Co-Designing Urban Carbon Sink Parks: Case Carbon Lane in Helsinki
- 1Department of Agricultural Sciences, University of Helsinki, Helsinki, Finland
- 2Department of Forest Sciences, University of Helsinki, Helsinki, Finland
- 3School of Business and Economics, School of Resource Wisdom, University of Jyväskylä, Jyväskylä, Finland
- 4Department of Design, Aalto University, Espoo, Finland
In order to achieve the goals of carbon (C) neutrality within next 20 year, municipalities worldwide need to increasingly apply negative emission technologies. We focus on the main principles of urban demonstration areas using biochars for C sequestration and explore the lessons learned from a co-creation process of one such park, Hyväntoivonpuisto in Helsinki, Finland. Demonstration sites of urban C sinks in public parks must be safe, visible and scientifically sound for reliable and cost-effective verification of carbon sequestration. We find that different interests can be arbitrated and that synergy that emerges from co-creation of urban C sink parks between stakeholders (scientists, city officials, companies, and citizens) can result in demo areas with maximized potential for impact, dissemination and consideration of principles of scientific experimentation.
Introduction
The enhanced drawdown of CO2 from the atmosphere in quantities exceeding 1,000 Gt of CO2 is critical for meeting the targets to control climate warming, even though the reduction of emissions of greenhouse gases (GHGs) needs to be the primary goal (IPCC 2019; Amonette et al., 2021). While the implementation of more sustainable management practices in agriculture and forestry is essential in mitigation of climate change, the importance of urban vegetation and soils have been underestimated (Brown et al., 2012).
Besides being potential carbon sinks and stores, urban vegetation and soils provide other ecosystem services, such as improved stormwater management, recreation of inhabitants and even food production. In general, green built environment and nature-based solutions in cities yield both environmental and social benefits by improving the quality of urban life. Currently, urban areas act as net sources of GHGs (Velasco and Roth 2010) and the trends for urbanization feed further growth of GHG emissions from cities in future. More sustainable practices are needed in cities to reverse this development. Like many other cities, City of Helsinki aims to become carbon neutral by 2035 (City of Helsinki 2018). This ambitious goal is not easily reached. Significant reductions to current emissions are essential, but also negative emissions technologies, such as biochars, will be increasingly important. Biochars are materials rich in C stable for hundreds to thousands of years (Kuzyakov et al., 2014), produced from biomass that would otherwise mineralize in a relatively short time as CO2 to the atmosphere. Also, adding urban trees and canopy cover is effective for C sequestration (e.g., Pataki et al., 2011).
Implementation of C drawdown from atmosphere at large scale demands that the reductions can be quantified by measuring and monitoring accurately and cost-efficiently—this remains challenging (Paustian et al., 2016). Further, in carbon market context, it is important that the emission reductions or CO2 removal are additional to the baseline scenario and the effect of the implemented activity can be reliably estimated.
Demonstration areas provide situated learning opportunities for novel solutions. Yet, if properly planned they can also serve as field trials and provide new knowledge of the principles and suitability of the solutions. Such knowledge is relevant for scientists and for development of new products and services. In addition, demonstrations in urban green areas are visible to public, supporting co-operation and accessibility. The Carbon Lane project brought together actors from different related sectors in Finland (e.g., suppliers of growing media, researchers and policymakers) in a co-creation process including multiple workshops for ideation and knowledge sharing. As part of the project, an urban demonstration site with different biochar-based planting soils and trees was established in Hyväntoivonpuisto, the central park of Jätkäsaari in Helsinki, Finland.
Here we focus on the main principles of urban demonstration areas for C sequestration, particularly those including biochars, and consider the technologies and practices that may be relevant based on scientific soundness and applicability to urban space. We also explore the lessons learned from a co-creation process of one such park.
Policy Options and Implications
Design Principles of Urban Demonstration Sites for Carbon Sequestration
Scientific Soundness
Proper documentation of all practices
We recommend that all materials used on setting up the demonstration site (e.g., biochars, composts, fertilisers) should be sampled representatively prior to adding them in soils, and their quality analysed. The effect of added biochars or other soil amendments can best be predicted from relevant analyses (Bird 2015). Sampling and analysing of biochar should preferably be done according to the guidelines of the European Biochar Certificate (EBC 2012) on-site or alternatively using incremental cross-stream sampling devices in the biochar production unit.
