- 1Plant Ecology, Institute of Biology, Freie Universität Berlin, Berlin, Germany
- 2Berlin-Brandenburg Institute of Advanced Biodiversity Research (BBIB), Berlin, Germany
Microplastic pollution is a topic of increasing concern, especially since this issue was first addressed in soils. Results have so far been variable in terms of effects, suggesting that there is substantial context-dependency in microplastic effects in soil. To better define conditions that may affect microplastic-related impacts, we here examined effects as a function of microplastic shape and polymer type, and we tested if effects on soil properties and soil microbial activities change with incubation time. In our laboratory study, we evaluated twelve different secondary microplastics representing four microplastic shapes: fibers, films, foams and fragments; and eight polymer types: polyamide (PA), polycarbonate (PC), polyethylene (PE), polyester (PES), polyethylene terephthalate (PET), polypropylene (PP), polystyrene (PS), and polyurethane (PU). We mixed the microplastics with a sandy soil (0.4% w/w) and incubated at 25°C for 31 days. Then, we collected soil samples on the 3rd, 11th, and 31st day, and measured soil pH, respiration and four enzyme activities (soil enzymatic activities). Our results showed that microplastics could affect soil pH, respiration and enzymatic activities depending on microplastic shape and polymer type, effects that were altered with incubation time. Soil pH increased with foams and fragments and overall decreased in the first days of incubation and then increased. Soil respiration increased with PE foams and was affected by the incubation time, declining over time. Overall, acid phosphatase activity was not affected by shape or polymer type. β-D-glucosidase activity decreased with foams, cellobiosidase activity decreased with fibers, films and foams while N-acetyl-β-glucosaminidase activities decreased with fibers and fragments. Enzymatic activities fluctuated during the incubation time, except N-acetyl-β-glucosaminidase, which showed a declining trend with incubation time. Enzymatic activities were negatively correlated with soil pH and this relationship was less strong when microplastics were added to the soil. Our study adds to the evidence that research should embrace the complexity and diversity of microplastics, highlighting the role of microplastic shape and polymer type in influencing effects; additionally, we show that incubation time is also a parameter to consider, as effects are dynamic even in the short term.
Introduction
Large amounts of plastics have been produced worldwide due to the widespread use of these materials in our daily life (Geyer et al., 2017), to the point that plastic is now becoming an important threat to terrestrial systems (Rillig 2012; Bläsing and Amelung 2018). Microplastics, plastic particles smaller than 5 mm, and their effects on soil systems, have received increasing attention in recent years (Rillig 2012; Mai et al., 2018). They can pollute terrestrial systems through a variety of pathways, including soil amendments, mulching, sludge, irrigation, flooding, atmospheric input and littering or street runoff (Rillig et al., 2017; Mai et al., 2018; Boots et al., 2019).
As a result of their manufacturing origin and environmental degradation, microplastics may occur in many shapes and a variety of physical and chemical properties (Helmberger et al., 2020; Rillig and Lehmann, 2020). The accumulation of microplastics in soil may impact soil characteristics (Liu et al., 2017; Yi et al., 2020), depending on microplastic properties (Lozano et al., 2021a). Indeed, microplastic shape may determine how microplastics interact with soil particles (de Souza Machado et al., 2018; Rillig et al., 2019a; Lehmann et al., 2020; Rillig and Lehmann 2020). For instance, fibers due to their linear shape, may destabilize soil structure once they are incorporated into soil aggregates (de Souza Machado et al., 2018).
In addition, the chemical properties of microplastics, such as molecular chain arrangement and functional group, could impact their capacity of absorption to other chemicals like heavy metals or antibiotics (Fred-Ahmadu et al., 2020), with potential consequences on soil properties and microbial activities (Pathan et al., 2020). For example, polyethylene (PE) had high sorption capacity for phenanthrene (Wang and Wang 2018), which along with its nitrogen heterocyclic analogues could inhibit microbial activities in soil (Anyanwu and Semple 2016). Likewise, studies have shown that different polymer types (e.g., PE, PP and PVC) may have different sorption capacities for certain chemicals (Teuten et al., 2009; Brennecke et al., 2016; Wang et al., 2018). For example, PE had greater sorption capacity for hydrophobic organic compounds such as pesticide and solvents (Teuten et al., 2009; Fred-Ahmadu et al., 2020), while PS had larger sorption capacity for Polycyclic Aromatic Hydrocarbons than PET, PVC, PE or PP (Rochman et al., 2013). In the same way, PVC could absorb more Cu than PS (Brennecke et al., 2016). Therefore, the effects of microplastics on soil enzymatic activities may be also influenced by their polymer type.
Among soil properties, little is known about microplastics effects on soil pH, a key soil parameter that could impact a range of microbial processes (Higashida and Takao, 1986). Some research has been done regarding the effects of PE on soil pH. For instance, low density polyethylene (LDPE) films may increase soil pH (Qi et al., 2020); while high density polyethylene (HDPE), may have the opposite pattern (Boots et al., 2019), however, a study by Wang et al. (2020) suggested that HDPE may also cause an increase in soil pH. Yet, how other types of microplastics (shapes or polymers) present in terrestrial systems (Bläsing and Amelung, 2018; Piehl et al., 2018; Rillig et al., 2019b) may affect this soil property is currently unknown.
In addition, our knowledge of microplastic effects on soil respiration is still rudimentary. Soil respiration, an indicator of the total soil microbial activity (Rousk et al., 2009), is very sensitive to environmental factors, such as soil texture, porosity, moisture, and pH (Luo and Zhou, 2006), soil properties that can be potentially altered by microplastics addition (Rillig et al., 2019b; de Souza Machado et al., 2019; Lozano et al., 2021a). Indeed, recent research has observed that microplastics could alter the soil microbial community (Huang et al., 2019; Fei et al., 2020), suggesting potential effects on soil respiration (Lozano et al., 2021a; Lozano et al., 2021b).
