Microbial Small RNAs – The Missing Link in the Nitrogen Cycle?
- 1School of Biological Sciences, University of East Anglia, Norwich Research Park, Norwich, United Kingdom
- 2Agrochemistry and Biochemistry Department, Biochemistry and Molecular Biology Division, Faculty of Science, University of Alicante, Alicante, Spain
Non-coding small RNAs (sRNAs) regulate a wide range of physiological processes in microorganisms that allow them to rapidly respond to changes in environmental conditions. sRNAs have predominantly been studied in a few model organisms, however it is becoming increasingly clear that sRNAs play a crucial role in environmentally relevant pathways. Several sRNAs have been shown to control important enzymatic processes within the nitrogen cycle and many more have been identified in model nitrogen cycling organisms that remain to be characterized. Alongside these studies meta-transcriptomic data indicates both known and putative sRNA are expressed in microbial communities and are potentially linked to changes in environmental processes in these habitats. This review describes the current picture of the function of regulatory sRNAs in the nitrogen cycle. Anthropogenic influences have led to a shift in the nitrogen cycle resulting in an increase in microbial emissions of the potent greenhouse gas nitrous oxide (N2O) into the atmosphere. As the genetic, physiological, and environmental factors regulating the microbial processes responsible for the production and consumption of N2O are not fully understood, this represents a critical knowledge gap in the development of future mitigation strategies.
Introduction
Microorganisms are required to sense, respond to and recover from changes in their external environment such as fluctuations in nutrient availability. To thrive under stressful conditions, complex transcriptional regulatory networks fine tune the expression of a variety of genes. Besides transcriptional regulators and the use of alternative sigma factors, gene regulation also involves short regulatory RNAs (sRNAs). These sRNAs are heterogenous in length, sequence composition and secondary structures and modulate a vast range of regulatory circuits required for the cellular response to spatio-temporal changes. The abundance of sequenced bacterial and archaeal genomes alongside the availability of improved sequencing and computational tools has led to a boost in the discovery of sRNAs, making it a fast and exciting area of research. Many of the discovered sRNAs regulate major biological processes such as stress responses by binding to target regions, called seed regions, in the mRNA. This can result in either the activation or the repression of gene expression at the posttranscriptional level (Figure 1) (Wassarman, 2002; Dutta and Srivastava, 2018). sRNAs can originate from within a gene of interest or be processed from the 5′ or 3′ untranslated regions (Bossi and Figuera-Bossi, 2016). Many are then further processed by RNase E to produce sRNA fragments. This can be observed in the processing of RoxS in Bacillus subtilis resulting in an expanded repertoire of target mRNAs (Durand et al., 2015). This review will present the regulatory circuits controlling the nitrogen cycle, discuss the emerging role of sRNAs in these regulatory networks and point towards the potential applications of sRNAs in the field.
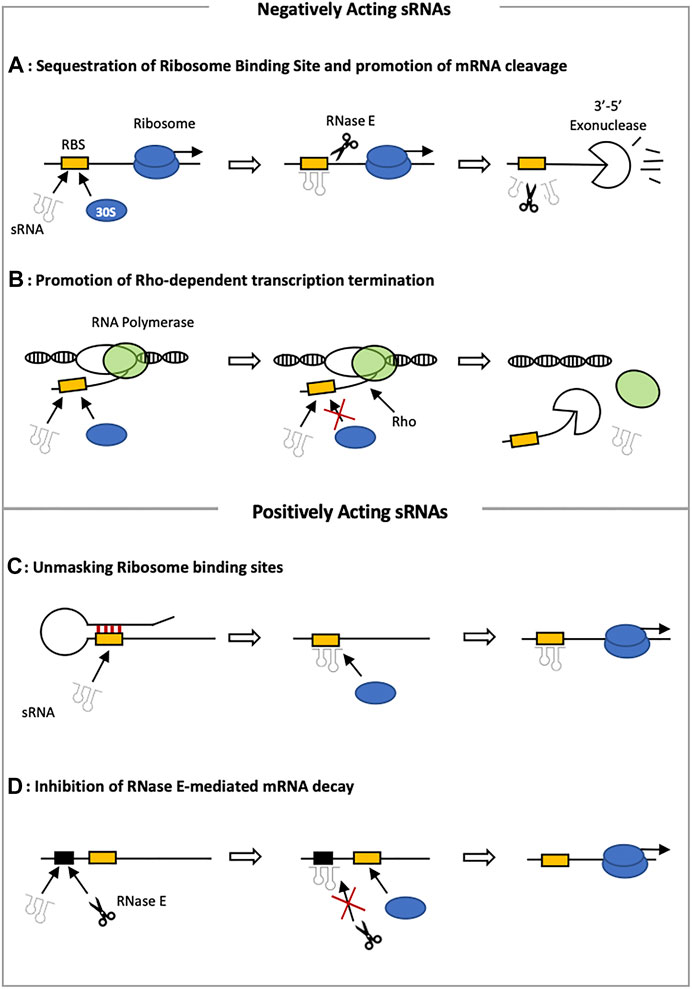
FIGURE 1. Mechanisms of sRNA induced gene repression and activation. (A) When the sRNA target sequence overlaps with the ribosome binding site (RBS) translation initiation is blocked. This leaves the RNA more susceptible to RNase-mediated decay. (B) Alternatively, sRNAs can enhance Rho-binding and subsequently cause premature termination of transcription. (C) Positively acting sRNAs can bind to hairpin-like structures in their target, causing conformational changes to expose a previously inaccessible RBS and stimulate translation initiation. (D) Lastly, sRNAs are able to mask RNase E sites to stabilize their target and activate expression.
Interactions of sRNAs and their targets rely on base-pairings between complementary sequences (Georg et al., 2019). There are two classes of sRNAs–cis-encoded and trans-encoded sRNAs. Cis-encoded sRNAs are transcribed from the DNA strand complementary to the one from which the target mRNA is transcribed resulting in high levels of complementarity. Trans-encoded sRNAs however, are transcribed from regions unrelated to those of their target genes often resulting in reduced complementarity (Gottesman, 2005; Bossi and Figuera-Bossi, 2016; Dutta and Srivastava, 2018). Due to a lower level of complementarity, trans encoded sRNAs can form base pairing with multiple target mRNAs and result in a global regulation of a physiological response. sRNA base-pairing with the target is initiated through fast, high affinity binding of a few exposed nucleotides in the stem loop of the sRNA. This initial interaction promotes pairing of additional nucleotides, which frequently results in alterations to the RNA secondary structure (Otaka et al., 2011; Dutta and Srivastava, 2018). Structure-driven pairing of sRNAs and their targets in which the sRNA recognizes C-rich stretches within accessible loops of the mRNA has also been demonstrated (Storz et al., 2011). Often, trans-encoded sRNAs require the presence of an RNA chaperone to facilitate binding to their target mRNA as their sequences are unrelated (Wagner, 2013). In enteric pathogenic bacteria, such as E. coli and Salmonella, sRNAs have already been particularly well studied and many are associated with pathogenicity (Bossi and Figuera-Bossi, 2016).
The Role of RNA Chaperones in sRNA-mRNA Interactions
The RNA chaperone Hfq is an Sm-like (Lsm) protein in the shape of a homohexameric ring, which can bind both sRNA and mRNA. Lsm proteins play key roles in RNA metabolism in Eukaryotes, Bacteria and Archaea. Hfq was first identified in E. coli, in which it acts as a host factor for the replication of the bacteriophage Qβ (Franze de Fernandez et al., 1968). Binding of Hfq acts to protect free sRNA from degradation by the cellular degradosome and increases local mRNA and sRNA concentrations, but Hfq can also recruit the degradosome to induce accelerated decay of the sRNA-mRNA complex (Georg et al., 2019). Most trans-encoded sRNAs contain a 3′-stem loop, which allows anchoring of the sRNA to Hfq via interactions of poly (U) to the inner rim of the Hfq homohexamer (Otaka et al., 2011). The molecular mechanism of Hfq action has been explained in detail for its role in positive regulation of rpoS mRNA by the sRNA DsrA in E. coli (McCullen et al., 2010). Multilateral interactions between Hfq and the mRNA are formed distorting the mRNA structure to a more compact form, which facilitates binding of the sRNA (McCullen et al., 2010; DeLay and Gottesman, 2011). The binding of the RNA chaperone to a sequence motif in rpoS mRNA results in correct positioning of Hfq and is therefore essential for the pairing of this sRNA to its target mRNA. It is also hypothesized that Hfq increases the local concentration of RNAs, increasing the likelihood of sRNA-mRNA pairing (De Lay et al., 2013).
In addition to Hfq, recent studies have revealed the existence of a second RNA chaperone, ProQ, that can be found additionally to Hfq in Salmonella Typhimurium and E. coli. ProQ has been shown to facilitate binding of sRNAs and their targets, the molecular mechanism for this is however unknown (Smirnov et al., 2016; Smirnov et al., 2017; Westermann et al., 2019). In Salmonella, a loss of this chaperone results in a loss of virulence, as ProQ controls the expression of genes involved in motility, chemotaxis as well as SPI-1 transcripts (Westermann et al., 2019). The FinO domain of ProQ as well as other chromosomally encoded proteins containing a FinO domain are equally grouped as an additional class of bacterial RNA chaperones (Oleiniczak and Storz, 2017).
Interactions between Lsm proteins and sRNAs have also been observed in Archaea. Some, including halophilic archaea, encode a single Lsm protein (Lsm1), while others encode two Lsm proteins (Lsm1 and Lsm2) (Fischer et al., 2010). Lsm1 proteins form heptamers capable of binding DNA. Lsm2 proteins have been shown to associate to hexameric or heptameric complexes in Archaeoglobus fulgidus (Kilic et al., 2006). Crenarchaeota contain a third Lsm3 protein which forms 14-mer complexes. Interestingly the archaeon Methanocaldococcus jannaschii lacks an archaeal Lsm gene and instead contains an Hfq-like protein (Sauter et al., 2003; Nielsen et al., 2007; Vogel and Luisi, 2011). Lsm crystal structures obtained from Archaeoglobus fulgidus and Pyrococcus abyssi show that they are able to bind U-rich RNA in a similar way to Hfq (Töro et al., 2001; Törö et al., 2002; Thore et al., 2003). Binding of Lsm proteins to U-rich stretches was also observed in the crenarchaeum Sulfolobus solfataricus (Märtens et al., 2017), However, despite in vivo confirmation of the interaction of FLAG-tagged archaeal Lsm protein and sRNAs, the physiological functions remain poorly understood (Fischer et al., 2010; Märtens et al., 2015).
Mechanisms of Gene Repression by sRNA
Regulatory sRNAs can directly or indirectly affect the expression of single or multiple genes. In numerous examples, sRNA binding results in blocking of the ribosome binding site (RBS), subsequent inhibition of translation initiation as well as mRNA cleavage via RNAse E and Rho-dependent transcription termination (Storz et al., 2004; Figures 1A,B). Binding of an sRNA within the physical boundary of the RBS of the target mRNA prevents entry of the 30S ribosomal subunit and therefore blocks translation initiation (Figure 1A) (Udekwu et al., 2005; Morita et al., 2006; Bouvier et al., 2008). Many sRNAs repress their targets by masking the Shine Dalgarno (SD) sequence or the AUG start codon. This mechanism is utilized by the sRNA RhyB found in E. coli. RhyB downregulates Fe-storage and non-essential Fe-binding proteins when iron availability is limited (Masse and Gottesman, 2002; Dutta and Srivastava, 2018). Absence of iron increases RhyB expression, which interferes with the binding of the 30S subunit to the RBS of the target mRNAs. An interaction of RhyB with Hfq can also result in the repression of the enzyme methionine sulfoxide reductase by binding to two sites on msrB mRNA. Binding to the first site stops ribosome entry at the RBS whereas binding to the second site results in a recruitment of RNase E (Bos et al., 2013; Dutta and Srivastava, 2018). Other sRNAs, such as OxyS, however can bind as far downstream as the 5th codon, without any interaction with the SD or the start codon (Bouvier et al., 2008). When ribosome entry sites are blocked, it is possible for the 30S subunit to bind to ‘Standby regions’, which are located 100 nucleotides upstream of the translation initiation site (Darfeuille et al., 2007). This mechanism is followed by the cis-acting sRNA Isr-1 in E. coli and does not require the presence of an Hfq chaperone (Darfeuille et al., 2007).
In addition to the blocking of ribosome entry sites, base pairing of an sRNA and its target at either the 5′UTR region or at downstream coding sequences can also lead to recruitment of ribonucleases such as RNase E. In prokaryotes, RNase E is a crucial ribonuclease responsible for the turnover of sRNAs and mRNAs (Chao et al., 2017). In some cases, Hfq can act as a protective layer against RNase E degradation by stabilizing the sRNA and promoting base-pairing with the target. It has also been shown that Hfq has the capacity to directly bind to an unstructured C-terminal domain within RNase E forming a ribonucleoprotein with the sRNA that induces mRNA decay (Morita et al., 2005). The involvement of RNase E in sRNA induced gene repression has been confirmed for a large number of sRNAs such as RhyB and SgrS in E. coli.
Attenuation of transcription is a final mechanism of sRNA-induced gene repression. An example of this is the repression of the virulence gene icsA by the sRNA, RnaG, in Shigella flexneri (Giangrossi et al., 2010). The promoter of both the RnaG sRNA and the icsA virulence gene are convergent and lie less than 120bp apart. Hetero-duplex formation of the sRNA and its target gene results in a conformational change generating an intrinsic terminator that blocks the movement of RNA polymerase and thus attenuating icsA transcription.
Mechanisms of sRNA-Induced Gene Activation
sRNAs are also able to mediate activation of genes involved in a wide array of physiological processes (Frohlich and Vogel, 2009; Dutta and Srivastava, 2018). One mechanism of gene activation is the stabilization of target mRNAs by protecting them from degradation by cellular RNases (Figure 1D). This has been observed for the glucose phosphate stress induced sRNA SgrS found in E. coli and Salmonella (Vanderpool and Gottesman, 2007). Binding of SgrS to its target mRNA pldB-yigL masks an RNase E site within the pldB open reading frame and facilitates production of the YigL phosphatase (Papenfort et al., 2013). Often, the secondary structure of mRNAs sequesters the ribosome binding site, which can be liberated for protein synthesis through pairing with an sRNA (Figure 1C). This process is also referred to as ‘anti-antisense’ mechanism. This activation of the 5′ UTR was first discovered for the sRNA, RNAIII, in Staphylococcus aureus (Morfeldt et al., 1995). RNAIII is regulated by cell density through quorum sensing and activates the hla gene, which encodes an α-Toxin (Novick and Geisinger, 2008; Papenfort and Vanderpool, 2015). Activation is achieved through an interaction of the 5′-end of the sRNA and the SD-sequence of the target, preventing the formation of a translation-inhibitory structure formation. This ‘anti-antisense’ mechanism can also be observed in the activation of the sigma factor σS in E. coli. As the 5′ UTR of the σS-mRNA (rpoS) is unusually long, it forms a complex hairpin structure, making it inaccessible for ribosomal entry (Battesti et al., 2011). Several sRNAs (DsrA, RprA, ArcZ) are able to bind to specific sections within the 5′ UTR to rearrange the structure and enhancing the rate of σS translation (Bossi and Figuera-Bossi, 2016).
In addition to the anti-antisense mechanism observed in rpoS activation, a unique transcription anti-termination system has been discovered to play a crucial role in inhibiting Rho-dependent transcription termination in the 5′ UTR of rpoS (Sedlyarova et al., 2016). Rho is a hexameric helicase protein and together with its cofactor NusG it acts as a global transcription termination factor in prokaryotes (Boudvillain et al., 2013). Rho binds to a stretch of C-rich unstructured RNA that is around 80 nucleotides in length and is located near the transcription terminator. After mRNA binding, Rho atpase activity is stimulated. Under specific circumstances, Rho also appears to be active in the 5′ UTR, which induces premature termination of transcription. Within rpoS, one of these Rho loading sites can be found in the leader sequence. Binding of an sRNA close to this Rho-loading site blocks binding of Rho and enhances transcription and protects from cleavage induced by RNase E (Figure 1D) (McCullen et al., 2010). Hfq further increases the stability of the sRNA-rpoS interaction.
