucalyptus which is common in Australasia, but not in the US Midwest.
Bioreactors that are supported with federal incentive payments (i.e., “cost share”) in the US must be designed to the United States Department of Agriculture Natural Resources Conservation Service (USDA NRCS) Conservation Practice Standard for denitrifying bioreactors which restricts the use of woodchips from high-tannin tree species (United States Department of Agriculture Natural Resources Conservation Service [USDA NRCS], 2020). This type of wood, most specifically oak (Quercus sp.), caused concern due to the potential for these tannins to negatively impact the denitrifying community within the bioreactor or the aquatic organisms downstream. Wickramarathne et al. (2020) showed that while oak woodchips do initially leach more tannic acid than other woods in a bioreactor application, the significant differences between wood types were eliminated given a sufficient flushing period. That woodchip flushing study reported that any negative impacts due to the high-tannin nature of the oak would likely be a bioreactor start-up effect or have limited occurrence after periods of woodchip drying/re-wetting.
Like widespread oak trees, ash trees (Fraxinus sp.) have the potential to be a convenient source of woodchips, especially considering the impact of the Emerald Ash Borer (EAB; Agrilus planipennis), an invasive, wood-boring beetle that kills ash trees by eating the tissue under the bark. This invasive pest has become the most destructive and costly forest insect in North America, and such large-scale devastation has generated interest in uses for EAB-killed ash wood, such as woodchips for composite lumber, landscaping, and composting (Poland and McCullough, 2006). The EAB causes physiological differences in ash wood. For example, EAB larvae feed on (that is, extract amino acids from) the cambium directly under the bark which disrupts the transport of nutrients in the tree (Chen et al., 2012). Structurally, EAB-infested ash wood can be more prone to cracking and branch failures at unions compared to non-infested wood (Persad et al., 2013). Finley et al. (2016) successfully used near infrared spectroscopy to detect chemical and physical EAB-induced changes in ash trees compared to non-infected trees as an early detection method to reduce EAB infestations. Collection sites within EAB quarantine areas allow disposal of infected ash trees, and subsequent debarking removes the bark/wood where the EAB resides, reducing risks of further introductions (Poland and McCullough, 2006). If ash woodchips from EAB-killed trees or oak woodchips perform well in denitrifying bioreactors treating NO3– in subsurface drainage water, they could provide a readily available and cost-effective bioreactor media.
The objective of this study was to examine NO3– removal in denitrifying bioreactors and denitrification potential of two common wood types in the US Midwest: ash woodchips from an EAB-killed tree and high-tannin oak woodchips. A secondary objective was to evaluate the potential for nitrous oxide (N2O) production and dissolved reactive phosphorus (DRP) flushing from these woods, to aid in assessing pollution swapping across wood species.
Materials and Methods
Bioreactor Operation and Water Chemistry
Eighteen rectangular Plexiglas® denitrifying bioreactors (15 × 15 × 20 cm) were constructed and operated as previously described by Wickramarathne et al. (2020). The bioreactors were packed with either EAB-killed ash woodchips, oak woodchips (Quercus rubra), or a “mixed hardwood” blend of commercially supplied woodchips. The previous study evaluated tannic acid leaching and leachate true color to assess that aspect of high-tannin oak wood in bioreactors (Wickramarathne et al., 2020). A major procedural difference between the previous study, where only nine bioreactors were evaluated, and the present study was that nine additional bioreactors containing an upstream rock filter were included here. However, there were no statistically significant differences in cumulative NO3-N or DRP removal between reactors with and without rock filters for a given wood type. Thus, the wood-only treatments were grouped with their wood + filter treatment pairs (n = 6 for three treatments).
The oak and ash branches were obtained locally in the fall season (October–November 2017), and the hardwood woodchip blend was purchased from a commercial sawmill (see Wickramarathne et al., 2020). The oak branches were cut from live trees and the ash branches were collected from the ground at the University of Illinois Forestry Plantation in Urbana, Illinois, United States, following an EAB infestation. It was not feasible to have a non-EAB-killed ash treatment as a control for the EAB-killed ash woodchips due to (1) the prevalence of the EAB across the Midwest and (2) the impracticality of harvesting one of the few remaining healthy ash trees for this application. Thus, this testing only allowed evaluation of the combined effect of ash wood plus the EAB-killed nature of that wood.