Microplastics could alter soil microbial communities (Fei et al., 2020; Wiedner et al., 2020; Yi et al., 2020), affecting enzymatic activities (Hargreaves and Hofmockel, 2014). Indeed, recent research has showed that microplastics could affect nutrient and/or substrate availability (Yu et al., 2020; Zhou et al., 2020; Lozano et al., 2021b), likely due to microplastic absorption or its competition for physicochemical niches with microorganisms (Yu et al., 2020). Microplastic shape and polymer type may also play a role. For instance, PE and polyvinyl chloride (PVC) microplastics could enhance enzymes such as urease and acid phosphatase (Huang et al., 2019; Fei et al., 2020) while PP, PES and PVC could inhibit or enhance soil fluorescein diacetate hydrolase activity, respectively (Liu et al., 2017; de Souza Machado et al., 2019; Liang et al., 2019; Fei et al., 2020), depending on the polymer type. Likewise, enzymes such as β-D-glucosidase and cellobiosidase (involved in cellulose degradation), N-acetyl-β-glucosaminidase (involved in chitin degradation), and phosphatase which are related to C, N, P-cycling, could be negatively affected by microplastics (Lozano et al., 2021b; Liang et al., 2021).
Depending on the shape, polymer type and exposure time, microplastics can have different effects on soil properties, adding to the strong context dependency of microplastic effects as reported in the literature. To systematically test this, we established a lab experiment that included four microplastic shapes (fibers, films, foams and fragments), each of them made of three different polymer types, in order to determine the effects of microplastics on soil pH and microbial activity. We hypothesized that soil pH, respiration and enzymatic activities may be affected by microplastic addition as a function of microplastic shape and polymer type; in addition, we examined effects of exposure time during our short-term laboratory incubation.
Materials and Methods
Test soil. We selected a loamy sandy soil from a dry grassland community located in Dedelow, Brandenburg, Germany (53° 37′ N, 13° 77′ W). Dry soil was sieved through a 2-mm mesh sieve, homogenized and mixed with microplastics. The detailed properties of test soil are shown in Table 1.
Microplastics. Primary microplastics are produced on purpose and used in cosmetic products and various industries, while secondary microplastics are obtained from degradation of larger plastics (Wang et al., 2018). We selected twelve different secondary microplastics, representing four microplastic shapes: fibers, films, foams and fragments and eight polymer types: polyamide (PA), polycarbonate (PC), PE, polyester (PES), polyethylene terephthalate (PET), polypropylene (PP), polystyrene (PS), and polyurethane (PU). See additional details on the plastics in Table 2. We manually cut the fibers and films with scissors. The length for fibers was 1.26 ± 0.03 mm, and the size of films was 1.55 ± 0.03 mm × 2.26 ± 0.04 mm. Plastic fragments and foams were cut into small pieces using a Philips HR3655/00 Standmixer (1,400 Watt, ProBlend 6 3D Technologie, Netherlands), and then sieved through a 4-mm mesh sieve. The sizes for the fragments were 1.28 ± 0.05 mm × 1.72 ± 0.07 mm, while for the foams were 1.28 ± 0.04 mm × 1.76 ± 0.06 mm. To minimize microbial contamination, microplastics were exposed in an oven at 101°C for 24 h, as previous assays using different temperatures, showed that at this temperature, microplastics did not present any type of distortion. Then, a sample of each microplastic was placed on PDA plates (PDA X931.2, Roth, Germany), which were incubated at 25°C for 1 week. No microbial colonies were observed.
Microplastic addition to soil. The soil was mixed with each of the microplastic types at a concentration of 0.4% (w/w), as this simulates higher levels of microplastic pollution (Scheurer and Bigalke, 2018; Xue et al., 2020; Zhu et al., 2019a), since we should be more concerned about the future than the current levels of microplastic contamination, just like is the case of other factors of global change. Therefore, 80 mg of each microplastic type were mixed into 20 g of soil by stirring with a metal spoon for 3 min in a large container before transferring the mixture into a 50-ml polypropylene centrifuge tube (Corning 431,720, Corning Incorporated), the caps of which had four vents to provide gas exchange. We had 12 microplastic types (4 shapes × 3 polymer types) × 9 replicates = 108 tubes. Fifteen additional tubes were included as a control without microplastics. Soil was stirred in the same way that in the control samples, to provide the same disturbance. All tubes were randomly distributed in the incubator chamber.
Throughout the incubation period, in order to maintain soil moisture at ∼70% water holding capacity, every four days we pipetted distilled water into the tubes according to their weight loss due to evaporation. Tubes were kept at 25°C throughout the experiment. Soil samples were randomly collected on the 3rd, 11th and 31st day. To avoid disturbance which could be a confounding factor, 1/3 of the samples were collected (three replicates for each microplastic treatment and five replicates for control), destructively harvested and analyzed for every harvest time (on the 3rd, 11th and 31st day). At harvest, soil respiration was measured, and then samples were collected and kept at 4 °C prior to measuring enzymatic activities and soil pH.
Measurements
Soil pH. Soil pH was measured following the procedure described by Hendershot and Lalande (2007). That is, air-dried soil samples were mixed with distilled water at the ratio of 1:2 (w: v), i.e., 10 g soil: 20 ml water. The tubes were shaken for 30 min and the suspensions were allowed to settle for 1 h. Then, 20 ml of each suspension was pipetted into a 50-ml tube (Sarstedt AG & Co. KG, Nümbrecht, Germany, item number 62.548.004) and centrifuged at 3,000 rpm for 5 min. The supernatants were filtered, and the pH was determined with a pH-meter 766 (Knick, Germany).