In some cases, sRNA can positively regulate expression of an open reading frame (ORF) through interactions with its 5′ UTR that can result in a subsequent upregulation of a different cistron of the mRNA which is translationally coupled to the ORF (Dutta and Srivastava, 2018). In Pseudomonas aruginosa, the oxygen-responsive sRNA PhrS activates the ufo-pqsR operon in the absence of oxygen (Sonnleitner et al., 2011). The transcriptional regulator PqsR controls the expression of several virulence genes in P. aeruginosa including the toxic pigment pyocyanin (PYO) and the quorum sensing and biofilm formation signal PQS. PhrS binds to the 5′ region of ufo which results in conformational change liberating the RBS. As ufo is translationally coupled to pqsR, the presence of sRNA PhrS eventually results in enhanced translation of PqsR and increased levels of PYO and PQS (Sonnleitner et al., 2011).
sRNA Induced Protein Sequestration
Certain sRNAs have the ability to directly sequester RNA-binding proteins inhibiting them from carrying out their functions or bind enzymatic proteins to inhibit or modify their enzymatic activity. Therefore, these sRNAs can indirectly regulate the expression of many genes related to this protein. The RNA-binding protein CsrA is a post-transcriptional regulator that has multiple targets, which include several genes involved in carbon flux pathways (Babitzke and Romeo, 2007). The presence of sRNAs such as CsrB results in an inactivation of CsrA activity as CsrB acts as a direct competitor for the CsrA target mRNAs in the cell removing its function and changing the expression level of a large number of genes. Inhibition of a protein’s enzymatic activity can be observed for sRNA 6S which binds to RNA polymerase in bacteria interfering with σ70-induced transcription (Wassarman and Storz, 2000). Production of 6S is maximized during stationary phase and as a result the expression of several genes is reprogrammed to allow the cell to adapt to the given environmental conditions. The RNase BN/Z facilitates 6S RNA decay (Chen et al., 2016).
The Role of sRNAs in Physiological Responses
As sRNAs are significantly smaller than mRNAs and do not require translation into a protein, they have a potential energetic advantage over the production of protein transcription factors (Beisel and Storz, 2010). sRNA copy number within the cell can also be very high while their turnover time is short, resulting in a sharp deterioration of sRNA numbers once they have exerted their rapid and effective function in response to an environmental signal. This suggests that sRNA could be crucial in the rapid adaptation to dramatic shocks such as sudden nutrient change that challenge the survival of the microorganism. As more advances are made in sRNA research, the more mechanisms are discovered that demonstrate the diverse mechanisms of action of sRNAs and their association with a large variety of physiological processes.
It is becoming more and more clear that cell communication during quorum sensing and biofilm formation is regulated by sRNAs. To react to changes in cell density V. cholerae possesses two-component membrane-bound sensor kinases. At low cell density, the response regulator LuxO is phosphorylated and activates the expression of five sRNAs that regulate the expression of genes involved in virulence and biofilm formation (Bardill et al., 2011; Michaux et al., 2014). In pathogenesis, sRNAs often modulate expression levels of outer membrane proteins which are targets for the immune system, as well as other responses required for the survival within the host. Members of the CsrB family of sRNAs in Salmonella, Yersinia, Vibrio and other pathogenic bacteria have already proven to alter infection by antagonizing global regulators of virulence genes (Waters and Storz, 2009). Other sRNAs are involved in the adaptation to nutrient availability. Switches between nutrient availability and starvation trigger major changes in gene expression and require a coordination of regulatory networks. In E. coli, the sRNA SgrR modulates the response to an accumulation of glucose 6-phosphate which is toxic when present at high concentrations (Vanderpool and Gottesman, 2004). Besides biofilm formation and pathogenesis, many of the known sRNAs are involved in stress responses such as oxidative stress, osmotic stress and the switch between aerobic and anaerobic metabolism.
The Global Nitrogen Cycle
Although the focus of sRNA studies has predominantly been on key model bacteria, with a particular focus on stress responses and pathogenesis, it is becoming clear that sRNAs also play a crucial role in environmentally relevant pathways. Biogeochemical cycles are critical to all forms of life on earth. They describe the dynamic transformation of energy and matter from different reservoirs including the atmosphere, the oceans, the terrestrial biosphere and the geosphere into usable forms that support the optimal function of all ecosystems. The major biotic drivers of these transformations are plants and microorganisms. Most of the naturally occurring organic compounds required for the existence of life contain the life sustaining elements carbon (C), hydrogen (H) and one or more of the elements: nitrogen (N), oxygen (O), phosphorus (P) and sulfur (S) (Brusseau, 2019). Cycling of these key elements through the different reservoirs is interconnected via anabolic and catabolic processes including photosynthesis, assimilation, respiration and decomposition (Brusseau, 2019). An example of this strong interconnection of the cycles is the use of reduced carbon forms in anoxic habitats which can be oxidized, creating an electron flow eventually utilized by microbes in respiration to reduce, for example, nitrate to atmospheric N2 (denitrification), to reduce sulfate to sulfite (sulfate reduction) or to reduce carbon dioxide (CO2) to methane (methanogenesis) (Madsen, 2011). Mineral metal oxide respiration such as Fe(III) and Mn(IV) are major drivers in organic carbon oxidation and therefore also influence nitrogen and sulfur cycles (Richardson et al., 2012). Perturbations to a single biogeochemical cycle can therefore have detrimental effects on all other cycles leading to changes in the health and function of ecosystems. Anthropogenic influences such as the combustion of fossil fuels and the use of synthetic fertilisers have already caused significant imbalance to the global P and N cycles. An understanding of the structure, function and regulation of the biogeochemical processes in a changing climate is crucial to determine future mitigation strategies.
The nitrogen cycle has already experienced a global scale perturbation. Nitrogen gas (N2) constitutes 78% of the Earth’s atmosphere. However, the gaseous form of nitrogen cannot be accessed by the majority of living organisms. Atmospheric N2 is only accessible to microorganisms, the N2-fixing Bacteria and Archaea, which are estimated to biologically fix approximately 0.1% of the N2 pool (Vitousek and Howarth, 1991). Once fixed by these microorganisms, nitrogen becomes available to plants and animals. With the discovery of the Haber-Bosch process at the beginning of the 20th century it became possible to industrially fix the atmospheric nitrogen converting N2 into reactive N-forms (Chen et al., 2019). Most of the industrially fixed nitrogen is utilized to produce nitrogen rich fertilizers used in agriculture to feed the ever-growing world population. It was estimated that in 2002, over half of the world’s population consumed food produced with N fixed via the Haber-Bosch process (Smil, 2002). Despite their importance, the application of these fertilizers can cause huge environmental concerns and major changes to the balance of the biogeochemical nitrogen cycle (Richardson et al., 2009). Large quantities of reactive nitrogen from fertilisers are lost to the environment due to runoff, or as gaseous products, which can cause soil acidification as well as increased emissions of the greenhouse gas nitrous oxide (N2O). N2O has a global warming potential almost 300 times higher than CO2 (Galloway and Cowling, 2002). Since the beginning of industrialization, the atmospheric loading of N2O has increased by over 20% and subsequently it has been listed as one of six gases subjected to restriction in the Kyoto protocol (Richardson et al., 2009; Smith et al., 2012; Prinn et al., 2018). The Intergovernmental Panel on Climate Change (IPCC) estimates that one third of the total global N2O emissions are a result of anthropogenic activities, with agriculture accounting for the largest fraction (Stocker et al., 2013). The economic costs as a result of nitrogen pollution across Europe are estimated to range from 70 to 320 billion euros a year, mainly due to reduced air and water quality (Sutton et al., 2011).
The nitrification pathway is responsible for the conversion of ammonium to nitrite. Nitrates and nitrites are a natural component of plant material, however an increase in nitrite from fertilisers can lead to accumulation of nitrate in vegetable tissue (Renseigne et al., 2007). High levels of nitrate in food are responsible for methemoglobinemia (blue baby syndrome) in young children (Chan, 2011). Additionally, increased conversion of ammonium can lead to a loss of soil nitrogen through leaching which results in a wastage of fertiliser and water pollution through eutrophication of rivers and lakes (Robertson and Vitousek, 2009; Sutton et al., 2011). In freshwater ecosystems, the levels of nitrite are continuously increasing due to industrial effluents from industries producing metals, dyes, sewage aquaculture and runoff from agricultural soils supplemented with nitrogen fertilisers (Van Maanen et al., 1996; Jensen 2003). As nitrite is rapidly oxidized to nitrate (NO3−), NO3− is often the predominant N-form found in ground- and surface waters. Elevated levels of nitrite in both sea and fresh-water environments have detrimental effects on aquatic animals by interfering with multiple physiological functions such as ion regulation, respiration and the cardiovascular system (Jensen et al., 2003). Biological removal of both nitrate and nitrite from aquatic environments can be achieved aerobically through the processes of nitrate or nitrite assimilation or anaerobically via denitrification. Microorganisms and plants are responsible for the transformation of more than 104 megatons of inorganic nitrogen per year via the process of assimilatory nitrate reduction (Guerrero et al., 1981).
The production of N2O in the soil is primarily attributed to the microbial processes of nitrification and denitrification, although under certain environmental conditions such as nitrate-sufficiency and nitrite accumulation, dissimilatory nitrate and nitrite reduction to ammonium may well significantly contribute to N2O production (Rowley et al., 2012; Stremińska et al., 2012). However, the denitrification process is the only known biological process physiologically capable of the consumption of this greenhouse gas (Bernhard, 2010), putting aside the non-physiological reduction of N2O by nitrogenase (Hoch et al., 1960). Complete denitrification is a sequential four-step reduction of soluble nitrogen oxides nitrate and nitrite to the gaseous nitrogen oxides nitric oxide, nitrous oxide and dinitrogen, which takes place in the absence of oxygen (Zumft and Kroneck, 2007). The enzymes catalyzing these reactions are nitrate reductase, nitrite reductase, nitric oxide reductase and the periplasmic nitrous oxide reductase respectively. As the denitrification process is a modular pathway, some organisms are capable of completely reducing NO3− to nitrogen gas while others may lack one or several of the enzymes required for the other steps involved in the reduction cascade (Philippot et al., 2002). The nitrous oxide reductase (NosZ) protein phylogeny has two distinct groups – clade I, and the recently discovered clade II (Jones et al., 2013; Hallin et al., 2018)). The two clades differ not only in protein phylogeny but also in the nos gene cluster organization, the NosZ translocation pathway as well as the frequency of co-occurrence with other denitrification genes. Clade I organisms are complete denitrifiers which also possess nirS or nirK genes encoding for nitrite reductase (Jones et al., 2013; Conthe et al., 2018). The majority of the clade II organisms lack complete denitrification machinery and appear to be non-denitifying N2O reducers capable of consuming N2O without contributing to its production, making these organisms of significant interest as they may be potential N2O sinks in the environment. Despite the pressing need to develop mitigation strategies to combat the ever-increasing N2O emissions, we still do not fully understand the regulatory network underlying the microbial reaction pathways responsible for the production and consumption of this greenhouse gas. An enhanced understanding of the ecology of the nosZ clade II organisms as well as the conditions under which their activity is favored is needed (Domeignoz-Horta et al., 2016). This includes an increased knowledge regarding sRNA content and activity in these organisms.
An Overview of Transcriptional Regulation of the Nitrogen Cycle
Bacteria and Archaea have developed a range of strategies allowing the uptake and utilization of various nitrogen sources from their environment. These processes are tightly regulated in response to environmental conditions to ensure the correct temporo-spatial control of the pathways and minimize any inappropriate energetic costs as well as maximize the competitive growth advantage. Biological nitrogen fixation is a crucial process providing an input of fixed nitrogen into soils and therefore directly affecting natural ecosystem productivity (Dixon and Kahn, 2004). Nitrogen fixing Bacteria and Archaea are found in a wide variety of habitats including soil and marine environments. The enzyme required for the fixation of nitrogen, nitrogenase (nif), is ATP-dependent as well as highly oxygen sensitive and is therefore tightly regulated in response to fixed nitrogen, carbon, energy and extracellular oxygen levels (Dixon and Kahn, 2004). Thus, in the model K. pneumoniae the transcription of the nif genes is under the regulation of the global two-component system ntrBC (Dixon et al., 1986). In the absence of nitrogen NtrB phosphorylates NtrC which subsequently activates the transcription of nifA which then modulates the expression of numerous other nif genes. The nifA gene is co-transcribed with nifL which encodes a nitrogen-responsive flavoprotein acting as a negative regulator of NifA. This co-transcription adds an additional level of nitrogenase regulation in response to changing oxygen levels and nitrogen fixation (Halbleib and Ludden, 2000). Variations of this core regulatory mechanism are found in many nitrogen fixing microorganisms. In response to changing levels of oxygen in diazotrophic Proteobacteria, the transcription of nif genes depends on the alternative sigma factor σ54 whose activation requires NifA, an enhancer binding protein. NifA transcription is directly oxygen-responsive in these bacteria. In other diazotrophs, NifA is not directly regulated by oxygen levels and instead its activity is regulated by the flavoprotein NifL (Martinez-Argudo et al., 2004). Another protein shown to be involved in the regulation of nif genes is the histidine kinase RegB which can respond to the cellular redox status through an active cysteine. RegB is found in Rhodobacter capsulatus in which it binds to the nifA2 promoter providing an additional layer of redox control (Elsen et al., 2004) Little is known about regulation of nitrogen fixation in archaea. The best studied examples of regulation in nitrogen fixing archaea are the methanogenic models Methanococcus maripaludis and Methanosarzina mazei. Under nitrogen sufficient conditions in these methanoarchaea, the global regulator NrpR regulates target gene expression by binding to the corresponding operator and blocking the recruitment of the RNA polymerase. Depletion of extracellular nitrogen levels result in an increased production of intracellular 2-oxoglutarate which binds to NrpR causing it to release its target promoters. Homologues of this regulator have been found across many other archaeal species (Dixon and Kahn, 2004).