All three wood types were chipped using a residential chipper (Earthquake® TazzTM 3″ 212cc Gas Woodchipper) and sieved to the 6.3–19 mm particle size range. The branches (oak and ash) were not debarked prior to chipping. Total porosities, median particle diameters (D50), and bulk densities for the three wood types in the 6.3–19 mm particle size range were 61–63%, 15.3–16.8 mm, and 263–300 kg m–3 (Wickramarathne et al., 2020). While this woodchip size was smaller than the 25–51 mm effective diameter required by the NRCS practice standard for woodchips used in full size bioreactors (United States Department of Agriculture Natural Resources Conservation Service [USDA NRCS], 2020), it met recommendations for EAB larval kill. McCullough and Poland (2017) reported there was no EAB survival when woodchippers or grinders were fit with 25 mm screens whereas survival was observed when a larger 100 mm size screen was used. The nutrient content of the wood media was analyzed using a wet digestion method (Brookside Laboratory, New Bremen, Ohio.
Each bioreactor cell continuously received drainage water in up-flow mode to achieve a 7.6 ± 0.9 h Hydraulic Retention Time (HRT) (mean ± stdev flowrate: 0.38 ± 0.03 L h–1), which is a realistic HRT for drainage bioreactors (Woli et al., 2010; Pluer et al., 2019). Inflow water was obtained from an agricultural drainage pond twice weekly, to ensure that its chemical and microbial composition accurately reflected environmental conditions. Potassium nitrate (KNO3) was dosed to a concentration of 30.9 ± 4.8 mg NO3–-N L–1 to simulate subsurface drainage water and to provide a sufficiently high NO3– concentration to avoid NO3– limitation during the experiment to facilitate evaluation of nitrate removal capacity. Bioreactor outflow rates were measured at least once weekly for the duration of the test using a graduated cylinder and a stopwatch.
The cells were operated for 254 days (803–847 cumulative pore volumes of water treated) at 20–22°C. Inflow and outflow samples were collected weekly, filtered within 48 h (0.45 μm filters), and stored frozen until analysis for NO3–-N and DRP within 2 weeks from the collection (Lachat Quickchem, methods 10-107-04-1-A and 10-115-01-1). Because woodchip bioreactors often have a start-up phase that is not representative of longer-term performance (Addy et al., 2016), nitrogen removal was reported beginning at day 157 (after 489–532 cumulative pore volumes). Substantial DRP flushing can occur during that early period, so the full period of operation was considered for P data. Nutrient removal rates (g N or mg DRP removed m–3 d–1) were calculated as the net cumulative mass of a nutrient removed divided by the pore volume of each bioreactor cell (2.54–2.62 L) divided by 98 for NO3– or by 255 days for DRP.
Denitrifying Enzyme Assays
Woodchip samples were collected from the outlet side of each bioreactor at the end of the experiment for use in denitrifying enzyme assays (DEAs) to determine the denitrification potential and N2O production potential of the three wood types (Hathaway et al., 2017, modified from Tiedje et al., 1989). Samples were sealed in plastic bags and stored at −20°C until analysis (approximately 6 months). Woodchips were thawed at 4°C ≈10 d prior to analysis. Individual assays were conducted with 10 g woodchips and 25 mL of nutrient solution (6.7 M glucose-C and 0.29 M NO3–-N) under a helium headspace. Acetylene was added (14% headspace volume) for assays measuring denitrification potential, and helium was added for assays measuring N2O production potential. For each assay, triplicate jars were incubated at 20°C and sampled at 2, 3, 4, and 5 h for N2O concentration measurements (gas chromatography: Shimadzu GC-2014 and AOC 5000 Plus, Kyoto, Japan). Potential denitrification and N2O production rates were determined using N2O concentration change over time, the ideal gas law, and moisture content, considering the effects of dilution and dissolved N2O.
Statistical Analyses
Water quality results were analyzed using a One Way Analysis of Variance (ANOVA) for normally distributed residuals (NO3– removal) and a Kruskal-Wallis One Way Analysis of Variance based on ranks when residuals were not normally distributed (DRP removal; Sigma Plot 14.0, Systat Software). Normality and equal variance assumptions were verified using Shapiro-Wilk and Brown-Forsythe testing, respectively. Differences in denitrification potential between woodchip types were assessed by ANOVA after log transformation to improve normality (R software, version 3.6.1). All testing used α = 0.05.