Enzyme activities. Acid phosphatase (EC3.1.3.2), β-D-glucosidase (EC3.2.1.21), cellobiosidase (EC3.2.1.91) and N-acetyl-β-glucosaminidase (EC3.2.1.52) were measured from 5 g of soil by using high throughput microplates assays following the methods described by Jackson et al. (2013). Briefly, 5 g soil was mixed with 10 ml 50 mM acetate buffer (pH 5.0–5.4) in a 50-ml falcon tube. Then, 150 ul of soil slurry was pipetted into each of six wells (six wells per sample) on a 96-deep well plate after vortexing. Then, 150 ul acetate buffer was added into the last two wells of each sample (sample buffer control), and 150 ul substrate solutions (5 mM 4-p-nitrophenyl-phosphate disodium salt hexahydrate, 5 mM 4-p-nitrophenyl-β-glucopyranoside, 2 mM 4-p-nitrophenyl-β-D-cellobioside and 2 mM 4-p-nitrophenyl-β-N-acetylglucosaminide, Sigma, Germany, item no.: N71768, N7006, N5759, and N9376) to the first four wells. Then, the plates were incubated at 25°C in dark for 2 h (for acid phosphatase and β-D-glucosidase) or 4 h (for cellobiosidase and N-acetyl-β-glucosaminidase). After the incubation, plates were centrifuged at 3,000 rpm for 5 min, and then 100 ul supernatant from each well was transferred into new microplates containing 10 ul 1M NaOH and 190 ul distilled water in each well. Finally, the absorbance at 410 nm was recorded by a microplate reader (Benchmark Plus Microplate Spectrophotometer System, BioRad Laboratories, Hercules, CA, United States).
Soil respiration. Soil respiration was measured on undisturbed soil samples. That is, each time soil respiration was measured before sample collection for enzymes and pH measurements. Therefore, on the 3rd day, soil respiration was measured on all replicates tubes; on the 11th day, it was measured on the six microplastic and ten control replicates remaining; and on the 31st day, it was measured on the three microplastic and five control replicates left. The CO2 concentration (ppm) was used to indicate the soil respiration. To control gas exchange, we used modified tube caps that had a rubber septum (VWR, Germany, item no. 548-3369) to provide a seal. Then, we flushed the tubes with CO2-free air for 3 min to normalize the experimental units and kept the tubes in the incubator at 25°C for 3 h under dark conditions. After the 3-h incubation, we took a 1-ml air sample from each tube and injected it into the infrared gas analyzer (LiCOR- 6400XT).
Statistical Analyses
The effects of microplastic shape, polymer type and exposure time on soil pH, respiration and enzymatic activities were analyzed using linear models and multiple comparisons. First, the residuals of linear models were checked to validate assumptions of normality and homogeneity. When necessary, we implemented the functions “varIdent” from the “vegan” R package to account for heterogeneity in variances. Then, we implemented the function “glht” and “Dunnet” test from the “multcomp” R package, to compare each microplastics treatment with the control (without microplastics). Respiration and enzymatic activities were log-transformed and correlated with soil pH by using the Pearson method. Plots were generated with the “ggplot2” R package (Wickham, 2016). Results shown throughout the text and figures are mean values ±1 SE. All analyses were conducted using R software version 3.6.3 (R Core Team, 2020).
Results
Soil pH. Soil pH was affected by microplastic shapes, polymer types, and incubation time (Table 3–5, Figure 1). Soil pH increased with foams and fragments and a slightly increase was observed with films (Table 4; Figure 1A). We found that pH was higher in the soil mixed with all the polymers used for foams and fragments than in control soils without microplastics (Table 4; Figure 1A). Regarding exposure time, overall, soil pH declined in the first 11 days and then increased (Table 5; Figure 1B). This pattern was observed for PA and PES fibers, all the films, PU foams, PC and PP fragments. However, pH of the soil treated with PE foams showed a contrary trend (Table 5; Figure 1B). Soil pH tended to increase over time with PS foams and PET fragments addition (Table 5; Figure 1B). Overall, pH was higher in soil mixed with foams and fragments polymers for each time of measurement, than in soils without microplastics (Table 5; Figure 1B).

TABLE 3. Microplastic type and exposure time (time) effects on soil pH, respiration, and enzymatic activities.
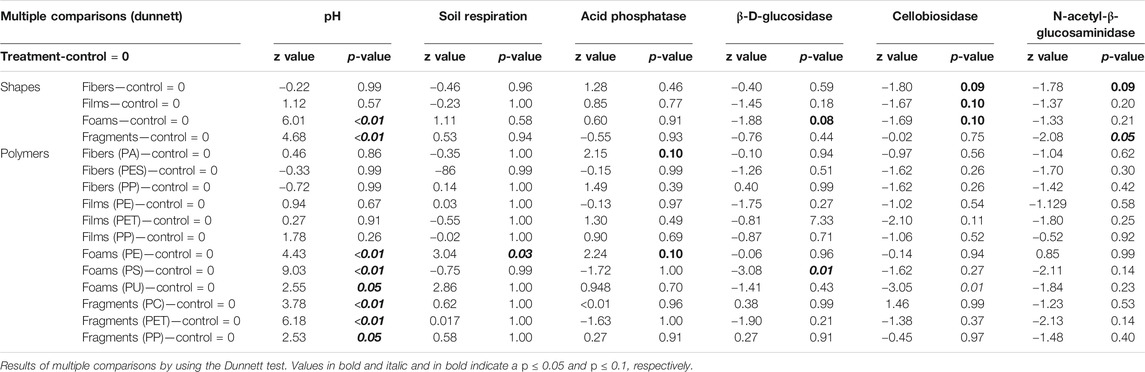
TABLE 4. Microplastic shape and polymer type effects on soil pH, respiration, and enzymatic activities.