As a part of the carbon sequestration verification process, however, also the persistence of biochars in soil should be assessed. In addition to measurements characterising the fractions of black C (e.g., by benzene polycarboxylic acid (BPCA) technique), the persistence of biochars in soil can also be predicted from cheap and easy-to-measure proxy characteristics like the molar H/Corg ratio of biochars. The amounts of materials added, and their C content needs to be measured and documented because the external C input must be considered as a part of the verification process. The starting points including e.g., tree dimensions and initial C stock estimate and initial soil C content should be measured and documented properly. Fertilisation, irrigation and other maintenance practices affecting plant growth and C sequestration through soil nutrient status and water content, should also be recorded.
Experimental Design
In order to assess the effectiveness of treatments tested (e.g., different biochar-based growing media), it is crucial to plan experimental controls so that the treatment effects clearly stand out. Control units (e.g., trees) should be as identical as possible to the units undergoing experimental manipulation. Controls and treatments are kept under same conditions; for example, similar management like watering the trees is conducted for the controls and treatments. Two different types of controls can be used: negative and positive. Negative controls receive manipulation that is expected to have no effect, often using Business-as-Usual is appropriate. Positive control in an experiment is a treatment which is expected to produce expected results and can be used to show that the experimental procedure is working- for example, using unpyrolized wood chips for providing the same amount of C as in biochar-containing treatments.
To avoid random variation or “noise” due to variations in environment and trees, each treatment needs to be replicated (at least 5–10 test subjects, e.g., trees per treatment) and replicates randomised. All measurements and samplings must be conducted avoiding edge effects. Each treatment should be randomly assigned over the area, while representatively considering shade, hilltops, and valleys as well as the distance from the paved routes.
Validation of Carbon Sequestration in Soil
Methods for estimating carbon sequestration are available from laboratory and field scale to ecosystem and regional level measurements (Nayak et al., 2019; Smith et al., 2020). Generally, the estimation of C stocks and potential C sequestration in urban C parks should be based on a combination of data from the laboratory and field measurements and modelling, rather than just a single measurement method.
To verify that soil organic carbon (SOC) sequestration has taken place with a certain treatment at the site, it is often necessary to be able to show an increase in SOC stock over time (Olson 2013). The main challenges in SOC measurements result from the spatial variability of SOC content in heterogeneous soil matrix and the relatively slow temporal changes in soil C stock.
The choice of the methods for the verification of soil and biochar C sequestration deserves careful consideration. There are wide range of techniques available but none of them is clearly superior (e.g., Hammes et al., 2007; Nayak et al., 2019). It would be beneficial to keep the methods (and if possible also devices) used for C determination the same throughout the monitoring period to ensure the comparability of data acquired at different points of time (Olson 2013). For routine analysis, elemental analyses via dry combustion has been proposed as the most suitable method for the measurement of total C content in soil: the equipment is widely available and the analysis is relatively cheap, but this method by itself does not provide separation of pyrogenic C fractions from other SOC fractions (FAO 2019).
The effects of biochars on native soil C (priming effect) over time are relevant to be considered as well. The priming effects can even have a larger impact on C sequestration potential than the direct effect of biochar addition, and first long-term field studies on the issue are promising (Weng et al., 2017; Blanco-Canqui et al., 2020). Namely, biochar addition has been found to enhance soil aggregation and hence, the retention of root-derived C by 20% in 10-year experiment in Australia (Weng et al., 2017) and in Midwestern United States, a SOC increase by twice the amount of biochar C applied was reported 6 years after biochar application (Blanco-Canqui et al., 2020). Thus, long-term experiments combined with repeated and representative soil sampling (including subsoil) are one way for the verification of biochar and SOC sequestration.
Nevertheless, since biochars degrade relatively slowly, estimating their degradation under the field conditions requires long timescales (Kuzyakov et al., 2014). Biochar particles are likely also eroded or leached (Obia et al., 2017), cases in which they may be lost from the analyses and calculations but not necessarily as CO2 to the atmosphere. Hence knowing the amount and longevity of the applied biochar (even by using proxies as H/Corg ratios) can be seen as even more important than quantifying the amount of biochar over time with sampling.