Results and Discussion
Nitrogen Removal
The oak woodchips exhibited significantly greater nitrogen removal than the ash and mixed hardwood during the steady-state period (d 157–255) with means of 16.0, 13.1, and 14.0 cumulative g NO3–N removed, respectively (Table 1; p < 0.001). The nitrate-removal performance between the three woodchip types had two interesting aspects. First, the NO3– removal with EAB-killed ash woodchips did not provide statistically significantly lower NO3– removal compared to the “control” mixed hardwood blend of woodchips. In other words, while the EAB is known to physically and chemically alter ash wood (e.g., Persad et al., 2013; Finley et al., 2016), any such differences were not enough to significantly reduce the NO3– removal potential of this wood compared to the mixed hardwood control. This indicated that the EAB-killed ash wood could be used as a comparable substitute to mixed hardwood for bioreactor NO3– removal. Nevertheless, it is acknowledged that the inability to have a healthy ash wood treatment was a limitation of this study. The transport and processing of this wood would need to be managed to avoid spreading EAB infestations. However, areas of tile-drained agriculture fall significantly within the federal EAB quarantine area (Figure 1); notable use of EAB-killed ash wood in subsurface drainage bioreactors could feasibly be accomplished without pest transmission. Chipping EAB-infested trees into relatively small woodchips reduces EAB larval survival (e.g., less than 25 mm; McCullough and Poland, 2017) and could be a mitigation measure for pest transmission in this application. However, the use of small woodchips to minimize pest survival should be balanced with chip sizes large enough for sufficient flow properties.
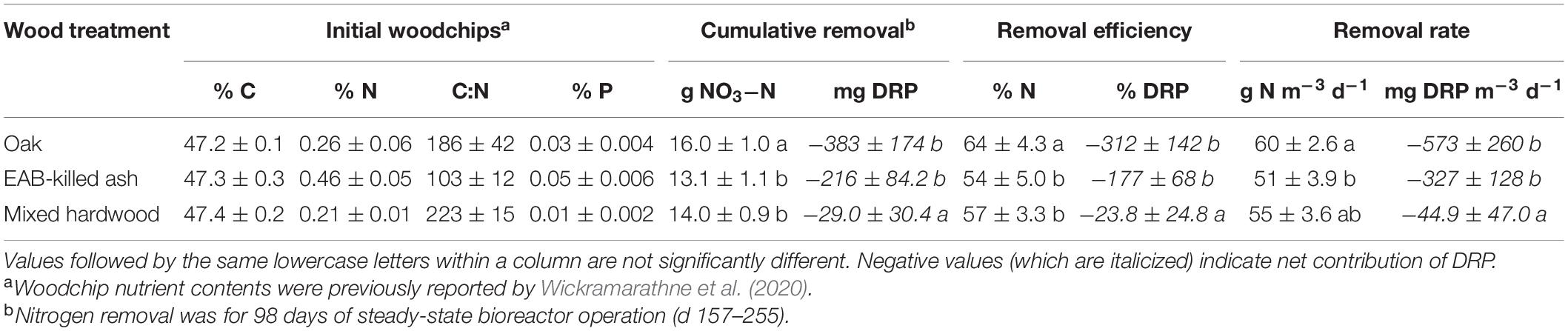
Table 1. Initial woodchip nutrient content (C, carbon; N, nitrogen; P, phosphorus) and nitrate-nitrogen (NO3-N) and dissolved reactive phosphorus (DRP) removal for three wood types (n = 6) in flow-through experiments.
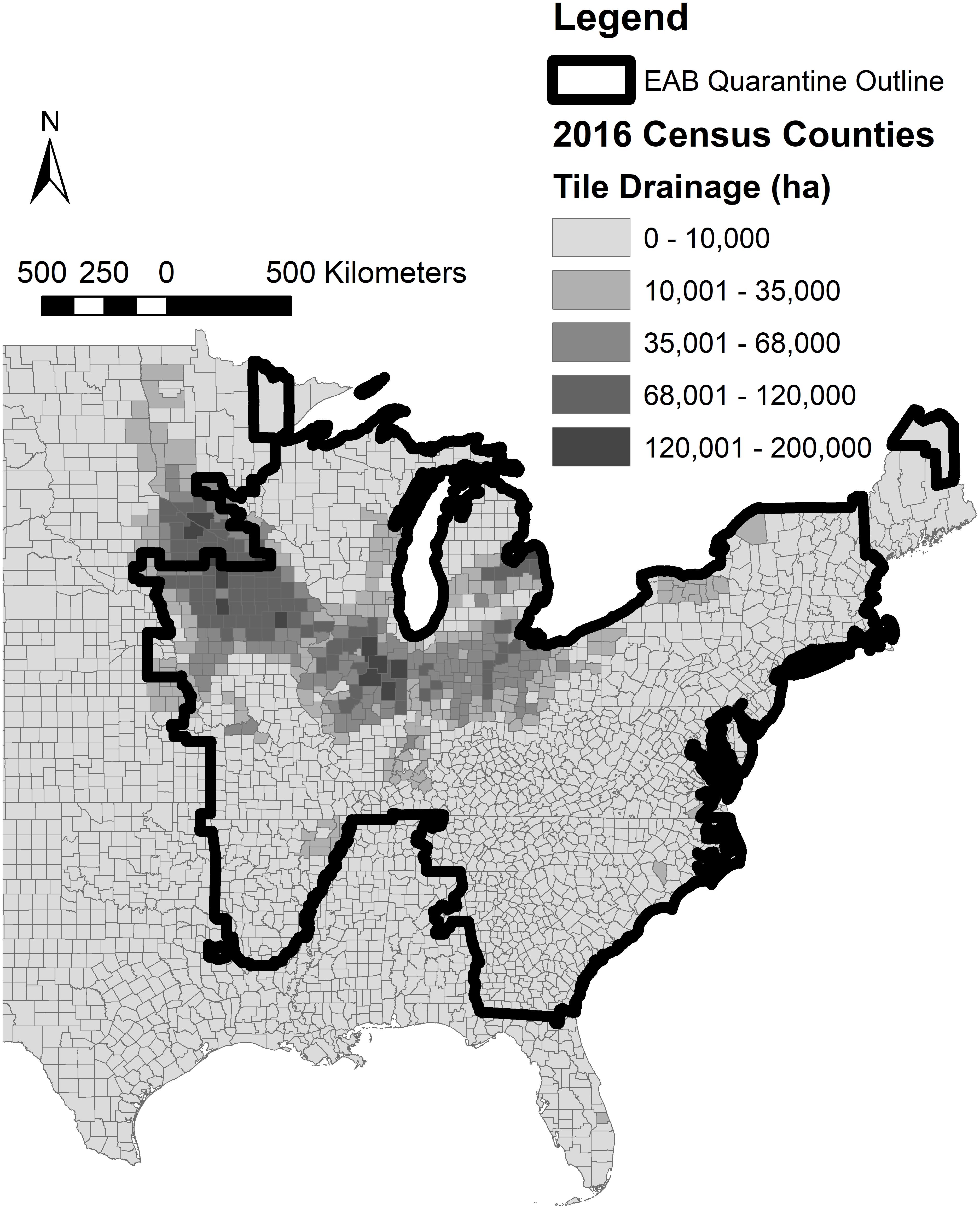
Figure 1. Current Emerald Ash Borer federal quarantine boundary overlaid with the prevalence of subsurface drainage in the United States where denitrifying woodchip bioreactors are needed for biological nitrate treatment (United States Department of Agriculture National Agricultural Statistics Service [USDA NASS], 2017; United States Department of Agriculture Animal and Plant Health Inspection Service [USDA APHIS], 2020).