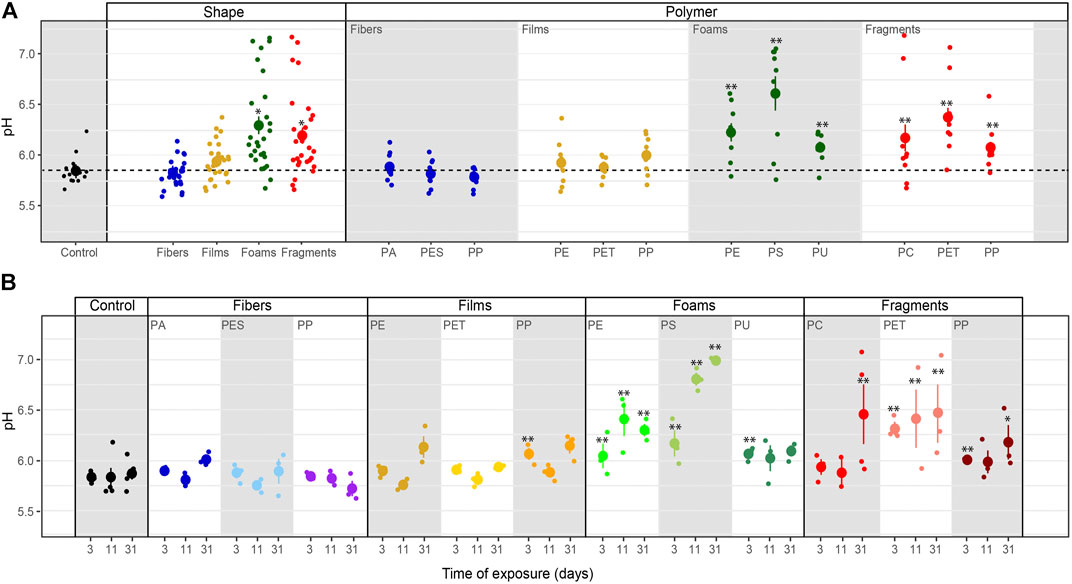
FIGURE 1. Microplastics effects on soil pH. Effects of shape, polymer type (A) and incubation time (B) on soil pH. Mean and standard error are shown. n = 5 (control); n = 3 (microplastic treatments). Significance *p ≤ 0.1, **p ≤ 0.05 compares each microplastic with its respective control treatment for each time of measurement.
Soil Respiration. Soil respiration was not affected by microplastic shapes, although it slightly increased with foams (Table 3–5; Figure 2). Only PE foams increased soil respiration within all the polymer types (Table 4 and 5; Figure 2). Overall, soil respiration declined over time, being more evident in soils with than without microplastics (Table 5; Figure 2B). Soil respiration was lower in soil mixed with each of all the microplastic types at the last measurement time (day 31st), than in soils without microplastics (Table 5; Figure 2B).
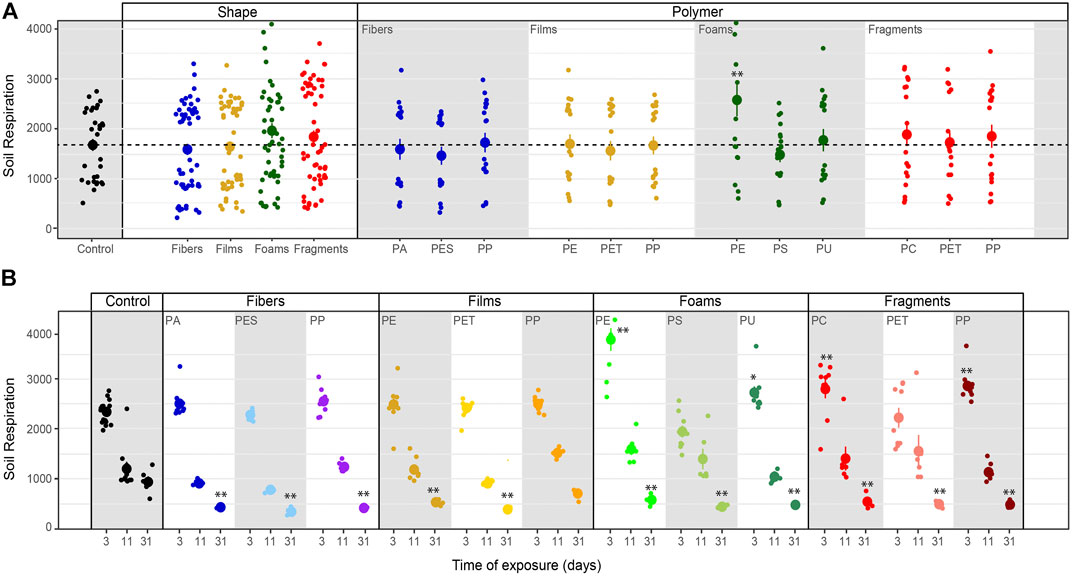
FIGURE 2. Microplastics effects on soil respiration. Effects of shape, polymer type (A) and incubation time (B) on soil respiration. Mean and standard error are shown (n = 15 for control; n = 9 for microplastic treatments, day 3); n = 10 (control, day 11); n = 6 (microplastic treatments, day 11); n = 5 (control, day 31); n = 3 (microplastic treatments, day 31). Soil respiration measured as CO2 unit (ppm). Significance *p ≤ 0.1, **p ≤ 0.05 compares each microplastic with its respective control treatment for each time of measurement.