Measurements of Vegetation
Plant growth over time is a simple measure for the success of planting and carbon sequestration to its standing biomass. Measuring change in plant dry biomass integrates the effects of C sequestration (via photosynthesis) and loss (e.g., respiration, grazing by pests, branch pruning).
Trees allocate the increase in biomass, and thus stored carbon, to plant compartments (such as trunk, fine roots, leaves) with varying longevity. The long-living woody compartments, coarse roots, trunk, and branches, contain the majority of live biomass C. These are more important to measure than short-lived fine roots and leaves which mainly feed the soil carbon pool. Tree biomass equations estimating dry biomass from trunk diameter can be considered a relatively accurate way to non-destructively assess total biomass C sequestration for urban trees (Riikonen et al., 2017; Figure 1A). Trunk diameter in itself is fairly simple to measure, but for repeated measurements the measuring height should be permanently marked on the trunk.
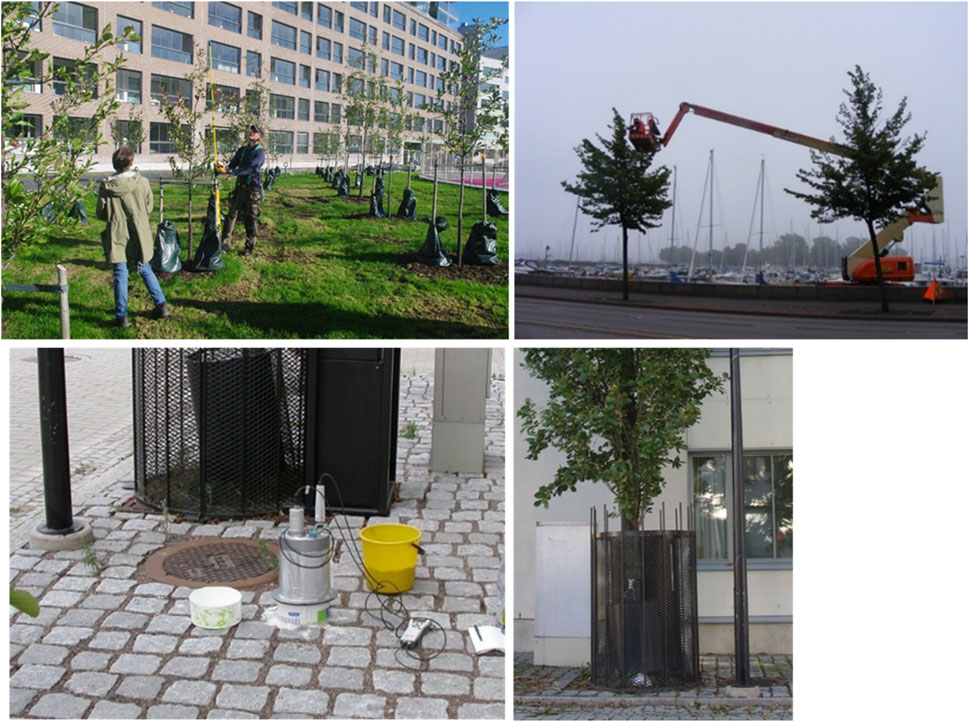
FIGURE 1. (A–D) clockwise]. Measurements of tree dimension from young trees in Hyväntoivonpuisto (A) and demonstration of how complicated can the collection of leaf biomass samples from street trees higher than 2–3 m be (B). Portable chamber system with infrared carbon dioxide analyzer and temperature and relative humidity probe for soil CO2 flux measurement (C). Sensors installed on urban tree trunks can be protected by surrounding the entire tree base in custom made steel mesh cage (D).
If biomass equations are not available for a given taxa or are judged to be unsuitable, the traditional method of measuring plant C stock is based on destructive sampling (Riikonen et al., 2017). This is rarely an option for valuable park trees (Figure 1B). Arguably the best non-destructive method is terrestrial laser scanning (McHale et al., 2009; Tanhuanpää et al., 2017), which gives excellent estimates for tree aboveground biomass volume but requires conversion to mass basis to attain C content.