There are no specific mentions of the use of EAB-killed ash woodchips in published denitrifying bioreactor literature, but personal communications suggest these results are supported by three other studies. A bioreactor column study by Feyereisen et al. (2016) used woodchips from a healthy ash tree, and while NO3– removal was observed, it was at a lower than expected rate (2.2 g m–3 d–1 at 15.5°C). Lepine et al. (2020) reported no notable difference in NO3– removal provided by EAB-killed white ash (Fraxinus americana) vs. Norway maple (Acer platanoides) woodchips also in a column study. Hassanpour et al. (2017) studied bioreactors filled with primarily ash (Fraxinus ornus sp.) woodchips and deemed the technology successful for NO3– treatment in the relatively cold New York climate. Their woodchips were from a lumber mill and it was not known if the trees were EAB-infested, although New York state lies within the federal quarantine boundary (Figure 1). These studies taken together with the controlled experiment performed here indicated EAB-killed ash woodchips appear to be a viable carbon source for bioreactors.
The second notable finding was that oak provided significantly greater NO3– removal compared to the ash and mixed hardwood treatments. This is especially important because the use of this wood is restricted by the NRCS Conservation Practice Standard for denitrifying bioreactors (United States Department of Agriculture Natural Resources Conservation Service [USDA NRCS], 2020) because of its high-tannin content. There are few reports of oak bioreactors, but Jaynes et al. (2008) constructed a denitrifying bioreactor wall in Iowa using “…woodchips obtained from an oak-pallet recycling center,” and Ranaivoson et al. (2019) used a mixture of maple (Acer negundo) and red oak (Quercus rubra) in a bioreactor in Minnesota. Both studies reported favorable NO3– removal (55 and 47%, respectively) and no notable side effects of the oak wood. However, Schrimpelová et al. (2017) did not recommend the use of oak or acacia wood for bioreactors based on a 24 h leaching study. Initial oak leachate is chemically distinct with a darker color and higher tannic acid, chemical oxygen demand, and total nitrogen concentrations than other woods, but these differences can be eliminated with sufficient flushing (Wickramarathne et al., 2020). Oak may provide important NO3– removal (and denitrification potential, see below) benefits despite its high-tannin content.