Enzymatic Activities
Acid phosphatase activity. Overall, acid phosphatase activity was not affected by microplastic shape although it tended to be higher with fibers, films and foams than in control samples without microplastics (Table 3–5; Figure 3). We observed that this enzyme increased with PA fibers and PE foams (Table 4; Figure 3A). Overall, acid phosphatase activity tended to decline during the first 11 days and then increased (Table 5; Figure 3B). This pattern was evident for PA and PP fibers, PP films, PE foams and PET fragments. Over time, acid phosphatase activity tended to decline with PES fibers, PS and PU foams, while tended to increase with PET films and PP fragments (Table 5; Figure 3B). Likewise, acid phosphatase activity was negatively correlated with soil pH when microplastics in the soil were absent (R = −0.55, p = 0.034) or present (R = −0.47, p < 0.01, Figure 4).
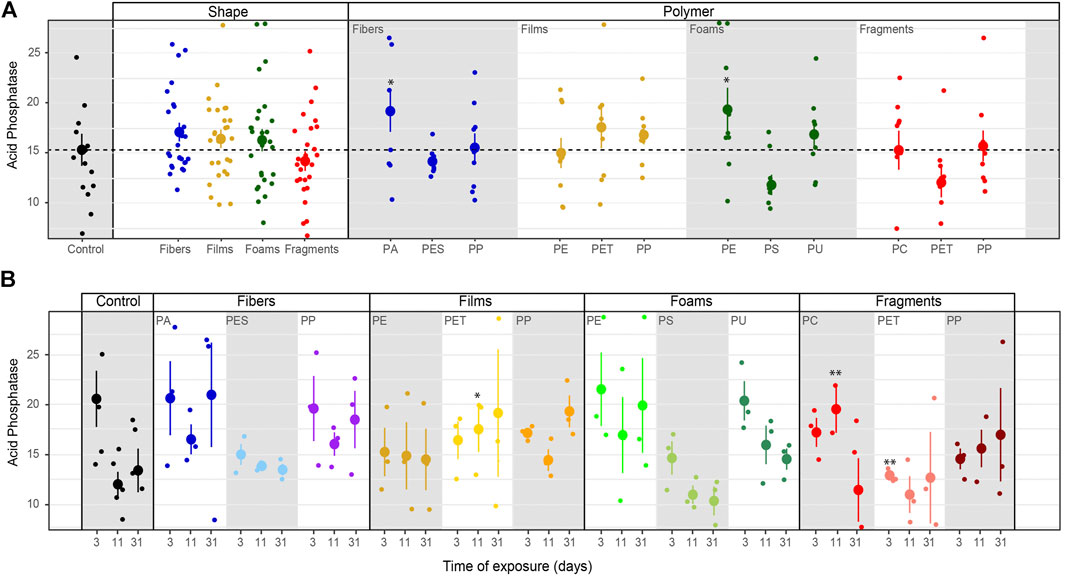
FIGURE 3. Microplastics effects on acid phosphatase. Effects of shape, polymer type (A) and incubation time (B) on acid phosphatase. Mean and standard error are shown. n = 5 (control); n = 3 (microplastic treatments). Unit: μmol mg−1 h−1. Significance *p ≤ 0.1, **p ≤ 0.05 compares each microplastic with its respective control treatment for each time of measurement.
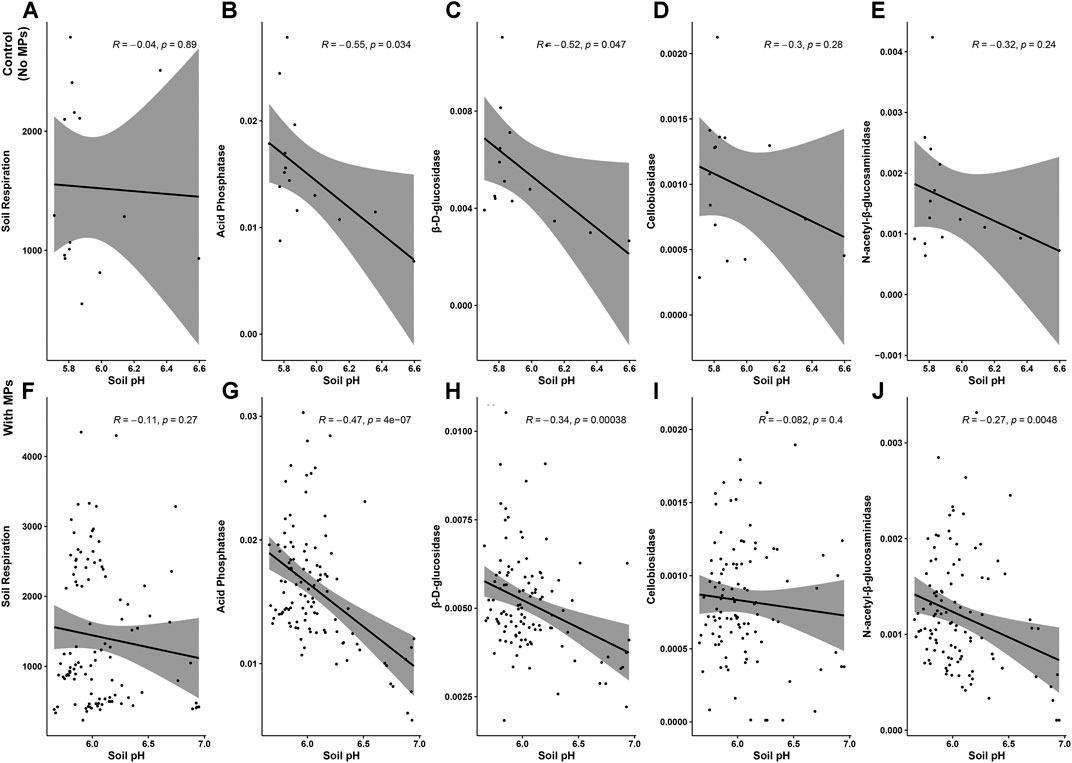
FIGURE 4. The negative correlation between microplastic effects on soil pH and microbial activities. The data for enzymatic activities were log-transformed to generate the figures. Negative correlation between soil pH and microbial activities for the control (A–E), and the microplastic treatment (F–J).