The bacterial assimilation of nitrogen sources such as ammonia or amino acids is also under tight control by several transcriptional regulators (Prasse and Schmitz, 2018). Nitrate assimilation is widespread across proteobacteria and is often controlled at a transcriptional level by extracellular concentrations of nitrate and nitrite as well as ammonium (Luque-Almagro et al., 2017). In the presence of ammonium in cyanobacteria, the transcriptional regulator NtcA represses the nitrate reduction machinery. Once this nitrogen source is depleted the nitrate reductase genes are activated. In proteobacteria, the two-component system NtrB/NtrC regulates the activation of σ54 promoters and therefore controls central nitrogen metabolism (Herrero et al., 2001; Muro-Pastor et al., 2005). The sensor histidine kinase NtrB senses low nitrogen conditions and subsequently autophosphorylates and transfers a phosphoryl group to the NtrC response regulator protein. NtrC then acts as a transcriptional activator (Sanders et al., 1992). Another two-component system with a proposed role in nitrogen assimilation and similarities to NtrBC is the NtrYX system found in diazotrophs. In Azorhizobium caulinodans it has been suggested to interact with the NtrBC system to respond to changing levels of nitrate (Pawlowsky et al., 1991). In the model denitrifier Paracoccus denitrificans, NtrBC is mainly required for nitrate regulation and NtrYX only has a minor contribution. It has been proposed that nitrate assimilation is regulated at three levels in P. denitrificans (Luque-Almagro et al., 2017). Firstly, NtrC activates the expression of the assimilatory nitrate reductase (Nas) in the absence of ammonium. Level two consists of regulation via the NasTS system, which controls expression of the nas operon by transcription antitermination in response to nitrate levels. In the absence of nitrate, the positively acting RNA-binding transcription antitermination regulator NasT is held inactive in inhibitory complex with the nitrate sensor NasS. When NasS binds nitrate, dissociation of the NasTS complex is triggered which leads to increased levels of free and active NasT. NasT then may activate expression at the level of mRNA synthesis and translation. This novel regulatory mechanism involving NasTS is similar to that present in Klebsiella oxytoca mediated by NasR and could be of importance in a wide number of other bacteria (Chai and Stewart, 1999; Luque-Almagro et al., 2011; Luque-Almagro et al., 2017). The crystal structure of both NasT and NasR has shown that both possess an ANTAR domain crucial for specific binding to a leader mRNA (Boudes et al., 2012). Recent work on the soybean endosymbiont Bradyrhizobium japonicum has provided evidence of a cross talk between N-assimilation and N-respiration at an RNA-level (Sánchez et al., 2014). The study suggested that the nasTS genes regulate respiratory nitrous oxide and periplasmic nitrate reductases. In cyanobacteria, regulation of N-assimilation is taken over by NtcA which forms dimers capable of binding promoters in response to the given levels of nitrogen available. As ammonium can be directly incorporated into glutamate it is often the preferred source of nitrogen for many organisms. However, the enzyme responsible for this conversion, glutamate dehydrogenase, has a low ammonium binding affinity. Therefore, a combination of the high ammonium-affinity enzyme glutamine synthetase and a glutamine oxoglutarate aminotransferase are responsible for the assimilation of ammonium under nitrogen limitation. This regulation is sensed and regulated by PII-like sensor proteins.
Little is known about the transcriptional regulation of nitrification. Nitrification is carried out in two steps: the conversion of ammonia to nitrite carried out by ammonia oxidisers such as Nitrosomonas and Nitrosospira and secondly the conversion of nitrite to nitrate which is carried out by nitrite oxidisers such as Nitrobacter and Nitrospira (Prosser, 2007; Norton and Stark, 2011). The rate of nitrification largely depends on environmental conditions such as temperature, soil moisture and pH (Norton and Stark, 2011). Heavy metals such as Ni (II), Zn (II), Cd (II) and Pb (II) have been shown to strongly impact the levels of nitrification. In Nitrosomonas europaea, exposure to Cd (II) caused a significant decrease in the production of ammonia monooxygenase (amoA), while exposure to Zn (II) resulted in an upregulation of amoA (Kapoor et al., 2015). Transcription levels of amoA have also been investigated in response to changes in water availability. Wetting of dry soil to model rainfall after a period of drought resulted in a rapid increase in amoA transcripts demonstrating a tight coupling of transcription levels of nitrification genes to the soil environment (Placella and Firestone, 2013; Norton and Ouyang, 2019).
In comparison to nitrification, transcriptional regulation in denitrification has been extensively studied, particularly in recent years (Gaimster et al., 2018). The expression of the denitrification enzymes Nar, Nir, Nor and Nos in P. denitrificans is regulated by environmental signals including availability of oxygen, nitrate, nitrite, nitric oxide and copper (Figure 2) (Gaimster et al., 2018).
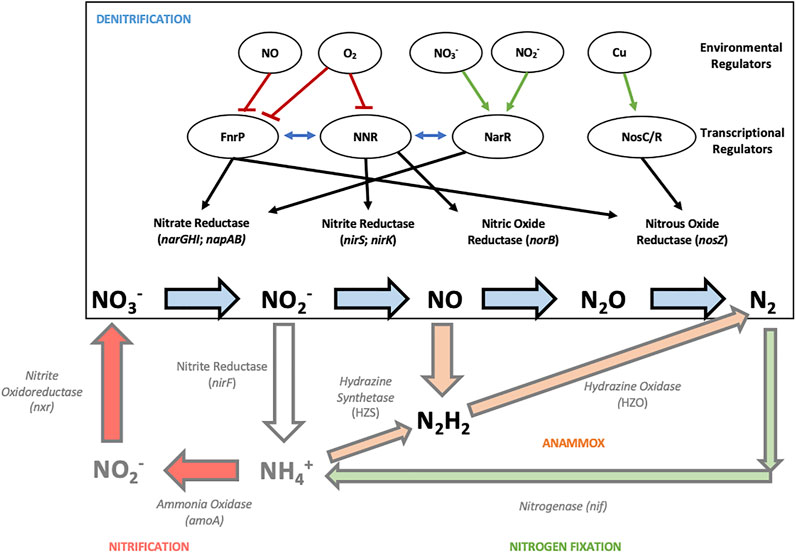
FIGURE 2. An overview of the known transcriptional and environmental regulators of the denitrification pathway in the model denitrifying bacterium Paracoccus denitrificans. The black and red arrows between the upper layer of the environmental regulatory signals and the layer of the regulatory proteins indicate signaling events (red indicates an inhibitory effect while green indicates activation), while the arrows between the regulatory proteins and the denitrification enzymes indicate regulation of gene expression. The blue arrows between the transcriptional regulators indicate the cross-talk between the regulators which compete with each other to bind upstream of their targets.
When oxygen levels become limiting, denitrifiers are forced to activate the expression of the denitrification enzymes to avoid entrapment in anoxic conditions without energy. Recent evidence has shown that P. denitrificans displays a bet hedging strategy, a phenomenon that has been observed across a variety of prokaryotes which accept energetic penalties for a fraction of the population to achieve a long-term fitness advantage (Lycus et al., 2018). In the model denitrifier P. denitrificans this strategy involves the production of Nos in all cells, while Nir is only synthesized in a small number of cells.
Transcriptional regulation in the model denitrifier P. denitrificans is partly controlled by the regulators FnrP (fumarate and nitrate reduction protein), NNR (nitrite reductase and nitric oxide reductase regulator) and NarR (nitrate reductase regulator). Both FnrP and NNR are sensitive to oxygen and NO and are therefore involved in the switch between aerobic and anaerobic respiration to achieve maximum energy yields for the given environmental conditions (Van Spanning et al., 1995; Gaimster et al., 2018). To further fine-tune the denitrification network, the three transcriptional regulators FnrP, NnrR and NarR may serve as repressors of each other by competing for the binding upstream of their targets (Figure 2) (Giannopoulos et al., 2017). FnrP acts as an activator of the nar and nos operons and recognizes FNR-binding sequences (TTGAGAATTGTCAA and TTGACCTAAGTCAA) in the promoter region of the genes (Bouchal et al., 2010). Interaction of the FnrP 4Fe-4S cluster with O2 leads to a separation of the transcriptionally active FnrP dimer into monomers (Crack et al., 2016). Hence, denitrification is switched off in the presence of oxygen as O2 respiration provides significantly higher ATP yields. Additionally, it has been shown that the FnrP 4Fe-4S cluster interacts with multiple NO molecules leading to a dissociation of the transcriptional regulator into monomers. The transcriptional regulator NNR is homologous to FnrP and activates expression of the genes encoding nitrite (Nir) and nitric oxide reductases (Nor) (Van Spanning et al., 1995). Homologs of these transcriptional regulators have been identified in various other bacterial denitrifiers such as Pseudomonas species, P. stutzeri and Rhodobacter (Tosques et al., 1996; Elsen et al., 2004; Schreiber et al., 2007; Torres et al., 2017).
In Pseudomonas species the regulation of the denitrification machinery is equally dominated by members of the FNR superfamily such as the FnrP equivalent ANR (anaerobic regulator of arginine deaminase and nitrite reductase) (Schreiber et al., 2007). This transcription factor activates transcription of genes encoding for a nitrite transporter and a nitrite reductase if oxygen is limited. P. stutzeri encodes four FNR-type proteins which lack the cysteine residues required for the formation of a 4Fe-4S cluster (Vollack et al., 1999; Gaimster et al., 2018). NNR homologs have also been identified in a wide range of denitrifiers including Rhodobacter sphaeroides (Tosques et al., 1996). In R. sphaeroides, the regulator NnrR activates nitrite reductase and nitric oxide reductase. Outside of P. denitrificans, other transcriptional regulators have also been shown to be involved in the regulation of denitrification. These include the RegB/RegA two- component system. First discovered in Rhodobacter capsulatus, this system has been shown to regulate a large number of biological processes (Elsen et al., 2004). By controlling the expression of nitrite reductase, the RegB/RegA system in R spaeroides acts in concert with the regulator NnrR and therefore plays an important role in the denitrification cascade. In B. diazoefficiens, the denitrification machinery is regulated by two interconnected regulatory cascades, FixLJ-FixK2-NnrR and RegSR-NifA that detect low levels of oxygen outside of the cell (Torres et al., 2017).
The transcriptional regulators, FNR, NNR and NarR underpin the ability of bacteria to sense and respond to oxygen and the denitrification intermediates. However, there are other critical external factors that must be detected and integrated into the regulatory network of the cell. Copper has long been recognized as an important factor in the regulation of NosZ activity (Figure 2) (Sullivan et al., 2013). Around 20% of Europe’s arable lands are biologically copper deficient and as NosZ requires the unique multi-copper-sulphide centres, CuZ and CuA to bind and activate N2O, it places a high Cu demand on the bacterium (Sinclair and Edwards, 2008; Pauleta and Moura, 2017). Other enzymes in bacterial enzymes require Cu for activity, such as haem Cu-oxidases or superoxide dismutases but for all of these enzymes there are non-Cu alternatives that can perform the same function in the absence of Cu (Zumft, 2005). This is however not the case for NosZ. As a result, in Cu deficient conditions, the final reduction step cannot be carried out leading to truncated denitrification and emission of N2O. Studies carried out in 2012 demonstrated that copper-limited environments indeed lead to a downregulation of nosZ expression and an increased net N2O emission without a significant effect on the biomass of the culture (Felgate et al., 2012; Sullivan et al., 2013). A down-regulation of nosZ expression in copper limited medium additionally influences expression of genes controlled by vitamin B12 riboswitches as accumulation of N2O inactivates vitamin B12 (Sullivan et al., 2013). This work also showed that the accessory proteins NosC and NosR play an important role in copper-dependent expression of the nos-operon. Copper levels can therefore be manipulated in laboratory studies to create N2O or N2 genic conditions and induce global changes in gene expression, a useful tool to further understand the underlying regulatory and biochemical pathways (Felgate et al., 2012).
Other environmental factors such as zinc and pH have also been linked to transcriptional regulation of denitrification enzymes (Bergaust et al., 2010; Gaimster et al., 2018). Zinc depletion has been shown to upregulate the expression of nitric oxide reductase and nitrite reductase as well as nosC, which was upregulated nearly 10-fold (Neupane et al., 2017). Low soil pH increases the N2O:N2 ratio which has been linked to lowered levels of NosZ protein synthesis and assembly as transcription rates were unaffected by changes in pH (Bergaust et al., 2010). Denitrification in heterotrophs is highly dependent on carbon sources and therefore, increasing levels of organic carbon in the soil enhance denitrification rates as well as N2O emissions (Saggar et al., 2013). Both environmental factors and transcriptional regulators strongly influence when denitrification is switched on and once switched on affect the denitrification rate. Numerous studies have analyzed their influence in both a laboratory environment as well as in an agricultural background. Nevertheless, many variables involved in the switch between N2O emission and N2O consumption remain unknown.
Beyond DNA Binding Proteins - sRNAs Regulating the Nitrogen Cycle
sRNAs Indirectly Involved in Nitrogen Metabolism
Diazotrophs in the soil and the ocean are capable of using molecular nitrogen as the sole nitrogen source, preventing a loss of N from the biosphere and providing sources of fixed nitrogen. The key enzymes of nitrogen fixation, dinitrogenase and dinitrogenase reductase are energy driven and are therefore costly for the cell. Therefore, the nitrogen fixation process is tightly regulated at both the transcriptional and posttranscriptional level (Prasse and Schmitz, 2018). With increasing research into the regulatory role of sRNAs it is predicted that large numbers of these influence nitrogen cycle associated metabolism across many microorganisms (Figure 3). Direct involvement of sRNAs in the response to N-fluctuations in the environment or in the regulation of N2-fixation has not been identified until recently. Indirect participation of sRNAs in N-metabolism however has been reported previously. In cyanobacteria for instance, NsiR1 controls the formation of heterocysts as well as the switch to nitrogen fixation (Ionescu et al., 2010). This trans-encoded sRNA is conserved across many heterocyst-forming cyanobacteria and is dependent on the regulatory protein HetR which is required for cell differentiation in Anabaena. Similar to NsiR1, sRNAs NsiR2, NsiR8 and NsiR9 have been shown to be co-expressed with heterocyst-specific genes. However, to date no specific function has been assigned to these three sRNAs. Furthermore, ArrF of Azotobacter vinelandii is involved in the regulation of FeSII (DeLay and Gottesman, 2009), which plays a key role in the protection of the nitrogenase (N2-fixing) enzyme under oxidative conditions (Jung and Kwon, 2008). sRNAs indirectly involved in nitrogen assimilation include CyaR, GcvB and MmgR. CyaR, present in E. coli, inhibits the translation of an ammonium dependent NAD-synthethase responsible for the catalysis of NAD synthesis from either NH3 or glutamine as well as the nicotinic acid adenine dinucleotide (De Lay and Gottesman, 2009). The sRNA GcvB is one of the most highly conserved Hfq associated sRNAs in Gram-negative bacteria. It inhibits the expression of a number of ABC transporters responsible for transporting amino acids in E. coli and Salmonella Typhimurium (Sharma et al., 2007). In Sinorhizobium meliloti, hundreds of sRNAs have been identified, with the focus on the sRNA MmgR which shows expression patterns highly dependent on the available nitrogen source (Ceizel Borella et al., 2016). Further work is required to elucidate its exact role.
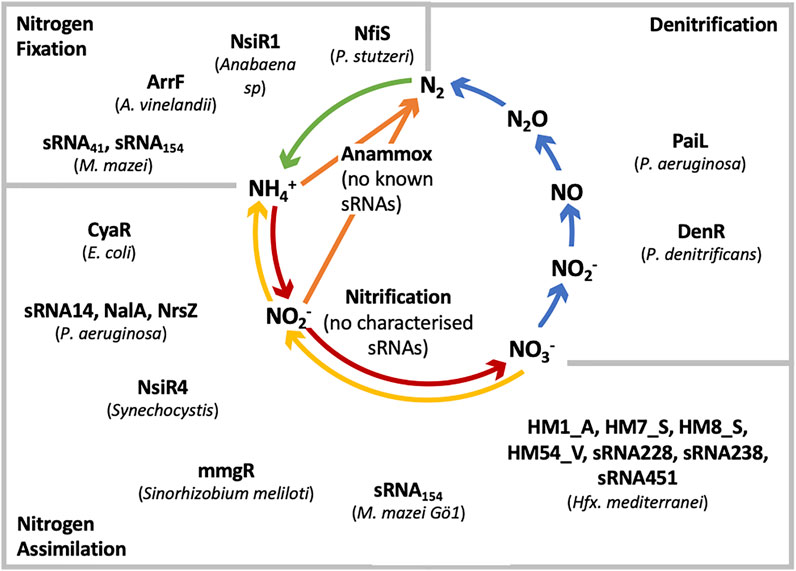
FIGURE 3. Microorganisms and known sRNAs that regulate the nitrogen cycle. The sRNAs are grouped into the processes of nitrogen fixation, assimilatory nitrate reduction and denitrification.
sRNAs Regulating Nitrogen Fixation
An sRNA found to be directly involved in N-metabolism, NfiS, was identified in the root associated bacterium P. stutzeri A1501. Via a stem loop in the sRNAs secondary structure it is predicted to bind to the 5′ region of nifK mRNA which encodes a subunit of the nitrogenase enzyme. This interaction increases mRNA half-life and thus increases the translation efficiency of nifK optimizing N-fixation (Zhan et al., 2016). The stability of NfiS appears to be strongly affected by the presence of Hfq, as the transcript is hardly detectable in an hfq deletion strain. A complete knockout of NfiS results in decreased nitrogenase activity, while an overexpression of this sRNA can lead to an increase of up to 150% activity. Although NfiS is highly conserved in P. stutzeri, it cannot be found in other bacterial species.