Denitrifying Enzyme Assays
The oak woodchips exhibited significantly higher denitrification potential (1,685 ± 167 ng N g–1 woodchips h–1; Figure 2; p = 0.049) than the ash and mixed hardwood treatments (1,051 ± 118 and 1,170 ± 260 ng N g–1 woodchips h–1, respectively), while the latter two were not significantly different from each other. All the woodchips were chipped on the same woodchipper and sieved to 6.3–19 mm particle size, but the oak woodchips tended to be slightly smaller within the given range. For example, the median particle diameter for the oak was 15.3 ± 0.17 mm compared to 15.8 ± 0.14 and 16.8 ± 0.19 mm for the ash and mixed hardwood, respectively, after sieving. These particle diameters would be considered “notably similar in practical applications of bioreactors…” (Wickramarathne et al., 2020), but in the small-scale studies performed here, the slightly smaller particle size of the oak woodchips, with its corresponding increase in surface area, may have contributed to the oak’s higher NO3–removal and denitrification potential. Differences in oak wood composition and the associated water chemistry may also have contributed.
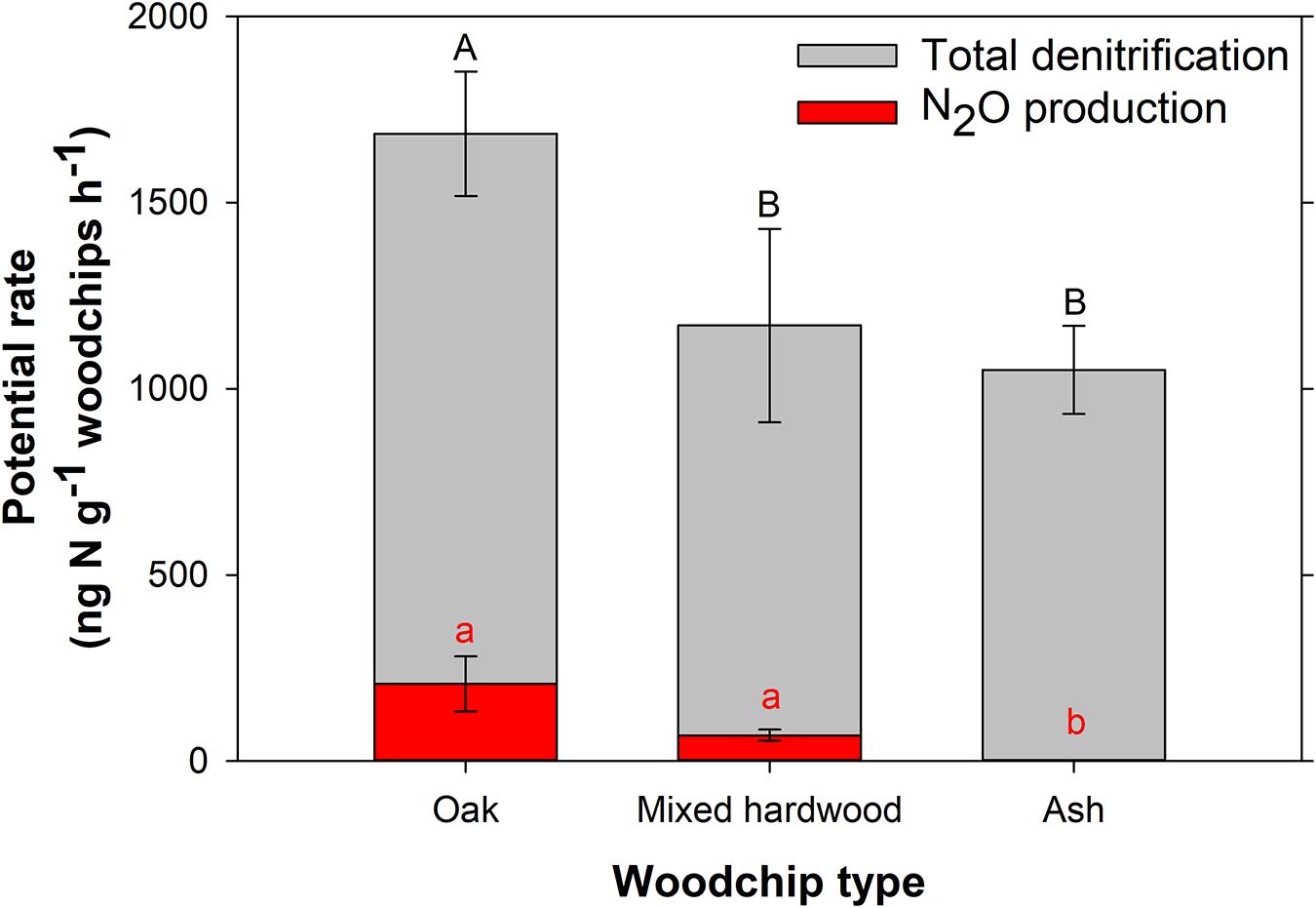
Figure 2. Total denitrification and nitrous oxide (N2O) production potentials for the three woodchip types. Error bars represent the standard deviation of three replicates. Different letters denote a statistically significant difference between potential rates (p < 0.05), with capital letters for total denitrification and lower-case letters for N2O production.