Regarding assessment of C sequestration via growth of non-woody plants like grasses and forbs, collecting biomass samples is feasible, but on annual scale, non-woody plant biomass C is less significant. In the case of lawns, the goal usually is a good visual appearance (greenness, evenness) and in case of meadow, presence, diversity, and abundance of flowering plants (e.g., Norton et al., 2019) rather than biomass growth.
Ecosystem Level Measurements
The eddy covariance (EC) technique is an established, yet expensive method to measure the exchange of various compounds, such as GHGs but also water vapour, between the atmosphere and land surface. EC measures fluxes on ecosystem scale, integrating over its entire footprint area (typically few thousands of m2). Understanding the processes behind the measured fluxes however require partitioning fluxes to their sources (e.g., Nordbo et al., 2012), thus usually also direct independent measurements of flux components are required.
Various chamber methods, both stationary and portable, are useful to complement EC measurements to establish the flux components (Figure 1C). A chamber is sealed against soil surface or e.g., a leaf, and an analyser for the gases which either are accumulated in the measuring chamber or led to the analyser while replacement air is vented into the chamber. Stationary, automated chambers allow longer-term measurements but are more expensive to establish (require a constant power source and data loggers). Portable chambers operated on batteries offer lower initial costs due to less required infrastructure and can be used in short-term measurements under supervision, requiring more labour.
Applicability to Urban Space
All carbon fixing treatments used in public demonstration sites need to be safe for humans and environment and hard to vandalise. All materials used in urban environment should be traceable and fulfil local safety criteria. As the EBC sets even higher standards for the quality of biochar (EBC 2012) than REACH regulations, only EBC-certified biochars are recommended in the EU. Biochar treatments need to be evaluated and planned considering practical issues like dustiness. For example, no biochar should be visible on the surface as fine-particles may be eroded with wind or water and large particles may tempt people to use it as barbeque or drawing coal. Rather the top 5 cm of soil should be a cover of gravel or rocks.
Any structures or devices installed for research purposes in public green areas should not interfere with site accessibility. Electrical and other hazardous or valuable research apparatus must be installed behind safety screens (Figure 1D). Portable equipment cannot be left unattended, and any soil disturbances caused by sampling must be evened out. Similarly, staff safety must be ensured by wearing reflective clothing, and appropriate training for working in traffic areas should be attended if needed.
Public Awareness
Raising the public awareness of the C sequestration is one of the key objectives of the urban C sink parks. The parks itself, no matter how well planned, will have only a very limited capacity to absorb carbon- thus it is highly important to inspire people to carry out their own actions elsewhere. The means of increasing public awareness are divided into those implemented in an urban demo park and those not bound to the physical park location, such as websites and campaigns using social media influencers (Table 1). We propose that the focus is on communication within the park, as it is more effective to influence on people when they are already at the site.
People gather information with all their senses, so the communication should not be limited to written form. A broad palette of actions should be used, including communicating measurement data with interactive and transforming artworks (Table 2). The artworks of light, sound and movement could react to the changes of the measured variables such as C drawdown, so the visitors could easily follow the changes happening on different natural phenomena.
Actionable Recommendations
Demonstration sites of urban C sequestration methods aid in achieving the goals of carbon (C) neutrality within next 20 year for municipalities. For maximising the impact of such sites and to facilitate validation of C sequestration, they need to be designed in a way that is scientifically sound. Thus, it is crucial to document well all practices and carefully plan the experimental design and follow-up (Scientific Soundness). All carbon fixing treatments need to be applicable to urban space (Applicability to Urban Space). Most importantly, they must be safe for humans and environment. Raising the public awareness of the C sequestration is one of the key objectives of the urban C sink parks to maximise the demonstration effect by inspiring people (Public Awareness).
Regarding the lessons learned from the Carbon Lane case, the main recommendation is to accept that the resources available and the timetables fixed with contractors will likely cause limitations to what extent the general principles outlined in Design Principles of Urban Demonstration Sites for Carbon Sequestration can be realised. For instance, when working with novel growing media, the issues to consider include the limited availability, extensive delivery times and properties differing from product sheet due to active product development.