Many mechanistic features of the cellular transcription and translation machinery in archaea are more closely related to the eukaryotic counterparts, however characterisations of archaeal sRNAs have suggested similar mechanisms as observed in bacteria. The best-known examples of sRNA regulation of nitrogen fixation in Archaea are the methanoarchaea M. mazei and M. maripaludis. Both strains contain the global N-repressor NrpR which is known to transcriptionally regulate a variety of target genes in response to changes in N-levels. An RNA-seq study in M. mazei Gö1 under conditions of varied nitrogen availabilities lead to the identification of 242 putative sRNAs (Jäger et al., 2009; Jäger et al., 2012). The discovery of sRNA41 in M. mazei Gö1 introduced a sRNA in Archaea with a regulatory impact on the metabolic cycles of both carbon and nitrogen (Buddeweg et al., 2018). The sRNA is induced 100-fold in a N- rich environment compared to N-limitation and interacts with the mRNA encoding for an acetyl-coenzyme a decarbonylase/synthase (ACDS) complex (Buddeweg et al., 2018). In the absence of nitrogen, reduced amounts of sRNA41 result in the upregulation of the ACDS complex and a subsequent production of amino acids for the synthesis of nitrogenase. A further sRNA found in M mazei, sRNA154, was found to be exclusively present under nitrogen-limited conditions (Ehlers et al., 2011). A computational analysis of the transcriptional regulation network in M. acetivorans has shown that 5% of genes in this methanoarchaeon are regulated under nitrogen limitation. Two sRNAs, sRNA154 and sRNA159 were identified which include Nrp binding sites suggesting an involvement in gene regulation under N-limitation (Peterson et al., 2014). The first confirmed directly acting sRNA in M. mazei, sRNA154, is under direct control of the global N-repressor NrpR (Weidenbach et al., 2008; Weidenbach et al., 2010). By stabilizing the polycistronic mRNA encoding for the nitrogenase enzyme as well as stabilizing the transcription of the regulatory protein NrpA it enhances expression of the N-fixing machinery (Prasse et al., 2017). The sequence and structure of this sRNA is highly conserved across members of the Methanosarcinales.
Despite nitrification being an important part of the nitrogen cycle, few sRNAs have been shown to be involved in the regulation of this pathway due to a lack of studies around this topic. In the ammonia oxidizing archaea Nitrosopumilus maritimus six candidates for sRNAs have been identified and it is highly likely that there many more with a potential involvement in the nitrification process (Walker et al., 2010).
sRNAs Controlling Nitrogen Assimilation
A differential RNA-seq analysis of the cyanobacteria Anabaena sp. PCC7120 in response to N-availability identified over 600 transcriptional start sites indicating an abundance of cis- and trans-encoded sRNAs involved in the regulation of N-assimilation. Cyanobacteria are of importance in both aquatic and terrestrial ecosystems and are important links between the C- and the N- cycle. A cyanobacterial small RNA directly involved in the regulation of N-assimilation, NsiR4, was first reported by Klähn et al. in 2015. NsiR4 expression in cyanobacteria is stimulated during nitrogen limiting conditions via the transcriptional regulator NtcA which is known to regulate a variety of genes involved in nitrogen metabolism. It is predicted to interact with the 5′ UTR of gifA mRNA, encoding for the glutamine synthetase inactivating factor (IF)7. By affecting IF7 expression, the sRNA also alters the activity of glutamine synthetase, a key enzyme in biological nitrogen assimilation (Klähn et al., 2015).
In Pseudomonas aeruginosa, the putative sRNA NalA is encoded upstream of the nitrate assimilation operon nirBD–PA1779–cobA. The transcription of this sRNA is σ54 and NtrC-dependent (Romeo et al., 2012). A deletion mutant of NalA was unable to grow in presence of nitrate as the sole nitrogen source, instead it grew similarly to the parental strain in presence of ammonium. The results showed that NalA sRNA and nitrate are required for transcription of the nitrate assimilation operon, being an essential sRNA for the assimilation of nitrate (Romeo et al., 2012). Further studies performed in P. aeruginosa allowed the identification of sRNAs related to detoxification of industrial cyanide-containing wastewaters. For this purpose, a differential expression study was carried out by RNA-seq from cells cultured with a cyanide-containing wastewater, sodium cyanide or ammonium chloride as the sole nitrogen source. Among the sRNAs identified, sRNA14 (overexpressed in the presence of ammonium) stood out, as its putative target genes include the nitrilase NitC, essential for cyanide assimilation, the FAD-dependent oxidoreductase NitH; and the glutamine synthetase, related to ammonia assimilation. Moreover, sRNA14 showed a high conservation among enterobacterial species (Olaya-Abril et al., 2019).
In the archaeon Haloferax mediterranei, sRNAs have been studied to elucidate their possible role in the regulation of nitrogen assimilation in Haloarchaea (Payá et al., 2018; Payá et al., 2020). The initial identification of sRNAs in H. mediterranei was performed using a library of sRNAs identified in other archaeal species which resulted in the discovery of 295 putative sRNAs genes (hot spots) in the genome of H. mediterranei. Via bioinformatic and RNomic approaches, 88 sRNAs were identified. The differential expression analysis of these 88 sRNAs showed 16 sRNAs with different expression patterns according to the nitrogen source. The expression of their predicted target genes also depends strongly on the nitrogen source. Three regulatory mechanisms mediated by sRNAs were proposed in this study (Figure 4). The sRNA HM8_S which is overexpressed in presence of nitrate is predicted to target glutamate dehydrogenase, under expressed in presence of nitrate. Therefore, this sRNA could negatively regulate the expression of glutamate dehydrogenase. Both HM7_S and HM54_V sRNAs, overexpressed in presence of nitrate, are predicted to target transcriptional regulators belonging to the ArsR family, whose expression depends on the nitrogen source. Finally, the putative target of HM1_A (overexpressed in the presence of ammonium) is an ammonium transporter (expressed in the presence of nitrate or under nitrogen starvation) and therefore this sRNA could be involved in the regulation of ammonium uptake from the extracellular medium. However, more work is needed to confirm these regulatory mechanisms (Payá et al., 2018).
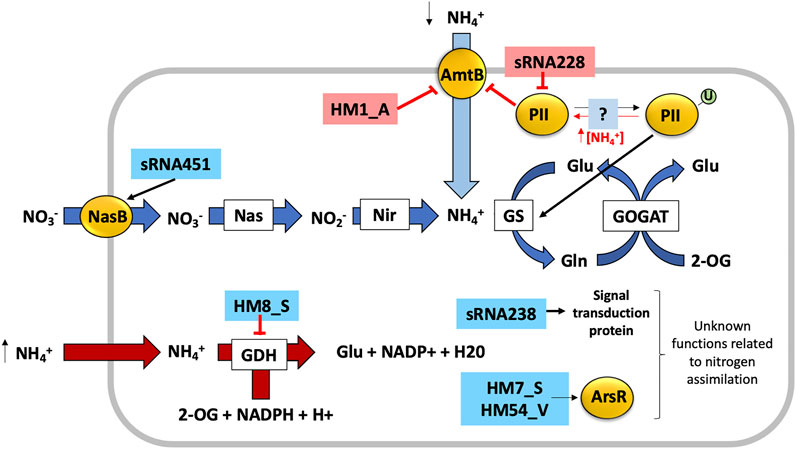
FIGURE 4. Proposed regulation of nitrogen assimilation by sRNAs in H. mediterranei. The sRNAs highlighted in red correspond to sRNAs expressed in presence of ammonium, while sRNAs shaded blue correspond to those expressed in the presence of nitrate as a source of nitrogen. The red arrows indicate negative regulation and the black arrows indicate positive regulation of their respective target genes.
The second step in the identification of sRNAs in H. mediterranei results in the identification of the complete sRNAome in presence of ammonium or nitrate as the sole nitrogen source. 460 sRNAs were present in both conditions, 102 of which showed differences in their transcriptional patterns. Specifically, sRNAs with potential target genes related to nitrogen metabolism, such as nosL, glnK1, gdh, glnA2, nasB, ilvB3, ilvE2, ilvAm, rrfh1, tyrA, gst2, gabT, gaD2, argD, gltp, purL, argB, gatD, nadE, fdx, exsB, gcvP1 and pyrF also presented differences in their transcriptional expression patterns according to the nitrogen source. From these findings, three potential regulatory mechanisms of nitrogen metabolism pathways mediated by sRNAs were proposed (Figure 4): 1) sRNA228 could be involved in the repression of nitrogen regulatory protein PII (glnK1) in the presence of ammonium, potentially through the posttranscriptional degradation of glnK1 mRNA preventing its transcription and therefore the activation of the GS/GOGAT pathway; 2) the sRNA451 could be involved in the positive regulation of the nitrate/nitrite transporter (nasB) expression in presence of nitrate as nitrogen source, by transcriptional stabilization of the nasB mRNA, increasing nitrate uptake under these conditions; and 3) sRNA238 could be involved in the transcriptional stabilization of the HFX_RS05100 gene (both overexpressed in presence of nitrate). Although HFX_RS05100 encodes a signal transduction protein of unknown function, the results of this work suggest that it may be involved in nitrogen metabolism (Payá et al., 2020).
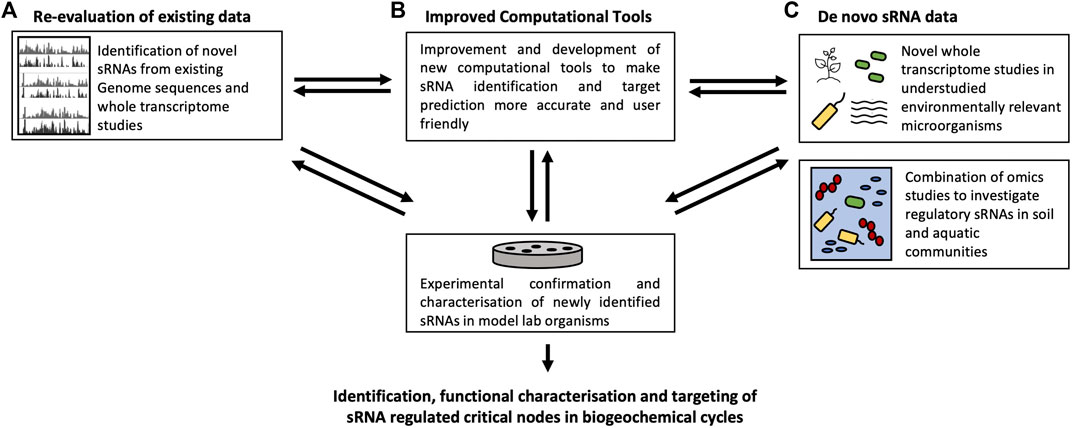
FIGURE 5. Roadmap for the progression of sRNA research in environmental cycles includes (A) the Re-evaluation of existing data, (B) the development of improved computational tools and (C) the generation of de-novo sRNA data.
sRNAs Controlling Denitrification
The importance of sRNA regulation in denitrifiers is a relatively recent discovery. However, 167 putative sRNAs across the P. denitrificans genome have now been identified when cultured under denitrifying conditions (Gaimster et al., 2016). Over one third of these sRNAs were differentially expressed between N2 and N2O emitting cultures suggesting a role of these sRNAs in production or consumption of the greenhouse gas. Several of these sRNAs showed sequence homology and conservation across other species in the α-proteobacteria. Interestingly, one particular sRNA, intergenic 28, showed sequence homology to members of the β-proteobacteria, including members of the Bordetella genus which include strains of human host-restricted pathogens as well as free-living environmental strains isolated from both aquatic and soil environments. Commonly predicted targets of sRNAs were transcriptional regulators such as Xre, Fis and TetR- Family regulators, which may act globally. This is consistent with other studies in which global regulators in other bacterial species have been shown to be subject to regulation by multiple Hfq-dependent sRNAs. P. denitrificans is predicted to encode an Hfq protein, Pden_4124, that has 95% sequence identity to Hfq found in R. sphaeroides and 54% sequence identity to P. aeruginosa Hfq. Many sRNAs found in both these bacteria are Hfq-dependent suggesting the same may be the case in P. denitrificans (Gamister et al., 2016). Additional predicted targets for sRNAs in P. denitrificans are transport proteins, also the most commonly predicted sRNA target in the marine denitrifier R. pomeroyi (Rivers et al., 2016).
Mechanistic studies carried out by Gaimster et al. then went on to report a novel regulatory pathway controlling denitrification via a single sRNA, sRNA29 (DenR) (Gaimster et al., 2019). DenR is suggested to stabilize the expression of a previously unknown GntR-type transcriptional regulator, NirR, which in turn represses the denitrification rate through repressing NirS, resulting in reduced N2O emissions. The predicted region of interaction is a 7bp-seed region located within the CDS of nirR, and the underlying mechanism is currently being resolved. GntR-type regulators have been identified across many bacterial species in which they play crucial roles in the regulation of intracellular processes. They are named after the gluconate-operon repressor in Bacillus subtilis and they consist of a conserved N-terminal helix-turn-helix DNA-binding domain, which is linked to a C-terminal signaling domain. The overexpression of DenR also results in altered expression levels of 53 other genes that are mostly genes of either unknown function, genes involved in energy metabolism or transport as well as genes involved in carbohydrate metabolism. Interestingly, DenR has been found to be conserved across several denitrifying bacterial species in the Rhodobacteraceae genus. This includes the closely related species Paracoccus aminophilus but also the more distantly related marine denitrifier Ruegeria pomeroyi. All these species encode a transcriptional regulator with homology to NirR, suggesting a similar, conserved mode of action. Mechanistic studies for other sRNA that are differentially regulated between N2 and N2O genic cultures are currently underway. Although there are limited findings in other denitrifiers, for the opportunistic pathogen P. aeruginosa, the anaerobically induced sRNA Pail is known to be required for efficient denitrification by affecting the conversion of nitrite to nitric oxide (Tata et al., 2017).
The Role of sRNAs in Other Major Environmental Cycles
As marine dissolved organic matter contains equal concentrations of carbon as the Earth’s atmosphere it represents a crucial component of the global carbon cycle. Despite microorganisms being important drivers if the carbon cycle, the microbial activities that regulate the turnover of dissolved organic matter still remain largely unsolved (McCarren et al., 2010). Studies focussing on marine cyanobacteria have identified key sRNAs involved in the regulation of the photosystem which is involved in photosynthesis. Several sRNAs have been found to play an important role in the response to light stress including six sRNAs in the marine bacterium Synechococcus that could have a regulatory effect on the light harvesting apparatus, a major driver of biogeochemical cycles through carbon fixation (Gierga et al., 2012). The sRNA PsrR1 in the cyanobacteria Synechocystis 6803 was established to downregulate the expression of several photosynthesis-related genes in response to high light intensity by targeting several photosynthesis related genes including photosystem I-related proteins, cytochrome c553 and subunit N of the light-independent protochlorophyllide reductase (Gierga et al., 2012; Kopf and Hess, 2015; Pei et al., 2017, Hu and Wang, 2018). The sRNA appears to be regulated by the RpaB protein which is responsible for an increase in the expression of several genes under low light.