The range of denitrification potentials observed here was similar to the ranges for DEAs reported in two other bioreactor studies. Specifically, Moorman et al. (2010) reported rates of 7–1,358 ng N g–1 woodchips h–1 with the same woodchips studied by Jaynes et al. (2008) mentioned above (“…obtained from an oak-pallet recycling center”). Hathaway et al. (2017) reported rates of 20–4,000 ng N g–1 woodchips h–1 with woodchips of mixed origin. One study reported substantially higher DEA rates of 24,600–38,300 ng N g–1 woodchips h–1 (Warneke et al., 2011), possibly due to differences in reactor influent (treating hydroponic greenhouse water with high NO3–), media (pine woodchips and sawdust), and/or surface area.
Considering N2O production potential, the ash woodchips had no detectable potential for N2O production, while oak and mixed hardwood had significantly higher N2O production potentials of 207 ± 74 and 69 ± 15 ng N g–1 woodchips h–1, respectively (Figure 2; p < 0.001). In soils, lower carbon:nitrogen (C:N) ratios were associated with higher N2O production relative to denitrification, and soils with C:N > 25 resulted in negligible N2O production (e.g., Hunt et al., 2007). Since all of the woodchips used here had C:N ratios much higher than 25 (Table 1), it is not clear what characteristic of the ash resulted in lower N2O production. Overall, the relatively low percentage emitted as N2O indicated woodchips generally have high potential for complete denitrification compared to soil.
Phosphorus Leaching
Another aim of this study was to assess the potential release of DRP. The mixed hardwood treatment resulted in significantly less DRP pollution swapping than the other two treatments (Table 1; p = 0.002). The mixed hardwood also had the lowest P content (Table 1), which may have accounted for this difference in water quality. Woodchip P leaching peaked on approximately day 44 (129–154 cumulative pore volumes), after which either a small amount of removal (oak, hardwood) or approximately net neutral (ash) P dynamics were observed (data not shown). Sharrer et al. (2016) similarly reported pilot-scale woodchip bioreactors treating aquaculture wastewater exhibited phases of both DRP leaching and removal, with removal rates ranging −740– + 230 mg P m–3 d–1, overlapping the range observed here. Based on these results, P leaching from ash woodchips falls within the range of other woodchip types and does not present an additional risk.
Conclusion
The EAB-killed ash wood was not significantly different from the conventional mixed hardwood in NO3– removal or denitrification potential. The ash also beneficially exhibited the lowest N2O production potential. Emerald ash borer-killed ash wood could be a source for denitrifying bioreactors, given precautions are taken to avoid transporting the pest. The use of oak in denitrifying bioreactors merits additional investigation, given that oak woodchips provided the greatest NO3– removal and denitrification potential of the treatments.
Data Availability Statement
The raw data supporting the conclusions of this article will be made available by the authors, without undue reservation, to any qualified researcher.
Author Contributions
NW performed the bioreactor flow-through experiment, provided initial data analysis, wrote the original draft, and assisted with editing. MF performed the DEAs, analyzed and wrote the DEA results, received funding for this work through graduate student grants, and assisted with editing. JZ assisted with methodology, data validation, and editing, and supervised MF. RDC generated the GIS data and map as well as edited the drafts. RAC conceptualized the experiment and provided supervision, data validation, and editing. LC provided funding acquisition, formal data analysis, project administration, supervision (of NW), and led the editing and submission process. All authors contributed to the article and approved the submitted version.
Funding
The authors acknowledge funding from project NR185A12XXXXC004 CESU under the Great Rivers Umbrella Agreement 68-3A75-18-518 504 (USDA NRCS); the Illinois Water Resources Center project #G16AP00051; and the Agriculture and Food Research Initiative program priority area of Renewable Energy, Natural Resources and Environment from the USDA National Institute of Food and Agriculture (grant no. 2018-67011-28071/project accession no. 1015478). Some equipment was supplied via university extension Smith-Lever funds. The funders had no involvement in the study design, data collection or analysis, or manuscript writing.
Conflict of Interest
The authors declare that the research was conducted in the absence of any commercial or financial relationships that could be construed as a potential conflict of interest.
Acknowledgments
We acknowledge Mr. Jay Hayek, University of Illinois Extension Forester, who helped source the wood media.