β-D-glucosidase activity. β-D-glucosidase activity decreased with foams although it also tended to decrease in the presence of the other microplastic shapes (Table 3–5; Figure 5). Specifically, β-D-glucosidase activity decreased with PS foams (Table 4; Figure 5A). Over time, β-D-glucosidase activity declined with PA fibers, PE and PET films, and PS foams, while tended to increase with PP fragments. Overall, β-D-glucosidase activity was lower in soil mixed with foams, films and fragments polymers for the first time of measurement (PE and PP films, PS foams and all fragments) than in soils without microplastics, while it was higher in soil with PE foams than control for the last time of measurement (Table 5; Figure 5B). This enzymatic activity was negatively correlated with soil pH without or with microplastics in the soil (R = −0.52, p = 0.047, R = −0.34, p < 0.01, respectively, Figure 4).
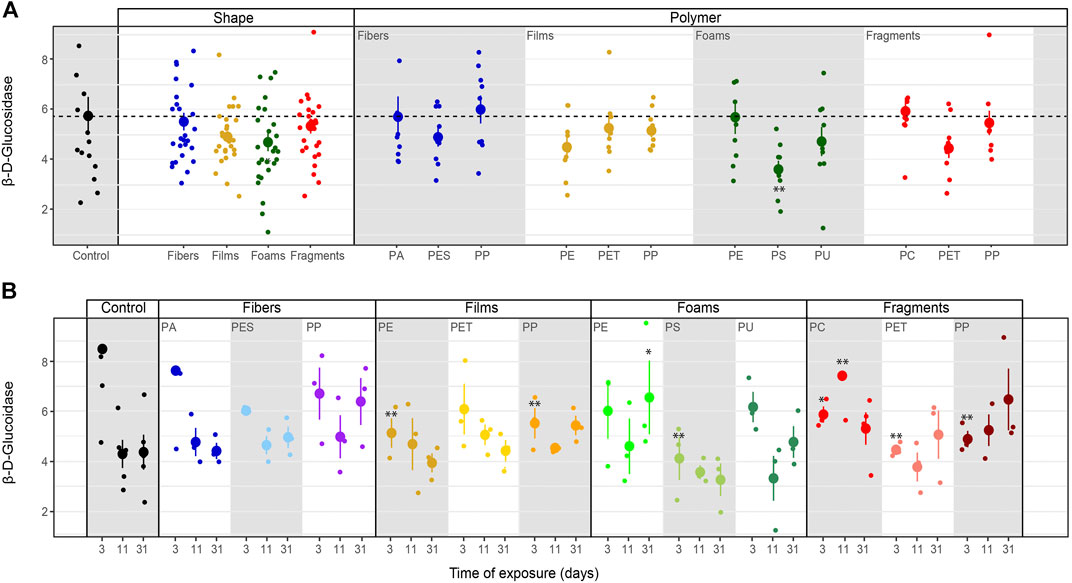
FIGURE 5. Microplastics effects on β-D-glucosidase. Effects of shape, polymer type (A) and incubation time (B) on β-D-glucosidase. Mean and standard error are shown. n = 5 (control); n = 3 (microplastic treatments). Unit: μmol mg−1 h−1. Significance *p ≤ 0.1, **p ≤ 0.05 compares each microplastic with its respective control treatment for each time of measurement.
Cellobiosidase activity. Cellobiosidase activity was reduced by all microplastic shapes except fragments, whose effects were similar to control (Table 3–5; Figure 6). In particular, cellobiosidase activity decreased with PET films and PU foams (Table 4; Figure 6A). Over time, this enzyme tended to decline with PA and PP fibers, while showing a contrary trend with PET, PP films and PE foams. Overall, cellobiosidase activity was lower in soils mixed with microplastics of different polymer type (for the first time of measurement) than in soils without microplastics, while promoted by PE films and foams, and fragment polymers for the last time of measurement (Table 5; Figure 6B). Cellobiosidase activity was not correlated with soil pH when microplastics in the soil were absent (R = −0.3, p = 0.28) or present (R = −0.08, p = 0.4, Figure 4).