Equally, the discovery of 99 putative sRNAs under carbon and nitrogen limitation in the model marine bacterium Ruegeria pomeroyi is of great interest, as this bacterium is suggested to scavenge for alternate sources of organic C, influencing the ratios of major biomolecules in C-limited conditions (McCarren et al., 2010; Rivers et al., 2016). Fourteen of these sRNAs were differentially expressed under C- and N- nutrient limited cultures and their predicted targets include genes involved in transport, cell-cell interactions and nitrogen metabolism. Interestingly, one sRNA showed homology to 6S RNA which is of importance in E. coli and many other bacteria and has been found to downregulate multiple genes under environmentally stressful conditions, including nutrient limitation (Cavanagh and Wassarman, 2014). In R. pomeroyi, 6S was upregulated under C- and N- limited conditions and also showed interesting expression patterns in the context of sulfur metabolism.
Carbon and sulfur cycling in the biosphere are tightly interwoven through various biological processes carried out by marine microorganisms. Dimethylsulfonioproprionate (DMSP) represents not only a major carbon source, but also a source of reduced sulfur in the ocean. It is produced by phytoplankton and is metabolized by bacteria via two separate pathways, the demethylation pathway or the cleavage pathway, that result either in the formation methanethiol (MeSH) or dimethyl sulfide (DMS) respectively (Reisch et al., 2013). MeSH is a major source of reduced carbon and sulfur utilized by marine microbes as an important food source while the production of DMS accounts for a significant flux of sulfur from the ocean (Burns et al., 2016). DMS emissions have been linked to cloud albedo and climate and it has also been shown that DMS emissions influence the level of ocean carbon uptake underlining the close link of carbon and sulfur cycle. The genes involved in both pathways have been extensively studied, however there is little knowledge on the underlying regulatory processes controlling the pathways (Williams and Todd, 2019). It has however been shown that increased light intensity and nitrogen starvation influence levels of DMSP production (Kettles et al., 2014). The enzyme DsyB was recently identified to catalyze a key step in the DMSP production pathway in phytoplankton. In the diatom F. cylindrus both DMSP production and DSYB transcription are increased when nitrogen becomes limiting (Curson et al., 2018). The discovery of 182 potential sRNAs with putative targets including a wide range of regulatory, transport and signaling molecules in R. pomeroyi when grown on DMSP, DMSP intermediates or methionine suggested a potential involvement of sRNAs in posttranscriptional regulation of both DMSP metabolic pathways. Indeed, a knockout of sRNA129 showed significant alterations in the release of DMS and MeSH compared to the wild type R. pomeroyi strain (Burns et al., 2016). Further sRNAs such as sRNA42 and sRNA53, suggested to play a metabolic role via the demethylation pathway, have been predicted to target DMSP lyase dddQ mRNA raising a possibility of post-transcriptional regulation of dddQ. Uncovering the roles of these and other sRNAs found in marine bacteria will enhance the understanding of the cycling of sulfur and other elements in the ocean which is of major importance (Burns et al., 2016).
Human activities have significantly increased the availability of phosphorus in marine habitats leading to eutrophication and increasing primary production of cyanobacterial blooms. A study by Teikari et al., examined the transcriptomic landscape of the cyanobacterium Anabaena sp., one of the most common bloom-forming bacteria, in response to P limitation to increase the understanding of how the changing levels of P affect these diazotrophs. Indeed, they identified differentially expressed intergenic regions which could give rise to sRNAs crucial in the functioning of the cell in response to changing P-levels (Teikari et al., 2015).
Evidence suggests that the archaeal sRNA162 plays a crucial role in the adaptation to different carbon sources, such as when cells switch from methanol to trimethylamine metabolism in the methanoarchaeon Methanosarcina mazei (Jäger et al., 2012). Overexpression of sRNA162 results in a reduced production of its trans-encoded target, the ArsR-type transcriptional regulator MM2441, which affects the transcription level of a number of soluble methyltransferase genes. These genes are recognized as the most highly regulated genes in methanoarchaea and are involved in the degradation of methanol and methylamines (Veit et al., 2005; Bose et al., 2008; Krätzer et al., 2009). The sRNA is suggested to be constitutively expressed during exponential growth phase with methanol as a carbon source, repressing MM2441 by blocking the translation initiation region (Jäger et al., 2012). A fast turnover of sRNA162 however ensures that low MM2441 protein levels are maintained that are still sufficient to repress the mtmB2C2 operon encoding for a methyltransferase and a cognate corrinoid protein. In stationary phase, the turnover time of sRNA162 is reinforced leading to full translational repression of MM2441 and subsequent expression of the mtmB2C2 operon. Additional to the trans-encoded target MM2441, sRNA162 also represents a cis acting RNA, interacting with the 5′-UTR of MM2442, encoding a conserved protein of unknown function.
How to Advance the Field of sRNA Research in Environmental Cycles
Although sRNAs regulate a wide range of important biological processes, our current understanding of their role is far from complete, particularly with respect to the microbial ecology of diverse environments. A manipulation of only a handful of these sRNAs in the lab can lead to drastic changes in the response of the lab organism to an experimental condition. For instance, overexpression of DenR in P. denitrificans leads to a drastic decrease in the levels of N2O emitted from the bacterial culture (Gaimster et al., 2019). This suggests that much larger networks of currently completely unknown sRNAs must be involved in an entire environmental response. Uncovering these networks would contribute largely to our understanding of the environmental stimuli that result in the switch from complete denitrification to incomplete denitrification and N2O emissions as well as other processes driving our major biogeochemical cycles.
The use of high-throughput RNA-seq has led to significant advances in the identification of sRNAs. However, the majority of available information for sRNAs associated with biogeochemical cycles is restricted to a few model organisms, and even in these only a few selected sRNAs and their targets have been characterized in detail. Traditionally, coding genes were annotated using automated pipelines, while non-coding regions were overlooked (Figure 5A). The diverse characteristics of sRNAs such as their variation in length and secondary structures as well as a lack of sRNA conservation across distantly related genomes have made their computational discovery a difficult task. Advances in computational biology such as comparative genomics, RNA structure and thermodynamic stability-based approaches as well as transcriptional signal-based sRNA identification have contributed to the identification of a plethora of sRNAs (Sridhar and Gunasekaran, 2013). Though the majority of sRNAs have been identified using comparative genomics, transcriptional signal-based approaches are promising in the discovery of novel intergenic sRNAs (Rajendran et al., 2020). The breadth of bacterial and archaeal species with fully sequenced genomes and pre-existing whole transcriptome studies may well also contribute to the identification of novel sRNA. In Salmonella Typhimurium SL1344 novel and refined sRNA identification methodologies have led to the discovery of large numbers of novel sRNA, suggesting that sRNA genes may even outnumber protein coding genes (Houserova et al., 2021).
Once an sRNA has been identified, the critical bottleneck in the functional characterization is the detection of sRNA targets. By identifying sRNA targets it is possible to integrate this regulatory RNA into the existing models of regulatory networks that fine-tune all microbial processes. This is a crucial step in the development of future mitigation strategies to counter anthropogenically induced shifts in the natural biogeochemical cycles. Experimental target identification via genetic screens, knockouts and sRNA overexpression often followed by proteomics and microarray analysis or qRT-PCR are time-consuming and laborious (Backofen and Hess, 2010; Georg et al., 2019). Therefore, efficient computational target prediction tools are highly desirable (Figure 5B). Existing tools predict sRNA targets based on sequence, thermodynamic scoring of mRNA-sRNA mixed duplexes and RNA secondary structure. However, the accuracy of these tools is highly variable, and their use is complex (Kumar et al., 2020). Therefore, we can assume that many sRNAs remain undiscovered, especially in organisms that are less studied. It will be a major challenge to adapt these methods to gain an idea of the sRNA-targets interactions in complex ecological environments. A more user-friendly approach is necessary to make sRNA identification and characterization more accessible in the future.
To increase our understanding of sRNAs outside of lab model organisms, it is crucial to extend the study of sRNAs in single organisms to a metagenomic scale. In our natural environment, microorganisms do not live by themselves. Instead, they are found in communities which often work together in the response to environmental stresses. Integrative omics approaches can give insights into genes, RNA, proteins and metabolites present in the entire microbial community with a particular function in the environment (Figure 5C). Novel sequencing methods based on sRNAs in a variety of human samples can generate complete metagenomic profiles giving insights into the bacterial communities present in patient samples which is a promising tool for the analysis of both the entire sRNA content and the microbial profile of a sample (Mjelle et al., 2020). Microbial metatransciptomic data sets from the ocean have already revealed the abundance of sRNA in microbial communities and their importance in processes such as carbon metabolism and nutrient acquisition (Shi et al., 2009). Many of these sRNAs were identified as part of pyrosequencing of the total RNA extracted from microbial communities extracted from the Hawaiian ocean. A large fraction of the sequences that shared no homology with known proteins were found to be comprised of known as well as novel, uncharacterized sRNAs (Shi et al., 2009). Another study identified an abundance of diverse sRNAs from an extremophilic microbial community in the Atacama Desert (Gelsinger et al., 2020). Putative targets of these sRNA are involved in osmotic adjustments to major rain events as well as nutrient acquisition which underpins the importance of sRNAs in the community stress response in the natural environment. Community studies like these are important contributors to uncover large numbers of sRNAs in situ that are of environmental importance which can then be further characterized in vitro (Gierga et al., 2012).
sRNA Applications
Recent work has demonstrated that integrated analyses of the microbiome and the bacterial as well as human small noncoding RNAs could be key in the development of novel diagnostic tools (Tarallo et al., 2019; Mjelle et al., 2020; Vogel et al., 2020). For instance, host-microbiome dysbiosis in colorectal cancer patients results in an altered sRNA profile in the human stool compared to that observed in healthy patient samples (Tarallo et al., 2019). Pathogenic bacteria such as Mycobacterium tuberculosis are able to secrete sRNAs which can subsequently be detected in the patient plasma. In both examples, sRNAs could potentially act as biomarkers for diseases. A pathogen often associated with colorectal cancer is Fusarium nucleatum (Brennan and Garrett, 2019). It has been suggested that selective depletion of this pathogen in the colon by administrating a short antisense sRNA targeting the mRNA of an essential gene could provide crucial insights into the link of F. nucleatum to disease (Vogel, 2020). Despite a lack of knowledge around the transcriptome structure and cell envelope of some of these potential target organisms, programmable RNA ‘antibiotics’ are a promising approach to target antibiotic resistant bacteria in the future.
These findings could also be applied in non-host microbiomes. Having established the importance of singular sRNAs in a physiological response, an analysis of the entirety of sRNA present in an environmental sample could provide valuable insights into the specific responses of each microbe present in the sample. Presence of known sRNAs could provide information about the nature of an environmental response. Similar to the potential use of sRNA biomarkers in infection, they could act as biomarkers or ‘ecomarkers’ to identify, for example, whether the microbial communities in an agricultural soil are contributing to the production or consumption of N2O. The idea of RNA ‘antibiotics’ could also be translated to a non-host environment. For example, sRNAs could be engineered to target key microbes or key enzymes in the denitrification cascade, known to be involved in switching on or off N2O production in situ. These could then be added to fertilizers, giving us a chance to control greenhouse gas emissions from the agriculture industry.
Conclusion
Microbial sRNAs have already proven to modulate a range of microbial responses. Meta analyses of communities in conjunction with mechanistic data obtained computationally and experimentally from model organisms as well as advancements in the computational sRNA and sRNA-target prediction tools, will are vital and needed to resolve the complex sRNA regulatory networks underpinning biogeochemical cycles such as the nitrogen cycle in the environment. A broader understanding of all sRNAs involved in the switch between N2O production and N2O consumption could contribute to the development of mitigation strategies and sRNAs could be applied to counter the rising levels of the greenhouse gas while maintaining global food security.
Author Contributions
SM and GP reviewed the literature, co-wrote the manuscript, and prepared the figures. M-JB, AG, DR, JE and GR conceived the idea and co-edited the drafts.
Funding
This work was funded by the Biotechnology and Biological Sciences Research Council (United Kingdom) (BB/L022796/1, BB/M00256X/1, BB/S008942/1) and a University of East Anglia studentship as well as a Generalitat Valenciana (Spain) studentship (grant ACIF/2018/200), “Programa Propio para el Formento de la I+D+I del Vicerrectorado de Investigación y Transferencia de Conociemiento (GRE20-02-C)” University of Alicante.
Conflict of Interest
The authors declare that the research was conducted in the absence of any commercial or financial relationships that could be construed as a potential conflict of interest.