References
Addy, K., Gold, A. J., Christianson, L. E., David, M. B., Schipper, L. A., and Ratigan, N. A. (2016). Denitrifying bioreactors for nitrate removal: a meta-analysis. J. Environ. Qual. 45, 873–881. doi: 10.2134/jeq2015.07.0399
Cameron, S. G., and Schipper, L. A. (2010). Nitrate removal and hydraulic performance of organic carbon for use in denitrification beds. Ecol. Eng. 36, 1588–1595. doi: 10.1016/j.ecoleng.2010.03.010
Chen, Y., Ulyshen, M. D., and Poland, T. M. (2012). Differential utilization of ash phloem by emerald ash borer larvae: ash species and larval stage effects. Agric. For. Entomol. 14, 324–330. doi: 10.1111/j.1461-9563.2012.00572.x
Christianson, L., Helmers, M., Bhandari, A., Kult, K., Sutphin, T., and Wolf, R. (2012). Performance evaluation of four field-scale agricultural drainage denitrification bioreactors in Iowa. Trans. ASABE 55, 2163–2174. doi: 10.13031/2013.42508
Feyereisen, G. W., Moorman, T. B., Christianson, L. E., Venterea, R. T., Coulter, J. A., and Tschirner, U. W. (2016). Performance of agricultural residue media in laboratory denitrifying bioreactors at low temperatures. J. Environ. Qual. 45, 779–787. doi: 10.2134/jeq2015.07.0407
Finley, K., Chhin, S., Nzokou, P., and O’Brien, J. (2016). Use of near-infrared spectroscopy as an indicator of emerald ash borer infestation in white ash stem tissue. For. Ecol. Manag. 366, 41–52. doi: 10.1016/j.foreco.2016.01.032
Gibert, O., Pomierny, S., Rowe, I., and Kalin, R. M. (2008). Selection of organic substrates as potential reactive materials for use in a denitrification permeable reactive barrier (PRB). Biores. Tech. 99, 7587–7596. doi: 10.1016/j.biortech.2008.02.012
Hassanpour, B., Giri, S., Pluer, W. T., Steenhuis, T. S., and Geohring, L. D. (2017). Seasonal performance of denitrifying bioreactors in the Northeastern United States: field trials. J. Environ. Manag. 202, 242–253. doi: 10.1016/j.jenvman.2017.06.054
Hathaway, S. K., Bartolerio, N. A., Rodríguez, L. F., Kent, A. D., and Zilles, J. L. (2017). Denitrifying bioreactors resist disturbance from fluctuating water levels. Front. Environ. Sci. 5:35. doi: 10.3389/fenvs.2017.00035
Healy, M. G., Ibrahim, T. G., Lanigan, G. J., Serrenho, A. J., and Fenton, O. (2012). Nitrate removal rate, efficiency and pollution swapping potential of different organic carbon media in laboratory denitrification bioreactors. Ecol. Eng. 40, 198–209. doi: 10.1016/j.ecoleng.2011.12.010
Hunt, P. G., Matheny, T. A., and Ro, K. S. (2007). Nitrous oxide accumulation in soils from riparian buffers of a coastal plain watershed–carbon/nitrogen ratio control. J. Environ. Qual. 36:1368. doi: 10.2134/jeq2006.0255
Jaynes, D. B., Kaspar, T. C., Moorman, T. B., and Parkin, T. B. (2008). In situ bioreactors and deep drain-pipe installation to reduce nitrate losses in artificially drained fields. J. Environ. Qual. 37, 429–436. doi: 10.2134/jeq2007.0279
Lepine, C., Christianson, L., Soucek, D., McIsaac, G., and Summerfelt, S. (2020). Solute metal leaching and ecotoxicity of denitrifying woodchip bioreactor outflow - potential reuse of effluent water. Aquac. Eng. 93:102129. doi: 10.1016/j.aquaeng.2020.102129
McCullough, D., and Poland, T. (2017). Evaluation of Chipping and Heat Treatment to Control Emerald Ash Borer. United States Department of Agriculture, Forest Service, Northern Research Station. Available online at: https://www.nrs.fs.fed.us/disturbance/invasive_species/eab/control_management/chipping_heat/ (accessed December 22, 2020).
Moorman, T. B., Parkin, T. B., Kaspar, T. C., and Jaynes, D. B. (2010). Denitrification activity, wood loss, and N2O emissions over 9 years from a wood chip bioreactor. Ecol. Eng. 36, 1567–1574. doi: 10.1016/j.ecoleng.2010.03.012
Persad, A. B., Siefer, J., Montan, R., Kirby, S., Rocha, O. J., Redding, M. E., et al. (2013). Effects of Emerald Ash Borer infestation on the structure and material properties of ash trees. Arboric. Urban For. 39, 11–16.