The first proposal of the Jätkäsaari demonstration site (Figure 2) was designed following closely the principles outlined in 2.1. The proposal included six different biochar containing planting soils +control in grass areas and five different treatments (+control) with trees. After discussions with stakeholders the proposal was amended iteratively. The final plan of the demonstration area was a compromise with limitations, the number of treatments and repetitions was determined by delivery schedules, and also the randomisation principle was compromised on. To facilitate construction, the trees are planted in connected pits with two to seven trees per pits clustered together (Figure 3). All these modifications can be viewed as local learning processes of realising urban demonstration areas on a general level.
Next, it might be relevant to set and communicate well the common criteria to growing media providers regarding e.g., C or nutrient contents or load-bearing properties of the materials- as well as expected delivery time and location in the heavily trafficked urban centre. In our case, the C and nutrient contents of growing media varied remarkably, requiring repeated top-filling and grass re-seeding in some treatments (Figure 1A). Further, the highly varying properties of different growing media could result in challenges when analyzing differences between treatments. For example, prominent variation in nutrient levels might mask effects of biochars on growth. Controlling such effects would be aided if potentially interfering properties could be standardized or by including additional control treatment which equivalents with these properties.
We found also active communication with contractors and stakeholders to be crucial regarding the follow-up study. As typical landscape construction site documentation is not sufficient for needs of research, the expectations related to documentation of construction process need to be communicated in advance and as specifically as possible, especially if researchers are not able to participate on site during key phases of construction as recommended.
Finally, an effort should be made to predict habits of people, e.g., to foresee future shortcut paths across the park areas and strive towards locating treatment combinations randomly across such potential paths. During summer 2020 an unanticipated dog-walking path appeared across the demonstration area (Figure 3) in such a way that all control treatment trees were located on one side of the path. As several of the trees there dried and had to be replaced, it was difficult to deduct what caused the phenomena: the path, the growing media or the nursery quality of the trees.
Conclusion
To achieve the goals of carbon (C) neutrality within next 20 year, municipalities worldwide need to increasingly apply negative emission technologies. We focus on the main principles of urban demonstration areas using trees and biochars for C sequestration and found that demonstration sites of urban C sinks in public parks need to be safe, visible and scientifically sound for reliable and cost-effective verification of carbon sequestration. We found that different interests can be arbitrated and that synergy that emerges from co-creation of urban C sink parks between stakeholders (scientists, city officials, companies, and citizens) can result in demo areas with maximized potential for impact.
Ethics Statement
Written informed consent was obtained from the relevant individual(s) for the publication of any potentially identifiable images or data included in this article.
Author Contributions
PT: Initial idea, Resources, Planning, Writing, Revision, Correspondence PS, ST, MK, and A-RS: Planning, Writing, Revision. AR, ES, and MJ: Initial idea, Resources, Planning, Writing, Revision TK: Visualization, Writing, Revision.
Conflict of Interest
The authors declare that the research was conducted in the absence of any commercial or financial relationships that could be construed as a potential conflict of interest.
Publisher’s Note
All claims expressed in this article are solely those of the authors and do not necessarily represent those of their affiliated organizations, or those of the publisher, the editors and the reviewers. Any product that may be evaluated in this article, or claim that may be made by its manufacturer, is not guaranteed or endorsed by the publisher.
Acknowledgments
We acknowledge the funding for the Carbon Lane project by EIT Climate KIC Early innovation project # 190365, the full report the current policy brief is based on is available at https://www.aalto.fi/fi/Carla. We also acknowledge the funding from Maiju ja Yrjö Rikalan Puutarhasäätiö for funding the first follow-up project of the Carbon Lane park, #Hiilipuisto. We are very grateful also for all the stakeholders and scientists who participated the workshops providing input for both the principles and design for the urban C sink parks.