References
Babitzke, P., and Romeo, T. (2007). CsrB sRNA Family: Sequestration of RNA-Binding Regulatory Proteins. Curr. Opin. Microbiol. 10, 156–163. doi:10.1016/j.mib.2007.03.007
Backofen, R., and Hess, W. R. (2010). Computational Prediction of sRNAs and Their Targets in Bacteria. RNA Biol. 7 (1), 33–42. doi:10.4161/rna.7.1.10655
Bardill, J. P., Zhao, X., and Hammer, B. K. (2011). The Vibrio cholerae Quorum Sensing Response Is Mediated by Hfq-dependent sRNA/mRNA Base Pairing Interactions. Mol. Microbiol. 80, 1381–1394. doi:10.1111/j.1365-2958.2011.07655.x
Battesti, A., Majdalani, N., and Gottesman, S. (2011). The RpoS-Mediated General Stress Response inEscherichia Coli. Annu. Rev. Microbiol. 65 (1), 189–213. doi:10.1146/annurev-micro-090110-102946
Beisel, C. L., and Storz, G. (2010). Base Pairing Small RNAs and Their Roles in Global Regulatory Networks. FEMS Microbiol. Rev. 34 (5), 866–882. doi:10.1111/j.1574-6976.2010.00241.x
Bergaust, L., Mao, Y., Bakken, L. R., and Frostegård, A. (2010). Denitrification Response Patterns during the Transition to Anoxic Respiration and Posttranscriptional Effects of Suboptimal pH on Nitrogen Oxide Reductase in Paracoccus Denitrificans. Appl Environ Microbiol. 76, 6387–6396. doi:10.1128/AEM.00608-10
Bernhard, A. (2010). The Nitrogen Cycle: Processes, Players and Human Impact. Nat. Educ. Knowledge Project 3 (10), 25
Bos, J., Duverger, Y., Thouvenot, B., Chiaruttini, C., Branlant, C., Springer, M., et al. (2013). The sRNA RyhB Regulates the Synthesis of the Escherichia coli Methionine Sulfoxide Reductase MsrB but Not MsrA. PLoS One. 8 (5), e63647. doi:10.1371/journal.pone.0063647
Bose, A., Pritchett, M. A., and Metcalf, W. W. (2008). Genetic Analysis of the Methanol- and Methylamine-specific Methyltransferase 2 Genes of Methanosarcina Acetivorans C2A. J Bacteriol. 190, 4017–4026. doi:10.1128/JB.00117-08
Bossi, L., and Figueroa-Bossi, N. (2016). Competing Endogenous RNAs: a Target-Centric View of Small RNA Regulation in Bacteria. Nat. Rev. Microbiol. 14 (12), 775–784. doi:10.1038/nrmicro.2016.129
Bouchal, P., Struhárová, I., Budinská, E., Šedo, O., Vyhlídalová, T., Zdráhal, Z., et al. (2010). Unraveling an FNR Based Regulatory Circuit in Paracoccus Denitrificans Using a Proteomics-Based Approach. Biochim. Biophys. Acta. 1804, 1350–1358. doi:10.1016/j.bbapap.2010.01.016
Boudes, M., Lazar, N., Graille, M., Durand, D., Gaidenko, T. A., Stewart, V., et al. (2012). The Structure of the NasR Transcription Antiterminator Reveals a One-Component System with a NIT Nitrate Receptor Coupled to an ANTAR RNA-Binding Effector. Mol. Microbiol. 85, 431–444. doi:10.1111/j.1365-2958.2012.08111.x
Boudvillain, M., Figueroa-Bossi, N., and Bossi, L. (2013). Terminator Still Moving Forward: Expanding Roles for Rho Factor. Curr. Opin. Microbiol. 16, 118–124. doi:10.1016/j.mib.2012.12.003
Bouvier, M., Sharma, C. M., Mika, F., Nierhaus, K. H., and Vogel, J. (2008). Small RNA Binding to 5′ mRNA Coding Region Inhibits Translational Initiation. Mol. Cel. 32 (6), 827–837. doi:10.1016/j.molcel.2008.10.027
Brennan, C. A., and Garrett, W. S. (2019). Fusobacterium Nucleatum - Symbiont, Opportunist and Oncobacterium. Nat. Rev. Microbiol. 17, 156–166. doi:10.1038/s41579-018-0129-6
Brusseau, M. L. (2019). Ecosystems and Ecosystem Services. Environmental and Pollution Science. Third Edition. Acad Press, 89–102. doi:10.1016/b978-0-12-814719-1.00006-9
Buddeweg, A., Sharma, K., Urlaub, H., and Schmitz, R. A. (2018). sRNA41affects Ribosome Binding Sites within Polycistronic mRNAs inMethanosarcina mazeiGö1. Mol. Microbiol. 107, 595–609. doi:10.1111/mmi.13900
Burns, A. S., Bullock, H. A., Smith, C., Huang, Q., Whitman, W. B., and Moran, M. A. (2016). Small RNAs Expressed during Dimethylsulfoniopropionate Degradation by a Model Marine Bacterium. Environ. Microbiol. Rep. 8 (5), 763–773. doi:10.1111/1758-2229.12437
Cavanagh, A. T., and Wassarman, K. M. (2014). 6S RNA, a Global Regulator of Transcription inEscherichia Coli,Bacillus Subtilis, and beyond. Annu. Rev. Microbiol. 68, 45–60. doi:10.1146/annurev-micro-092611-150135
Ceizel Borella, G., Lagares, A., and Valverde, C. (2016). Expression of theSinorhizobium Melilotismall RNA genemmgRis Controlled by the Nitrogen Source. FEMS Microbiol. Lett. 363, fnw069. doi:10.1093/femsle/fnw069
Chai, W., and Stewart, V. (1999). RNA Sequence Requirements for NasR-Mediated, Nitrate-Responsive Transcription Antitermination of the Klebsiella Oxytoca M5al nasF Operon Leader 1 1Edited by M. Gottesman. J. Mol. Biol. 292, 203–216. doi:10.1006/jmbi.1999.3084
Chan, T. Y. K. (2011). Vegetable-borne Nitrate and Nitrite and the Risk of Methaemoglobinaemia. Toxicol. Lett. 200, 107–108. doi:10.1016/j.toxlet.2010.11.002
Chao, Y., Li, L., Girodat, D., Förstner, K. U., Said, N., Corcoran, C., et al. (2017). In Vivo Cleavage Map Illuminates the Central Role of RNase E in Coding and Non-coding RNA Pathways. Mol. Cel. 65, 39–51. doi:10.1016/j.molcel.2016.11.002
Chen, H., Dutta, T., and Deutscher, M. P. (2016). Growth Phase-dependent Variation of RNase BN/Z Affects Small RNAs. J. Biol. Chem. 291, 26435–26442. doi:10.1074/jbc.M116.757450
Chen, S., Perathoner, S., Ampelli, C., and Centi, G. (2019). “Electrochemical Dinitrogen Activation: To Find a Sustainable Way to Produce Ammonia,” in “Electrochemical Dinitrogen Activation: To Find a Sustainable Way to Produce Ammonia” Studies in Surface Science and Catalysis (Elsevier), Vol. 178, 31–46. doi:10.1016/b978-0-444-64127-4.00002-1
Conthe, M., Wittorf, L., Kuenen, J. G., Kleerebezem, R., Hallin, S., and Van Loosdrecht, M. C. M. (2018). Growth Yield and Selection of nosZ Clade II Types in a Continuous Enrichment Culture of N2 O Respiring Bacteria. Environ. Microbiol. Rep. 10 (3), 239–244. doi:10.1111/1758-2229.12630
Crack, J. C., Hutchings, M. I., Thomson, A. J., and Le Brun, N. E. (2016). Biochemical Properties of Paracoccus Denitrificans FnrP: Reactions with Molecular Oxygen and Nitric Oxide. J. Biol. Inorg. Chem. 21 (1), 71–82. doi:10.1007/s00775-015-1326-7
Curson, A. R. J., Williams, B. T., Pinchbeck, B. J., Sims, L. P., Martínez, A. B., Rivera, P. P. L., et al. (2018). DSYB Catalyses the Key Step of Dimethylsulfoniopropionate Biosynthesis in Many Phytoplankton. Nat. Microbiol. 3, 430–439. doi:10.1038/s41564-018-0119-5
Darfeuille, F., Unoson, C., Vogel, J., and Wagner, E. G. H. (2007). An Antisense RNA Inhibits Translation by Competing with Standby Ribosomes. Mol. Cel. 26, 381–392. doi:10.1016/j.molcel.2007.04.003
De Lay, N., and Gottesman, S. (2009). The Crp-Activated Small Noncoding Regulatory RNA CyaR (RyeE) Links Nutritional Status to Group Behavior. J Bacteriol. 191, 461–476. doi:10.1128/JB.01157-08
De Lay, N., and Gottesman, S. (2011). Role of Polynucleotide Phosphorylase in sRNA Function in Escherichia coli. RNA. 17 (6), 1172–1189. doi:10.1261/rna.2531211
De Lay, N., Schu, D., and Gottesman, S. (2013). Bacterial Small RNA-based Negative Regulation: Hfq and its Accomplices. J. Biol. Chem. 288 (12), 7669–8003. doi:10.1074/jbc.R112.441386
Dixon, R., and Kahn, D. (2004). Genetic Regulation of Biological Nitrogen Fixation. Nat. Rev. Microbiol. 2 (8), 621–631. doi:10.1038/nrmicro954
Dixon, R. A., Buck, M., Drummond, M., Hawkes, T., Khan, H., MacFarlane, S., et al. (1986). Regulation of the Nitrogen Fixation Genes inKlebsiella Pneumoniae: Implications for Genetic Manipulation. Plant Soil. 90, 225–233. doi:10.1007/BF02277399
Domeignoz-Horta, L. A., Putz, M., Spor, A., Bru, D., Breuil, M. C., Hallin, S., et al. (2016). Non-denitrifying Nitrous Oxide-Reducing Bacteria - an Effective N2O Sink in Soil. Soil Biol. Biochem. 103, 376–379. doi:10.1016/j.soilbio.2016.09.010
Durand, S., Tomasini, A., Braun, F., Condon, C., and Romby, P. (2015). sRNA and mRNA Turnover in Gram-Positive Bacteria. FEMS. Microbiol. Rev. 39 (3), 316–330. doi:10.1093/femsre/fuv007
Dutta, T., and Srivastava, S. (2018). Small RNA-Mediated Regulation in Bacteria: A Growing Palette of Diverse Mechanisms. Gene. 656 (656), 60–72. doi:10.1016/j.gene.2018.02.068
Ehlers, C., Jäger, D., and Schmitz, R. A. (2011). Establishing a Markerless Genetic Exchange System forMethanosarcina mazeiStrain Gö1 for Constructing Chromosomal Mutants of Small RNA Genes. Archaea. 2011, 1–7. doi:10.1155/2011/439608
Elsen, S., Swem, L. R., Swem, D. L., and Bauer, C. E. (2004). RegB/RegA, a Highly Conserved Redox-Responding Global Two-Component Regulatory System. Microbiol Mol Biol Rev. 68 (2), 263–279. doi:10.1128/MMBR.68.2.263-279.2004
Felgate, H., Giannopoulos, G., Sullivan, M. J., Gates, A. J., Clarke, T. A., Baggs, E., et al. (2012). The Impact of Copper, Nitrate and Carbon Status on the Emission of Nitrous Oxide by Two Species of Bacteria with Biochemically Distinct Denitrification Pathways. Environ. Microbiol. 14 (7), 1788–1800. doi:10.1111/j.1462-2920.2012.02789.x
Fischer, S., Benz, J., Späth, B., Maier, L.-K., Straub, J., Granzow, M., et al. (2010). The Archaeal Lsm Protein Binds to Small RNAs. J. Biol. Chem. 285 (45), 34429–34438. doi:10.1074/jbc.M110.118950
Franze de Fernandez, M. T., Eoyang, L., and August, J. T. (1968). Factor Fraction Required for the Synthesis of Bacteriophage Qβ-RNA. Nature. 219 (5154), 588–590. doi:10.1038/219588a0
Fröhlich, K. S., and Vogel, J. (2009). Activation of Gene Expression by Small RNA. Curr. Opin. Microbiol. 12, 674–682. doi:10.1016/j.mib.2009.09.009
Gaimster, H., Chalklen, L., Alston, M., Munnoch, J. T., Richardson, D. J., Gates, A. J., et al. (2016). Genome-wide Discovery of Putative sRNAs in Paracoccus Denitrificans Expressed under Nitrous Oxide Emitting Conditions. Front. Microbiol. 7 (7), 1806. doi:10.3389/fmicb.2016.01806
Gaimster, H., Alston, M., Richardson, D. J., Gates, A. J., and Rowley, G. (2018). Transcriptional and Environmental Control of Bacterial Denitrification and N2O Emissions. FEMS. Microbiol. Lett. 365, 5. doi:10.1093/femsle/fnx277
Gaimster, H., Hews, C. L., Griffiths, R., Soriano-Laguna, M. J., Alston, M., Richardson, D. J., et al. (2019). A Central Small RNA Regulatory Circuit Controlling Bacterial Denitrification and N2O Emissions. mBio. 10 (4), e01165–19. doi:10.1128/mBio.01165-19
Galloway, J. N., and Cowling, E. B. (2002). Reactive Nitrogen and the World: 200 Years of Change. AMBIO: A J. Hum. Environ. 31 (2), 64–71. doi:10.1579/0044-7447-31.2.64
Gelsinger, D. R., Uritskiy, G., Reddy, R., Munn, A., Farney, K., and DiRuggiero, J. (2020). Regulatory Noncoding Small RNAs Are Diverse and Abundant in an Extremophilic Microbial Community. mSystems. 5, e00584. doi:10.1128/mSystems.00584-19
Georg, J., Lalaouna, D., Hou, S., Lott, S. C., Caldelari, I., Marzi, S., et al. (2019). The Power of Cooperation: Experimental and Computational Approaches in the Functional Characterization of Bacterial sRNAs. Mol. Microbiol. 113 (3), 603–612. doi:10.1111/mmi.14420
Giangrossi, M., Prosseda, G., Tran, C. N., Brandi, A., Colonna, B., and Falconi, M. (2010). A Novel Antisense RNA Regulates at Transcriptional Level the Virulence Gene icsA of Shigella Flexneri. Nucleic Acids Res. 38 (10), 3362–3375. doi:10.1093/nar/gkq025
Giannopoulos, G., Sullivan, M. J., Hartop, K. R., Rowley, G., Gates, A. J., Watmough, N. J., et al. (2017). Tuning the modularParacoccus Denitrificansrespirome to Adapt from Aerobic Respiration to Anaerobic Denitrification. Environ. Microbiol. 19, 4953–4964. doi:10.1111/1462-2920.13974
Gierga, G., Voss, B., and Hess, W. R. (2012). Non-coding RNAs in Marine Synechococcus and Their Regulation under Environmentally Relevant Stress Conditions. ISME. J. 6 (8), 1544–1557. doi:10.1038/ismej.2011.215
Gottesman, S. (2005). Micros for Microbes: Non-coding Regulatory RNAs in Bacteria. Trends Genet. 21 (7), 399–404. doi:10.1016/j.tig.2005.05.008
Guerrero, M. G., Vega, J. M., and Losada, M. (1981). The Assimilatory Nitrate-Reducing System and its Regulation. Annu. Rev. Plant Physiol. 32, 169–204. doi:10.1146/annurev.pp.32.060181.001125
Halbleib, C. M., and Ludden, P. W. (2000). Regulation of Biological Nitrogen Fixation. J. Nutr. 130 (5), 1081–1084. doi:10.1093/jn/130.5.1081
Hallin, S., Philippot, L., Löffler, F. E., Sanford, R. A., and Jones, C. M. (2018). Genomics and Ecology of Novel N2O-Reducing Microorganisms. Trends Microbiol. 26, 43–55. doi:10.1016/j.tim.2017.07.003
Herrero, A., Muro-pastor, A. M., and Flores, E. (2001). Nitrogen Control in Cyanobacteria. J. Bacteriol. 183 (2), 411–425. doi:10.1128/jb.183.2.411-425.2001
Hoch, G. E., Schneider, K. C., and Burris, R. H. (1960). Hydrogen Evolution and Exchange, and Conversion of N2O to N2 by Soybean Root Nodules. Biochim. Biophys. Acta. 37 (2), 273–279. doi:10.1016/0006-3002(60)90234-1
Houserova, D. M., Dahmer, D. J., Amin, S. V., King, V. M., Barnhill, E. C., Huang, Y., et al. (2021). Characterization of 475 Novel, Putative Small RNAs (sRNAs) in Carbon-Starved Salmonella enterica Serovar Typhimurium. Biorxiv. Preprint. 10, 305. doi:10.1101/2021.01.11.426214
Hu, J., and Wang, Q. (2018). Regulatory sRNAs in Cyanobacteria. Front. Microbiol. 9, 2399. doi:10.3389/fmicb.2018.02399
Ionescu, D., Voss, B., Oren, A., Hess, W. R., and Muro-Pastor, A. M. (2010). Heterocyst-Specific Transcription of NsiR1, a Non-coding RNA Encoded in a Tandem Array of Direct Repeats in Cyanobacteria. J. Mol. Biol. 398 (2), 177–188. doi:10.1016/j.jmb.2010.03.010