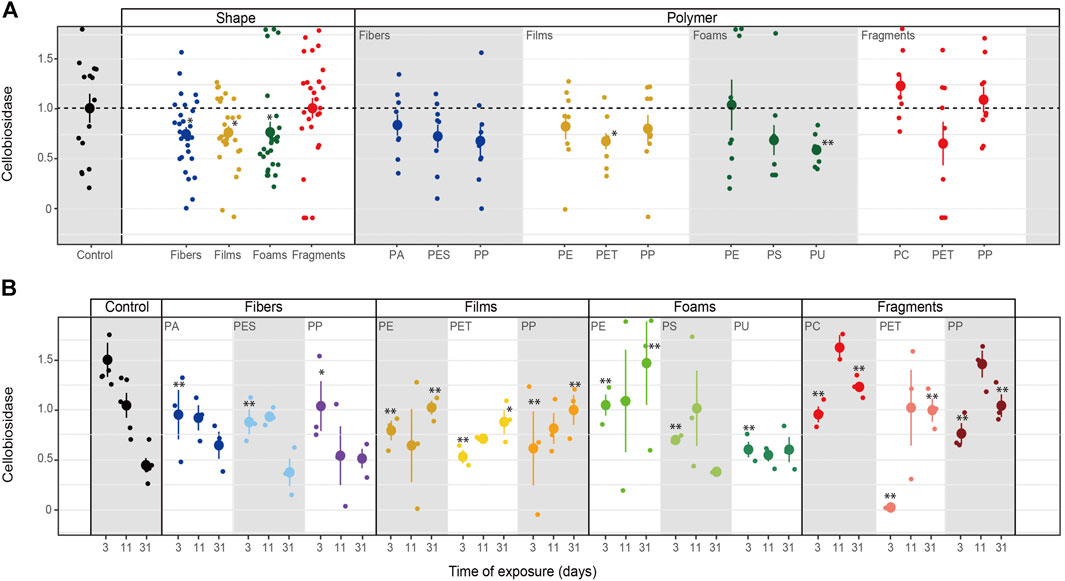
FIGURE 6. Microplastics effects on cellobiosidase. Effects of shape, polymer type (A) and incubation time (B) on cellobiosidase. Mean and standard error are shown. n = 5 (control); n = 3 (microplastic treatments). Unit: μmol mg−1 h−1. Significance *p ≤ 0.1, **p ≤ 0.05 compares each microplastic with its respective control treatment for each time of measurement.
N-acetyl-β-glucosaminidase activity. N-β-glucosaminidase activity was lower in the presence of microplastic fibers and fragments compared to the control and was neutrally or slight negatively affected by all the polymers (Table 3–5; Figure 7). N-acetyl-β-glucosaminidase activity steadily decreased with films, foams and fragments over time (Table 5; Figure 7B). Overall, this enzyme activity was lower in soils mixed with microplastics of different polymer type for the first time of measurement, than in soils without microplastics (Table 5; Figure 7B). Likewise, N-acetyl-β-glucosaminidase activity was not correlated with soil pH when microplastics in the soil were absent (R = −0.32, p = 0.24) but it was negatively correlated when the microplastics were present (R = −0.27, p = <0.01, Figure 4).
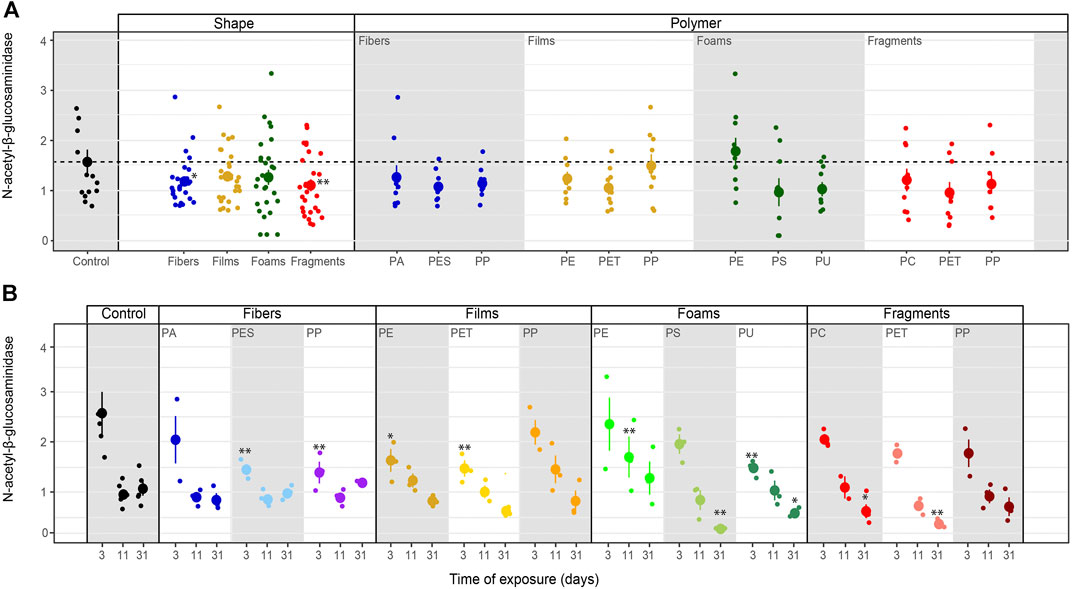
FIGURE 7. Microplastics effects on N-acetyl-β-glucosaminidase. Effects of shape, polymer type (A) and incubation time (B) on N-acetyl-β-glucosaminidase. Mean and standard error are shown. n = 5 (control); n = 3 (microplastic treatments). Unit: μmol mg−1 h−1. Significance *p ≤ 0.1, **p ≤ 0.05 compares each microplastic with its respective control treatment for each time of measurement.
Discussion
Our results showed that microplastic effects on soil pH, respiration, and enzymatic activities depended on microplastic shape, polymer type, and the effects changed with incubation time.
Microplastics Increased Soil pH
Our results indicated that microplastic foams and fragments increased soil pH, which can be due to the increase in soil aeration and porosity when these microplastics were added into the soil (de Souza Machado et al., 2019; Lozano et al., 2021a). This along with the leaching of microplastic chemical compounds into the soil (Kim et al., 2020; Waldman and Rillig 2020), may alter soil biota with consequences for soil pH. Likewise, although slightly, microplastic films increased soil pH. In this regard, it has observed that PE films may alter the diversity of nitrogen fixation bacteria taxa in the soil (Fei et al., 2020), which would alter the contents of soil NH4+, increasing soil pH, as the conversion of organic N to NH4+ would consume H+ (Butterly et al., 2010; You et al., 2015). Notably, PE foams increased soil pH more than PE films, this may be due to the shape or additives differences. Our results showed that soil pH increased with PE polymers, which agrees with previous research on that topic (Qi et al., 2020; Wang et al., 2020). However, recent research indicates that soil type may also play a role (Boots et al., 2019; Yu et al., 2020); for example, depending on the soil organic matter content acid buffering and retention of major cations may change (Jiang et al., 2018). In addition to the soil type, the presence of plant species in the system may also influence microplastics effects on soil pH (Lozano et al., 2021b), as plants could potentially mitigate the effects of microplastics on soil pH in comparison to a bare soil. Finally, we found a negative correlation between soil pH and enzymatic activities.