References
Amonette, J. E., Blanco-Canqui, H., Hassebrook, C., Laird, D. A., Lal, R., Lehmann, J., et al. (2021). Integrated Biochar Research: A Roadmap. J. Soil Water Conservation 76, 24A–29A. doi:10.2489/jswc.2021.1115a
Bird, M. (2015). “Test Procedures for Biochar Analysis in Soils,” in In: Biochar for Environmental Management: Science, Technology and Implementation. Editors J. Lehmann, and S. Joseph (New YorkAbingdon, Oxon): Routledge), 679–716.
Blanco‐Canqui, H., Laird, D., Heaton, E., Rathke, S., and Acharya, B. S. (2020). Soil Carbon Increased by Twice the Amount of Biochar Carbon Applied after Six Years: Field Evidence of Negative Priming. 12, GCB Bioenergy. doi:10.1111/gcbb.12665
Brown, S., Miltner, E., and Cogger, C. (2012). Carbon Sequestration Potential in Urban Soils, Carbon Sequestration in Urban Ecosystems. Dordrecht: Springer, 173–196. doi:10.1007/978-94-007-2366-5_9
City of Helsinki (2018). The Carbon-Neutral Helsinki 2035 Action Plan, 4. Publications of the Central Administration of the City of, 121. Available at: https://www.hel.fi/static/liitteet/kaupunkiymparisto/julkaisut/julkaisut/HNH-2035/Carbon_neutral_Helsinki_Action_Plan_1503019_EN.pdf, Accessed 2 25, 2021).
EBC (2012). European Biochar Certificate - Guidelines for a Sustainable Production of Biochar. Arbaz, Switzerland: European Biochar Foundation (EBC). Available at: http:European-biochar.org, Accessed 25 2 2021.Version 9.2E of 2nd December 2020
FAO (2019). Measuring and Modelling Soil Carbon Stocks and Stock Changes in Livestock Production Systems: Guidelines for Assessment (Version 1) Licence: CC BY-NC-SA 3.0 IGO, Livestock Environmental Assessment and Performance (LEAP) Partnership. Rome: FAO, 170.
Hammes, K., Schmidt, M. W. I., Smernik, R. J., Currie, L. A., Ball, W. P., Nguyen, T. H., et al. (2007). Comparison of Quantification Methods to Measure Fire-Derived (Black/elemental) Carbon in Soils and Sediments Using Reference Materials from Soil, Water, Sediment and the Atmosphere. Glob. Biogeochem. Cycles 21, a–n. doi:10.1029/2006gb002914
Kuzyakov, Y., Bogomolova, I., and Glaser, B. (2014). Biochar Stability in Soil: Decomposition during Eight Years and Transformation as Assessed by Compound-specific 14C Analysis. Soil Biol. Biochem. 70, 229–236. doi:10.1016/j.soilbio.2013.12.021
McHale, M. R., Burke, I. C., Lefsky, M. A., Peper, P. J., and McPherson, E. G. (2009). Urban forest Biomass Estimates: Is it Important to Use Allometric Relationships Developed Specifically for Urban Trees? Urban Ecosyst. 12, 95–113. doi:10.1007/s11252-009-0081-3
Nayak, A. K., Rahman, M. M., Naidu, R., Dhal, B., Swain, C. K., Nayak, A. D., et al. (2019). Current and Emerging Methodologies for Estimating Carbon Sequestration in Agricultural Soils: A Review. Sci. Total Environ. 665, 890–912. doi:10.1016/j.scitotenv.2019.02.125
Nordbo, A., Järvi, L., and Vesala, T. (2012). Revised Eddy Covariance Flux Calculation Methodologies - Effect on Urban Energy Balance. Tellus B: Chem. Phys. Meteorology 64, 18184. doi:10.3402/tellusb.v64i0.18184
Norton, B. A., Bending, G. D., Clark, R., Corstanje, R., Dunnett, N., Evans, K. L., et al. (2019). Urban Meadows as an Alternative to Short Mown Grassland: Effects of Composition and Height on Biodiversity. Ecol. Appl., e01946. doi:10.1287/acaff3d5-4552-4fb3-8e7c-3d6c7a70dda9
Obia, A., Børresen, T., Martinsen, V., Cornelissen, G., and Mulder, J. (2017). Vertical and Lateral Transport of Biochar in Light-Textured Tropical Soils. Soil Tillage Res. 165, 34–40. doi:10.1016/j.still.2016.07.016