Jäger, D., Sharma, C. M., Thomsen, J., Ehlers, C., Vogel, J., and Schmitz, R. A. (2009). Deep Sequencing Analysis of the Methanosarcina Mazei Go1 Transcriptome in Response to Nitrogen Availability. Proc. Natl. Acad. Sci. 106 (51), 21878–21882. doi:10.1073/pnas.0909051106
Jäger, D., Pernitzsch, S. R., Richter, A. S., Backofen, R., Sharma, C. M., and Schmitz, R. A. (2012). An Archaeal sRNA Targeting Cis - and Trans -encoded mRNAs via Two Distinct Domains. Nucleic Acids Res. 40 (21), 10964–10979. doi:10.1093/nar/gks847
Jensen, F. B. (2003). Nitrite Disrupts Multiple Physiological Functions in Aquatic Animals. Comp. Biochem. Physiol. A: Mol. Integr. Physiol. 135, 9–24. doi:10.1016/s1095-6433(02)00323-9
Jones, C. M., Graf, D. R., Bru, D., Philippot, L., and Hallin, S. (2013). The Unaccounted yet Abundant Nitrous Oxide-Reducing Microbial Community: a Potential Nitrous Oxide Sink. Isme J. 7, 417–426. doi:10.1038/ismej.2012.125
Jung, Y.-S., and Kwon, Y.-M. (2008). Small RNA ArrF Regulates the Expression of sodB and feSII Genes in Azotobacter vinelandiisodB and feSII Genes in Azotobacter Vinelandii. Curr. Microbiol. 57, 593–597. doi:10.1007/s00284-008-9248-z
Kapoor, V., Li, X., Elk, M., Chandran, K., Impellitteri, C. A., and Santo Domingo, J. W. (2015). Impact of Heavy Metals on Transcriptional and Physiological Activity of Nitrifying Bacteria. Environ. Sci. Technol. 49, 13454–13462. doi:10.1021/acs.est.5b02748
Kettles, N. L., Kopriva, S., and Malin, G. (2014). Insights into the Regulation of DMSP Synthesis in the Diatom Thalassiosira Pseudonana through APR Activity, Proteomics and Gene Expression Analyses on Cells Acclimating to Changes in Salinity, Light and Nitrogen. PLoS One. 9, e94795. doi:10.1371/journal.pone.0094795
Kilic, T., Sanglier, S., Van Dorsselaer, A., and Suck, D. (2006). Oligomerization Behavior of the Archaeal Sm2-type Protein fromArchaeoglobus Fulgidus. Protein Sci. 15 (10), 2310–2317. doi:10.1110/ps.06219150910.1110/ps.062191506
Klähn, S., Schaal, C., Georg, J., Baumgartner, D., Knippen, G., Hagemann, M., et al. (2015). The sRNA NsiR4 Is Involved in Nitrogen Assimilation Control in Cyanobacteria by Targeting Glutamine Synthetase Inactivating Factor IF7. Proc. Natl. Acad. Sci. USA. 112 (45), E6243–E6252. doi:10.1073/pnas.1508412112
Kopf, M., and Hess, W. R. (2015). Regulatory RNAs in Photosynthetic Cyanobacteria. FEMS. Microbiol. Rev. 39 (3), 301–315. doi:10.1093/femsre/fuv017
Krätzer, C., Carini, P., Hovey, R., and Deppenmeier, U. (2009). Transcriptional Profiling of Methyltransferase Genes during Growth of Methanosarcina Mazei on Trimethylamine. J. Bacteriol. 191, 5108–5115. doi:10.1128/JB.00420-09
Kumar, K., Chakraborty, A., and Chakrabarti, S. (2020). PresRAT: A Server for Identification of Bacterial Small-RNA Sequences and Their Targets with Probable Binding Region. RNA Biol., 1–8. doi:10.1080/15476286.2020.1836455
Luque-Almagro, V. M., Gates, A. J., Moreno-Vivián, C., Ferguson, S. J., Richardson, D. J., and Roldán, M. D. (2011). Bacterial Nitrate Assimilation: Gene Distribution and Regulation. Biochem. Soc. Trans. 39 (6), 1838–1843. doi:10.1042/BST20110688
Luque-Almagro, V. M., Manso, I., Sullivan, M. J., Rowley, G., Ferguson, S. J., Moreno-Vivián, C., et al. (2017). Transcriptional and Translational Adaptation to Aerobic Nitrate Anabolism in the Denitrifier Paracoccus Denitrificans. Biochem. J. 474 (11), 1769–1787. doi:10.1042/BCJ20170115
Lycus, P., Soriano-Laguna, M. J., Kjos, M., Richardson, D. J., Gates, A. J., Milligan, D. A., et al. (2018). A Bet-Hedging Strategy for Denitrifying Bacteria Curtails Their Release of N2O. Proc. Natl. Acad. Sci. USA. 115 (46), 11820–11825. doi:10.1073/pnas.1805000115
Märtens, B., Sharma, K., Urlaub, H., and Bläsi, U. (2017). The SmAP2 RNA Binding Motif in the 3′UTR Affects mRNA Stability in the Crenarchaeum Sulfolobus solfataricus. Nucleid Acids Res. 45 (15), 8957–8967. doi:10.1093/nar/gkx581
Madsen, E. L. (2011). Microorganisms and Their Roles in Fundamental Biogeochemical Cycles. Curr. Opin. Biotechnol. 22 (3), 456–464. doi:10.1016/j.copbio.2011.01.008
Märtens, B., Bezerra, G., Kreuter, M., Grishkovskaya, I., Manica, A., Arkhipova, V., et al. (2015). The Heptameric SmAP1 and SmAP2 Proteins of the Crenarchaeon Sulfolobus Solfataricus Bind to Common and Distinct RNA Targets. Life (Basel). 5, 1264–1281. doi:10.3390/life5021264
Martinez-Argudo, I., Little, R., Shearer, N., Johnson, P., and Dixon, R. (2004). The NifL-NifA System: a Multidomain Transcriptional Regulatory Complex that Integrates Environmental Signals. J Bacteriol. 186, 601–610. doi:10.1128/jb.186.3.601-610.2004
Masse, E., and Gottesman, S. (2002). A Small RNA Regulates the Expression of Genes Involved in Iron Metabolism in Escherichia coli. Proc. Natl. Acad. Sci. 99 (7), 4620–4625. doi:10.1073/pnas.032066599
McCarren, J., Becker, J. W., Repeta, D. J., Shi, Y., Young, C. R., Malmstrom, R. R., et al. (2010). Microbial Community Transcriptomes Reveal Microbes and Metabolic Pathways Associated with Dissolved Organic Matter Turnover in the Sea. Proc. Natl. Acad. Sci. 107 (38), 16420–16427. doi:10.1073/pnas.1010732107
McCullen, C. A., Benhammou, J. N., Majdalani, N., and Gottesman, S. (2010). Mechanism of Positive Regulation by DsrA and RprA Small Noncoding RNAs: Pairing Increases Translation and Protects rpoS mRNA from Degradation. J Bacteriol. 192 (21), 5559–5571. doi:10.1128/JB.00464-10
Michaux, C., Verneuil, N., Hartke, A., and Giard, J.-C. (2014). Physiological Roles of Small RNA Molecules. Microbiol. 160 (6), 1007–1019. doi:10.1099/mic.0.076208-0
Mjelle, R., Aass, K., Sjursen, W., Hofsli, E., and Saetrom, P. (2020). sMETAseq: Combined Profiling of Microbiota and Host Small RNAs. iScience. 23, 5. doi:10.1016/j.sci.2020.10113110.1016/j.isci.2020.101131
Morfeldt, E., Taylor, D., von Gabain, A., and Arvidson, S. (1995). Activation of Alpha-Toxin Translation in Staphylococcus aureus by the Trans-encoded Antisense RNA, RNAIII. EMBO J. 14, 4569–4577. doi:10.1002/j.1460-2075.1995.tb00136.x
Morita, T., Maki, K., and Aiba, H. (2005). RNase E-Based Ribonucleoprotein Complexes: Mechanical Basis of mRNA Destabilization Mediated by Bacterial Noncoding RNAs. Genes Development. 19, 2176–2186. doi:10.1101/gad.1330405
Morita, T., Mochizuki, Y., and Aiba, H. (2006). Translational Repression Is Sufficient for Gene Silencing by Bacterial Small Noncoding RNAs in the Absence of mRNA Destruction. Proc. Natl. Acad. Sci. 103 (13), 4858–4863. doi:10.1073/pnas.0509638103
Muro-Pastor, M. I., Reyes, J. C., and Florencio, F. J. (2005). Ammonium Assimilation in Cyanobacteria. Photosynth. Res. 83, 135–150. doi:10.1007/s1120-004-2082-710.1007/s11120-004-2082-7
Neupane, D. P., Jacquez, B., Sundararajan, A., Ramaraj, T., Schilkey, F. D., and Yukl, E. T. (2017). Zinc-dependent Transcriptional Regulation in Paracoccus Denitrificans. Front. Microbiol. 8, 569. doi:10.3389/fmicb.2017.00569
Nielsen, J. S., Boggild, A., Andersen, C. B. F., Nielsen, G., Boysen, A., Brodersen, D. E., et al. (2007). An Hfq-like Protein in Archaea: Crystal Structure and Functional Characterization of the Sm Protein from Methanococcus Jannaschii. RNA. 13, 2213–2223. doi:10.1261/rna.689007
Norton, J., and Ouyang, Y. (2019). Controls and Adaptive Management of Nitrification in Agricultural Soils. Front. Microbiol. 10. doi:10.3389/fmicb.2019.01931
Norton, J. M., and Stark, J. M. (2011). Regulation and Measurement of Nitrification in Terrestrial Systems. Methods Enzymol. 486, 343–368. doi:10.1016/B978-0-12-381294-0.00015-8
Novick, R. P., and Geisinger, E. (2008). Quorum sensing in Staphylococci. Annu. Rev. Genet. 42, 541–564. doi:10.1146/annurev.genet.42.110807.091640
Olaya-Abril, A., Luque-Almagro, V. M., Pérez, M. D., López, C. M., Amil, F., Cabello, P., et al. (2019). Putative Small RNAs Controlling Detoxification of Industrial Cyanide-Containing Wastewaters by Pseudomonas pseudoalcaligenes CECT5344. Plos. One. 14 (2), e0212032. doi:10.1371/journal.pone.0212032
Oleijniczak, M., and Storz, G. (2017). ProQ/FinO-domain Protein: Another Ubiquitous Family of RNA Matchmakers?. Mol. Microbiol. 104 (6), 905–915. doi:10.1111/mmi.13679
Otaka, H., Ishikawa, H., Morita, T., and Aiba, H. (2011). PolyU Tail of Rho-independent Terminator of Bacterial Small RNAs Is Essential for Hfq Action. Proc. Natl. Acad. Sci. USA. 108, 13059–13064. doi:10.1073/pnas.1107050108
Papenfort, K., and Vanderpool, C. K. (2015). Target Activation by Regulatory RNAs in Bacteria. FEMS Microbiol. Rev. 39, 362–378. doi:10.1093/femsre/fuv016
Papenfort, K., Sun, Y., Miyakoshi, M., Vanderpool, C. K., and Vogel, J. (2013). Small RNA-Mediated Activation of Sugar Phosphatase mRNA Regulates Glucose Homeostasis. Cell. 153, 426–437. doi:10.1016/j.cell.2013.03.003
Pauleta, S. R., and Moura, I. (2017). Assembly of CuZ and CuA in Nitrous Oxide Reductase. Encycl Inorg. Bioinorg Chem., 1–11. doi:10.1002/9781119951438.eibc2477
Pawlowsky, K., Klosse, U., and the Brujin, F. (1991). Characterization of a Novel Azorhizobium Caulinodans ORS571 Two-Component Regulatory System, NtrY/NtrX, Involved in Nitrogen Fixation Metabolism. Mol. Gen. Genet. 231 (1), 124–138. doi:10.1007/BF00293830
Payá, G., Bautista, V., Camacho, M., Castejón-Fernández, N., Alcaraz, L., Bonete, M.-J., et al. (2018). Small RNAs of Haloferax Mediterranei: Identification and Potential Involvement in Nitrogen Metabolism. Genes. 9 (2), 83. doi:10.3390/genes9020083
Payá, G., Bautista, V., Camacho, M., Bonete, M.-J., and Esclapez, J. (2020). New Proposal of Nitrogen Metabolism Regulation by Small RNAs in the Extreme Halophilic Archaeon Haloferax Mediterranei. Mol. Genet. Genomics. 295 (3), 775–785. doi:10.1007/s00438-020-01659-9
Pei, G., Sun, T., Chen, S., Chen, L., and Zhang, W. (2017). Systematic and Functional Identification of Small Non-coding RNAs Associated with Exogenous Biofuel Stress in Cyanobacterium Synechocystis Sp. PCC 6803. Biotechnol. Biofuels. 10, 57. doi:10.1186/s13068-017-0743-y
Peterson, J. R., Labhsetwar, P., Ellermeier, J. R., Kohler, P. R. A., Jain, A., Ha, T., et al. (2014). Towards a Computational Model of a Methane Producing Archaeum. Archaea. 2014, 1–18. doi:10.1155/2014/898453
Philippot, L., Piutti, S., Martin-Laurent, F., Hallet, S., and Germon, J. C. (2002). Molecular Analysis of the Nitrate-Reducing Community from Unplanted and Maize-Planted Soils. Appl Environ Microbiol. 68 (12), 6121–6128. doi:10.1128/aem.68.12.6121-6128.2002
Placella, S. A., and Firestone, M. K. (2013). Transcriptional Response of Nitrifying Communities to Wetting of Dry Soil. Appl. Environ. Microbiol. 79 (10), 3294–3302. doi:10.1128/AEM.00404-13
Prasse, D., and Schmitz, R. A. (2018). Small RNAs Involved in Regulation of Nitrogen Metabolism. Microbiol. Spectr. 6, 4. doi:10.1128/microbiolspec.RWR-0018-2018
Prasse, D., Förstner, K. U., Jäger, D., Backofen, R., and Schmitz, R. A. (2017). sRNA154 a Newly Identified Regulator of Nitrogen Fixation in Methanosarcina Mazei Strain Gö1. RNA Biol. 14 (11), 1544–1558. doi:10.1080/15476286.2017.1306170
Prinn, R. G., Weiss, R. F., Arduini, J., Arnold, T., DeWitt, H. L., Fraser, P. J., et al. (2018). History of Chemically and Radiatively Important Atmospheric Gases from the Advanced Global Atmospheric Gases Experiment (AGAGE). Earth Syst. Sci. Data 10, 985–1018. doi:10.5194/essd-10-985-2018
Prosser, J. I. (2007). The Ecology of Nitrifying Bacteria. Biol. nitrogen Cycle, 223–243. doi:10.1016/B978-04445285710.1016/b978-044452857-5.50016-3
Rajendran, K., Kumar, V., Raja, I., Kumariah, M., and Tennyson, J. (2020). Identification of Small Non-coding RNAs from Rhizobium Etli by Integrated Genome-wide and Transcriptome-Based Methods. ExRNA. 2, 14. doi:10.1186/s41544-020-00054-1
Reisch, C. R., Crabb, W. M., Gifford, S. M., Teng, Q., Stoudemayer, M. J., Moran, M. A., et al. (2013). Metabolism of Dimethylsulphoniopropionate byRuegeria Pomeroyi DSS-3. Mol. Microbiol. 89, 774–791. doi:10.1111/mmi.12314
Renseigne, N., Umar, S., and Iqbal, M. (2007). Nitrate Accumulation in Plants, Factors Affecting the Process, and Human Health Implication. A. Review. Agron. Sustain. Dev. Springer/edp Sciences/inra. 27 (1), 45–57. doi:10.1051/agro:2006021
Richardson, D., Felgate, H., Watmough, N., Thomson, A., and Baggs, E. (2009). Mitigating Release of the Potent Greenhouse Gas N2O from the Nitrogen Cycle - Could Enzymic Regulation Hold the Key?. Trends Biotechnol. 27 (7), 388–397. doi:10.1016/j.tibtech.2009.03.009
Richardson, D. J., Edwards, M. J., White, G. F., Baiden, N., Hartshorne, R. S., Fredrickson, J., et al. (2012). Exploring the Biochemistry at the Extracellular Redox Frontier of Bacterial Mineral Fe(III) Respiration. Biochem. Soc. Trans. 40, 493–500. doi:10.1042/BST20120018
Rivers, A. R., Burns, A. S., Chan, L.-K., and Moran, M. A. (2016). Experimental Identification of Small Non-coding RNAs in the Model Marine Bacterium Ruegeria Pomeroyi DSS-3. Front. Microbiol. 7 (7), 380. doi:10.3389/fmicb.2016.00380
Robertson, G. P., and Vitousek, P. M. (2009). Nitrogen in Agriculture: Balancing the Cost of an Essential Resource. Annu. Rev. Environ. Resour. 34 (1), 97–125. doi:10.1146/annurev.environ.032108.105046