Pluer, W. T., Morris, C. K., Walter, M. T., and Geohring, L. D. (2019). Denitrifying bioreactor response during storm events. Agric. Water Mgmt. 213, 1109–1115. doi: 10.1016/j.agwat.2018.12.004
Poland, T. M., and McCullough, D. G. (2006). Emerald Ash Borer: invasion of the urban forest and the threat to North America’s ash resource. J. For. 104, 118–124. doi: 10.1093/jof/104.3.118
Ranaivoson, A., Rice, P., Moncrief, J. F., Feyereisen, G. W., and Dittrich, M. (2019). Acetochlor and atrazine dissipation in a woodchip denitrifying bioreactor: a comparison of experimental results with model estimates. Int. J. Hydrol. 3, 286–306.
Schipper, L. A., Robertson, W. D., Gold, A. J., Jaynes, D. B., and Cameron, S. C. (2010). Denitrifying bioreactors–An approach for reducing nitrate loads to receiving waters. Ecol. Eng. 36, 1532–1543. doi: 10.1016/j.ecoleng.2010.04.008
Schrimpelová, K., Maršálková, E., Malá, J., Bílková, Z., and Hrich, K. (2017). “Leachability of denitrifying bioreactor fillings,” in Proceedings of the 17th International Multidisciplinary Scientific GeoConference SGEM, Varna. doi: 10.5593/sgem2017/52
Sharrer, K., Christianson, L. E., Lepine, C., and Summerfelt, S. T. (2016). Modeling and mitigation of denitrification ‘woodchip’ bioreactor phosphorus releases during treatment of aquaculture wastewater. Ecol. Eng. 93, 135–143. doi: 10.1016/j.ecoleng.2016.05.019
Tiedje, J. M., Simkins, S., and Groffman, P. M. (1989). Perspectives on measurement of denitrification in the field including recommended protocols for acetylene based methods. Plant Soil 115, 261–284. doi: 10.1007/BF02202594
United States Department of Agriculture Animal and Plant Health Inspection Service [USDA APHIS] (2020). Areas Under Federal Quarantine in the United States for Emerald Ash Borer. Washington, DC: United States Department of Agriculture Animal and Plant Health Inspection Service.
United States Department of Agriculture National Agricultural Statistics Service [USDA NASS] (2017). Census of Agriculture. Washington, DC: United States Department of Agriculture National Agricultural Statistics Service.
United States Department of Agriculture Natural Resources Conservation Service [USDA NRCS] (2020). Conservation Practice Standard Denitrifying Bioreactor Code 605. Washington, DC: United States Department of Agriculture Natural Resources Conservation Service.
Warneke, S., Schipper, L. A., Bruesewitz, D. A., McDonald, I., and Cameron, S. (2011). Rates, controls and potential adverse effects of nitrate removal in a denitrification bed. Ecol. Eng. 37, 511–522. doi: 10.1016/j.ecoleng.2010.12.006
Wickramarathne, N., Cooke, R. A., Book, R., and Christianson, L. (2020). Denitrifying woodchip bioreactor leachate tannic acid and true color: lab and field studies. Trans. ASABE 63, 1747–1757. doi: 10.13031/trans.14020
Keywords: bioreactor, denitrification, nitrate, oak (Quercus sp.), denitrification potential, ash (Fraxinus sp.)
Citation: Wickramarathne NM, Christianson LE, Foltz ME, Zilles JL, Christianson RD and Cooke RAC (2021) Biological Nitrate Removal With Emerald Ash Borer-Killed Ash and High-Tannin Oak Woodchips. Front. Environ. Sci. 9:648393. doi: 10.3389/fenvs.2021.648393
Received: 31 December 2020; Accepted: 24 February 2021;
Published: 17 March 2021.
Edited by:
Maria Elisa Magri, Federal University of Santa Catarina, BrazilReviewed by:
Zacharias Frontistis, University of Western Macedonia, GreeceIsaac Dennis Amoah, Durban University of Technology, South Africa
Copyright © 2021 Wickramarathne, Christianson, Foltz, Zilles, Christianson and Cooke. This is an open-access article distributed under the terms of the Creative Commons Attribution License (CC BY). The use, distribution or reproduction in other forums is permitted, provided the original author(s) and the copyright owner(s) are credited and that the original publication in this journal is cited, in accordance with accepted academic practice. No use, distribution or reproduction is permitted which does not comply with these terms.
*Correspondence: Laura E. Christianson, TEVDaHJpc0BJbGxpbm9pcy5lZHU=; bGF1cmFlY2hyaXN0aWFuc29uQGdtYWlsLmNvbQ==