Olson, K. R. (2013). Soil Organic Carbon Sequestration, Storage, Retention and Loss in U.S. Croplands: Issues Paper for Protocol Development. Geoderma 195-196, 201–206. doi:10.1016/j.geoderma.2012.12.004
Pataki, D. E., Carreiro, M. M., Cherrier, J., Grulke, N. E., Jennings, V., Pincetl, S., et al. (2011). Coupling Biogeochemical Cycles in Urban Environments: Ecosystem Services, green Solutions, and Misconceptions. Front. Ecol. Environ. 9, 27–36. doi:10.1890/090220
Paustian, K., Lehmann, J., Ogle, S., Reay, D., Robertson, G. P., and Smith, P. (2016). Climate-smart Soils. Nature 532, 49–57. doi:10.1038/nature17174
IPCC, (2019). in Climate Change and Land: An IPCC Special Report on Climate Change, Desertification, Land Degradation, Sustainable Land Management, Food Security, and Greenhouse Gas Fluxes in Terrestrial Ecosystems. Editors P. R. Shukla, J. Skea, E. Calvo Buendia, V. Masson-Delmotte, H.- O. Pörtner, D. C. Robertset al. Available at: https://spiral.imperial.ac.uk/bitstream/10044/1/76618/2/SRCCL-Full-Report-Compiled-191128.pdf
Riikonen, A., Pumpanen, J., Mäki, M., and Nikinmaa, E. (2017). High Carbon Losses from Established Growing Sites Delay the Carbon Sequestration Benefits of Street Tree Plantings - A Case Study in Helsinki, Finland. Urban For. Urban Green. 26, 85–94. doi:10.1016/j.ufug.2017.04.004
Smith, P., Soussana, J. F., Angers, D., Schipper, L., Chenu, C., Rasse, D. P., et al. (2020). How to Measure, Report and Verify Soil Carbon Change to Realize the Potential of Soil Carbon Sequestration for Atmospheric Greenhouse Gas Removal. Glob. Change Biol. 26, 219–241. doi:10.1111/gcb.14815
Tanhuanpää, T., Kankare, V., Setälä, H., Yli-Pelkonen, V., Vastaranta, M., Niemi, M. T., et al. (2017). Assessing Above-Ground Biomass of Open-Grown Urban Trees: A Comparison between Existing Models and a Volume-Based Approach. Urban For. Urban Green. 21, 239–246. doi:10.1016/j.ufug.2016.12.011
Velasco, E., and Roth, M. (2010). Cities as Net Sources of CO2: Review of Atmospheric CO2 Exchange in Urban Environments Measured by Eddy Covariance Technique. Geogr. Compass 4, 1238–1259. doi:10.1111/j.1749-8198.2010.00384.x
Keywords: biochar, carbon sequestration, demonstration sites, soils, trees, urban parks
Citation: Tammeorg P, Soronen P, Riikonen A, Salo E, Tikka S, Koivunen M, Salonen A-R, Kopakkala T and Jalas M (2021) Co-Designing Urban Carbon Sink Parks: Case Carbon Lane in Helsinki. Front. Environ. Sci. 9:672468. doi: 10.3389/fenvs.2021.672468
Received: 26 February 2021; Accepted: 04 August 2021;
Published: 19 August 2021.
Edited by:
Claudia Irene Kammann, Hochschule Geisenheim University, GermanyReviewed by:
Gerard Cornelissen, Norwegian Geotechnical Institute, NorwayKurt A. Spokas, Agricultural Research Service, United States Department of Agriculture, United States
Copyright © 2021 Tammeorg, Soronen, Riikonen, Salo, Tikka, Koivunen, Salonen, Kopakkala and Jalas. This is an open-access article distributed under the terms of the Creative Commons Attribution License (CC BY). The use, distribution or reproduction in other forums is permitted, provided the original author(s) and the copyright owner(s) are credited and that the original publication in this journal is cited, in accordance with accepted academic practice. No use, distribution or reproduction is permitted which does not comply with these terms.
*Correspondence: Priit Tammeorg, priit.tammeorg@helsinki.fi