Romeo, A., Sonnleitner, E., Sorger-Domenigg, T., Nakano, M., Eisenhaber, B., and Bläsi, U. (2012). Transcriptional Regulation of Nitrate Assimilation in Pseudomonas aeruginosa Occurs via Transcriptional Antitermination within the nirBD-PA1779-cobA Operon. Microbiol. 158, 1543–1552. doi:10.1099/mic.0.053850-0
Rowley, G., Hensen, D., Felgate, H., Arkenberg, A., Appia-Ayme, C., Prior, K., et al. (2012). Resolving the Contributions of the Membrane-Bound and Periplasmic Nitrate Reductase Systems to Nitric Oxide and Nitrous Oxide Production in Salmonella enterica Serovar Typhimurium. Biochem. J. 441, 755–762. doi:10.1042/BJ20110971
Sánchez, C., Itakura, M., Okubo, T., Matsumoto, T., Yoshikawa, H., Gotoh, A., et al. (2014). The Nitrate-Sensing NasST System Regulates Nitrous Oxide Reductase and Periplasmic Nitrate Reductase inBradyrhizobium Japonicum. Environ. Microbiol. 16, 3263–3274. doi:10.1111/1462-2920.12546
Saggar, S., Jha, N., Deslippe, J., Bolan, N. S., Luo, J., Giltrap, D. L., et al. (2013). Denitrification and N2O:N2 Production in Temperate Grasslands: Processes, Measurements, Modelling and Mitigating Negative Impacts. Sci. Total Environ. 465, 173–195. doi:10.1016/j.scitotenv.2012.11.050
Sanders, D. A., Gillece-Castro, B. L., Burlingame, A. L., and Koshland, D. E. (1992). Phosphorylation Site of NtrC, a Protein Phosphatase Whose Covalent Intermediate Activates Transcription. J. Bacteriol. 174, 5117–5122. doi:10.1128/jb.174.15.5117-5122.1992
Sauter, C., Basquin, J., and Suck, D. (2003). Sm-like Proteins in Eubacteria: the Crystal Structure of the Hfq Protein from Escherichia coli. Nucleic Acids Res. 31, 4091–4098. doi:10.1093/nar/gkg480
Schreiber, K., Krieger, R., Benkert, B., Eschbach, M., Eschbach, M., Arai, H., et al. (2007). The Anaerobic Regulatory Network Required for Pseudomonas aeruginosa Nitrate Respiration. J Bacteriol. 189 (11), 4310–4314. doi:10.1128/JB.00240-07
Sedlyarova, N., Shamovsky, I., Bharati, B. K., Epshtein, V., Chen, J., Gottesman, S., et al. (2016). sRNA-mediated Control of Transcription Termination in E. coli. Cell. 167, 111–121. doi:10.1016/j.cell.2016.09.004
Sharma, C. M., Darfeuille, F., Plantinga, T. H., and Vogel, J. (2007). A Small RNA Regulates Multiple ABC Transporter mRNAs by Targeting C/A-rich Elements inside and Upstream of Ribosome-Binding Sites. Genes Development. 21 (21), 2804–2817. doi:10.1101/gad.447207
Shi, Y., Tyson, G. W., and DeLong, E. F. (2009). Metatranscriptomics Reveals Unique Microbial Small RNAs in the Ocean's Water Column. Nature. 459, 266–269. doi:10.1038/nature08055
Sinclair, A. H., and Edwards, A. C. (2008). Micronutrient Deficiency Problems in Agricultural Crops in Europe. Micronutrient Deficiencies in Global Crop Production. Dordrecht: Springer, 225–244. doi:10.1007/978-1-4020-6860-7_9
Smil, V. (2002). Nitrogen and Food Production: Proteins for Human Diets. AMBIO: A J. Hum. Environ. 31, 126–131. doi:10.1579/0044-7447-31.2.126
Smirnov, A., Förstner, K. U., Holmqvist, E., Otto, A., Günster, R., Becher, D., et al. (2016). Grad-seq Guides the Discovery of ProQ as a Major Small RNA-Binding Protein. Proc. Natl. Acad. Sci. USA. 113, 11591–11596. doi:10.1073/pnas.1609981113
Smirnov, A., Wang, C., Drewry, L. L., and Vogel, J. (2017). Molecular Mechanism of mRNA Repression in Trans by a ProQ‐dependent Small RNA. EMBO. J. 36, 1029–1045. doi:10.15252/embj.201696127
Smith, K. A., Mosier, A. R., Crutzen, P. J., and Winiwarter, W. (2012). The Role of N 2 O Derived from Crop-Based Biofuels, and from Agriculture in General, in Earth's Climate. Phil. Trans. R. Soc. B. 367, 1169–1174. doi:10.1098/rstb.2011.0313
Sonnleitner, E., Gonzalez, N., Sorger-Domenigg, T., Heeb, S., Richter, A. S., Backofen, R., et al. (2011). The Small RNA PhrS Stimulates Synthesis of the Pseudomonas aeruginosa Quinolone Signal. Mol. Micobiol. 80, 868–885. doi:10.1111/j.1365-2958.2011.07620.x
Sridhar, J., and Gunasekaran, P. (2013). Computational Small RNA Prediction in Bacteria. Bioinform. Biol. Insights. 7, BBI.S11213–95. doi:10.4137/BBI.S11213
Stocker, T., Qin, D., Plattner, G., Tignor, M., Allen, S., Boschung, J., et al. (2013). IPCC (2013) Climate Change 2013: The Physical Science Basis. Contribution of Working Group I to the Fifth Assessment Report of the Intergovernmental Panel on Climate Change. Cambridge, United Kingdom and New York. NY. USA: Cambridge University Press, 1535
Storz, G., Opdyke, J. A., and Zhang, A. (2004). Controlling mRNA Stability and Translation with Small, Noncoding RNAs. Curr. Opin. Microbiol. 7 (2), 140–144. doi:10.1016/j.mib.2004.02.015
Storz, G., Vogel, J., and Wassarman, K. M. (2011). Regulation by Small RNAs in Bacteria: Expanding Frontiers. Mol. Cel 43 (6), 880–891. doi:10.1016/j.molcel.2011.08.022
Stremińska, M. A., Felgate, H., Rowley, G., Richardson, D. J., and Baggs, E. M. (2012). Nitrous Oxide Production in Soil Isolates of Nitrate-Ammonifying Bacteria. Environ. Microbiol. Rep. 4, 66–71. doi:10.1111/j.1758-2229.2011.00302.x
Sullivan, M. J., Gates, A. J., Appia-Ayme, C., Rowley, G., and Richardson, D. J. (2013). Copper Control of Bacterial Nitrous Oxide Emission and its Impact on Vitamin B12-dependent Metabolism. Proc. Natl. Acad. Sci. 110 (49), 19926–19931. doi:10.1073/pnas.1314529110
Sutton, M., Howard, C., Erisman, J., Billen, G., Bleeker, A., van Grinsven, H., et al. (2011). The European Nitrogen Assessment: Sources, Effects and Policy Perspectives. Cambridge: Cambridge University Press. ISBN 9781107006126
Tarallo, S., Ferrero, G., Gallo, G., Francavilla, A., and Clerico, G. (2019). Altered Fecal Small RNA Profiles in Colorectal Cancer Reflect Gut Microbiome Composition in Stool Samples. mSystems. 4 (5), e00289–19. doi:10.1128/mSystems.00289-19
Tata, M., Amman, F., Pawar, V., Wolfinger, M. T., Weiss, S., Häussler, S., et al. (2017). The Anaerobically Induced sRNA PaiI Affects Denitrification in Pseudomonas aeruginosa PA14. Front. Microbiol. 8, 2312. doi:10.3389/fmicb.2017.02312
Teikari, J., Österholm, J., Kopf, M., Battchikova, N., Wahlsten, M., Aro, E.-M., et al. (2015). Transcriptomic and Proteomic Profiling of Anabaena Sp. Strain 90 under Inorganic Phosphorus Stress. Appl. Environ. Microbiol. 81 (15), 5212–5222. doi:10.1128/AEM.01062-15
Thore, S., Mayer, C., Sauter, C., Weeks, S., and Suck, D. (2003). Crystal Structures of the Pyrococcus Abyssi Sm Core and its Complex with RNA. J. Biol. Chem. 278, 1239–1247. doi:10.1074/jbc.M207685200
Törö, I., Thore, S., Mayer, C., Basquin, J., Séraphin, B., and Suck, D. (2001). RNA Binding in an Sm Core Domain: X-Ray Structure and Functional Analysis of an Archaeal Sm Protein Complex. EMBO. J. 20, 2293–2303. doi:10.1093/emboj/20.9.2293
Törö, I., Basquin, J., Teo-Dreher, H., and Suck, D. (2002). Archaeal Sm Proteins Form Heptameric and Hexameric Complexes: Crystal Structures of the Sm1 and Sm2 Proteins from the Hyperthermophile Archaeoglobus Fulgidus. J. Mol. Biol. 320, 129–142. doi:10.1016/S0022-2836(02)00406-0
Torres, M. J., Bueno, E., Jiménez-Leiva, A., Cabrera, J. J., Bedmar, E. J., Mesa, S., et al. (2017). FixK2 Is the Main Transcriptional Activator of Bradyrhizobium Diazoefficiens nosRZDYFLX Genes in Response to Low Oxygen. Front. Microbiol. 8, 1621. doi:10.3389/fmicb.2017.01621
Tosques, I. E., Shi, J., and Shapleigh, J. P. (1996). Cloning and Characterization of nnrR, Whose Product Is Required for the Expression of Proteins Involved in Nitric Oxide Metabolism in Rhodobacter Sphaeroides 2.4.3. J. Bacteriol. 178, 4958–4964. doi:10.1128/jb.178.16.4958-4964.1996
Udekwu, K. I., Darfeuille, F., Vogel, J., Reimegard, J., Holmqvist, E., and Wagner, E. (2005). Hfq-dependent Regulation of OmpA Synthesis Is Mediated by an Antisense RNA. Genes Development 19 (19), 2355–2366. doi:10.1101/gad.354405
Van Maanen, J. M., Welle, I. J., Hageman, G., Dallinga, J. W., Mertens, P. L., and Kleinjans, J. C. (1996). Nitrate Contamination of Drinking Water: Relationship with HPRT Variant Frequency in Lymphocyte DNA and Urinary Excretion of N-Nitrosamines. Environ. Health Perspect. 104, 522–528. doi:10.1289/ehp.96104522
Van Spanning, R. J. M., De Boer, A. P. N., Reijnders, W. N. M., Spiro, S., Westerhoff, H. V., Stouthamer, A. H., et al. (1995). Nitrite and Nitric Oxide Reduction in Paracoccus Denitrificans Is under the Control of NNR, a Regulatory Protein that Belongs to the FNR Family of Transcriptional Activators. FEBS. Lett. 360 (2), 151–154. doi:10.1016/0014-5793(95)00091-m
Vanderpool, C., and Gottesman, S. (2007). A Dual Function for a Bacterial Small RNA: SgrS Performs Base Pairing-dependent Regulation and Encodes a Functional Polypeptide. PNAS. 104 (51), 20454–20459. doi:10.1073/pnas.0708102104
Vanderpool, C. K., and Gottesman, S. (2004). Involvement of a Novel Transcriptional Activator and Small RNA in Post-transcriptional Regulation of the Glucose Phosphoenolpyruvate Phosphotransferase System. Mol.r Microbiol. 54 (4), 1076–1089. doi:10.1111/j.1365-2958.2004.04348.x
Veit, K., Ehlers, C., and Schmitz, R. A. (2005). Effects of Nitrogen and Carbon Sources on Transcription of Soluble Methyltransferases in Methanosarcina Mazei Strain Gö1. J Bacteriol. 187, 6147–6154. doi:10.1128/JB.187.17.6147-6154.2005
Vitousek, P., and Howarth, R. (1991). Nitrogen Limitation on Land and in the Sea: How Can it Occur?. Biogeochemistry. 13, 87–115. doi:10.1007/BF00002772
Vogel, J., and Luisi, B. F. (2011). Hfq and its Constellation of RNA. Nat. Rev. Microbiol. 9, 578–589. doi:10.1038/nrmicro2615
Vogel, J. (2020). An RNA Biology Perspective on Species‐specific Programmable RNA Antibiotics. Mol. Microbiol. 113 (3), 550–559. doi:10.1111/mmi.14476
Vollack, K.-U., Härtig, E., Körner, H., and Zumft, W. G. (1999). Multiple Transcription Factors of the FNR Family in Denitrifying Pseudomonas Stutzeri : Characterization of Four Fnr-like Genes, Regulatory Responses and Cognate Metabolic Processes. Mol. Microbiol. 31 (6), 1681–1694. doi:10.1046/j.1365-2958.1999.01302.x
Walker, C. B., de la Torre, J. R., Klotz, M. G., Urakawa, H., Pinel, N., Arp, D. J., et al. (2010). Nitrosopumilus Maritimus Genome Reveals Unique Mechanisms for Nitrification and Autotrophy in Globally Distributed Marine Crenarchaea. Proc. Natl. Acad. Sci. 107 (19), 8818–8823. doi:10.1073/pnas.0913533107
Wassarman, K. M., and Storz, G. (2000). 6S RNA Regulates E. coli RNA Polymerase Activity. Cell. 101, 613–623. doi:10.1016/s0092-8674(00)80873-9
Wassarman, K. M. (2002). Small RNAs in Bacteria. Cell. 109 (2), 141–144. doi:10.1016/S0092-8674(02)00717-1
Waters, L. S., and Storz, G. (2009). Regulatory RNAs in Bacteria. Cell. 136 (4), 615–628. doi:10.1016/j.cell.2009.01.043
Weidenbach, K., Ehlers, C., Kock, J., Ehrenreich, A., and Schmitz, R. A. (2008). Insights into the NrpR Regulon in Methanosarcina Mazei Gö1. Arch. Microbiol. 190, 319–332. doi:10.1007/s00203-008-0369-3
Weidenbach, K., Ehlers, C., Kock, J., and Schmitz, R. A. (2010). NrpRII Mediates Contacts between NrpRI and General Transcription Factors in the Archaeon Methanosarcina Mazei Gö1. FEBS. J. 277, 4398–4411. doi:10.1111/j.1742-4658.2010.07821.x
Westermann, A. J., Venturini, E., Sellin, M. E., Förstner, K. U., Hardt, W.-D., and Vogel, J. (2019). The Major RNA-Binding Protein ProQ Impacts Virulence Gene Expression inSalmonella entericaSerovar Typhimurium. mBIO. 10 (1), e02504–18. doi:10.1128/mBio.02504-18
Williams, B. T., and Todd, J. D. (2019). A Day in the Life of Marine Sulfonates. Nat. Microbiol. 4 (10), 1610–1611. doi:10.1038/s41564-019-0576-5
Zhan, Y., Yan, Y., Deng, Z., Chen, M., Lu, W., Lu, C., et al. (2016). The Novel Regulatory ncRNA, NfiS, Optimizes Nitrogen Fixation via Base Pairing with the Nitrogenase Gene nifK mRNA in Pseudomonas Stutzeri A1501. Proc. Natl. Acad. Sci. USA. 113 (30), E4348–E4356. doi:10.1073/pnas.1604514113
Zumft, W. G., and Kroneck, P. M. (2007). Respiratory Transformation of Nitrous Oxide (N2O) to Dinitrogen by Bacteria and Archaea. Adv. Microb. Physiol. 52, 107–227. doi:10.1016/S0065-2911(06)52003-X
Keywords: denitrification, biogeochemical cycles & processes, Paracoccus denitrificans, sRNA, nitrous oxide, nitrogen cycle
Citation: Moeller S, Payá G, Bonete M-J, Gates AJ, Richardson DJ, Esclapez J and Rowley G (2021) Microbial Small RNAs – The Missing Link in the Nitrogen Cycle?. Front. Environ. Sci. 9:660055. doi: 10.3389/fenvs.2021.660055
Received: 28 January 2021; Accepted: 28 April 2021;
Published: 17 May 2021.
Edited by:
Yupeng Wu, Huazhong Agricultural University, ChinaReviewed by:
Patrick Sorensen, Lawrence Berkeley National Laboratory, United StatesBritt Dianne Hall, University of Regina, Canada
Copyright © 2021 Moeller, Payá, Bonete, Gates, Richardson, Esclapez and Rowley. This is an open-access article distributed under the terms of the Creative Commons Attribution License (CC BY). The use, distribution or reproduction in other forums is permitted, provided the original author(s) and the copyright owner(s) are credited and that the original publication in this journal is cited, in accordance with accepted academic practice. No use, distribution or reproduction is permitted which does not comply with these terms.
*Correspondence: Gary Rowley, g.rowley@uea.ac.uk