Microplastics Affect Soil Respiration After Long Incubation Time
Our results also showed that soil respiration decreased over time for all microplastic treatments and the control, a situation that can be linked to the reduction of labile substrates (Chen and Wu 2019). However, we observed that after longer incubation time (i.e., 31 days), the decrease in respiration was more pronounced in soils with microplastics than without. This sharp decrease would be linked to the potential harmful effects of microplastics leachates on soil biota (Kim et al., 2020), a situation that was only evident after several days of soil subjected to microplastics. Added to this, our results showed that soil respiration was higher with PE foams than with the control (without microplastics). This positive effect may be due to their loose spongy structure that may increase soil aeration (Lehmann et al., 2020). This may could be the reason that PE foams caused higher respiration that PE films. Films and fragments had neutral effects on soil respiration, while positive effects have been observed on this property when a plant species was included in the system (Lozano et al., 2021a). The latter as the presence of roots in the soil matrix contributes to soil aggregation and facilitates water uptake and its redistribution through the soil profile, which in the end promote soil microbial activity (Lozano et al., 2021a).
Microplastics Have Negative Effects on Most Enzymatic Activities
Microplastics in the soil, inhibited most of the enzymatic activities. That is, β-D-glucosidase, cellobiosidase and N-acetyl-β-glucosaminidase activities.
Fibers negatively affected cellobiosidase and N-acetyl-β-glucosaminidase activities which can be linked to the negative effects that fibers may have on soil aggregation as they may prevent macroaggregates formation (Zhang and Liu 2018) and/or introduce fracture points into aggregates, affecting aggregate stability. As soil aggregation is positively correlated with soil microbial activity (Bronick and Lal, 2005), the negative effects of microplastics on soil aggregation may have consequences for soil microbial activity. Reduction in oxygen diffusion within the soil pores and the effects on water flows (Six et al., 2004) may explain the decrease in enzymatic activities. Likewise, changes in physicochemical niches, which provide space for growth and activity of soil microorganisms (Yu et al., 2020), would be altered with the presence of microplastics. In addition, N-acetyl-β-glucosaminidase activity could be reduced as macro-aggregates (>2 mm) where this enzyme is highly active (Wang et al., 2015), were affected in their formation due to the presence of microfibers (Lehmann et al., 2020). On the other hand, foams decreased β-D-glucosidase, cellobiosidase and N-acetyl-β-glucosaminidase activities, which can be linked with the sorption capacities of microplastics. Microplastics can carry toxic chemicals serving as vectors of transport for different pollutants (Wang et al., 2018) and in addition, different hazardous chemicals are voluntarily added during their production such as additives to increase polymer properties and prolong their life (Lithner et al., 2011). All these substances can be released into the soil matrix with negative effects on soil biota (Kim et al., 2020) and potentially on soil enzymatic activities.
Likewise, films caused lower activities of cellobiosidase and N-acetyl-β-glucosaminidase, which may be influenced by their negative effects on soil water evaporation (Wan et al., 2019), a soil condition that negatively affects soil microbial activity (Six et al., 2004). Films might also increase N-cycling microorganisms, and thus N-fixation (Fei et al., 2020), which could decrease N-acetyl-β-glucosaminidase activity as observed early in the incubation time (day 3) for PE and PET films. Similar to Yu et al. (2020), microplastic films had negative effects on β-D-glucosidase, however this was only evident at early stages of incubation (day 3).
Our results also showed that microplastics in the soil can stimulate enzymatic activity. Specifically, we observed that after some time of incubation, microplastic foams (e.g., PE) increased cellobiosidase, β-D-glucosidase (31 days) and N-acetyl-β-glucosaminidase (11 days) activities. This can be linked to the loose spongy structures of this plastic, which may increase soil pore space and thus water and air flows, promoting soil microbial activity. Indeed, PE foam was the microplastic that promoted the most soil respiration.
We observed that three enzymes (acid phosphatase, β-D-glucosidase and cellobiosidase) showed fluctuation trends during the incubation time. As recently observed, microplastics may have toxicity effects on soil biota after 24 h, although at higher concentration (1%) (Kim et al., 2020), which would have negative effects on microbial activity, thus causing reductions of the enzyme activities during the first days of exposure. Later, the microbiota may have adjusted to the new environmental conditions (Yi et al., 2020), and/or some of the toxic additives may have been inactivated or degraded, causing a rebound of enzyme activities. In that sense, previous studies indicated that microplastic effects on enzymatic activities might differ with incubation time i.e., 30 vs. 150 days (Liu et al., 2017; Yu et al., 2020).
Finally, we found that negative correlation between enzymatic activity and soil pH, which is consistent with previous studies (Adetunji et al., 2017; Ullah et al., 2019). However, this relationship was weakened in the presence of microplastics, as the increase in soil pH may promote the abundance, diversity, biomass and activity of certain bacterial groups (Rousk et al., 2009; Zhalnina et al., 2015), as observed for members of Acidobacteria, Nitrospira or Proteobacteria phyla (Rousk et al., 2010). In this way, microplastic may have weakened the negative correlation between soil pH and enzymatic activities.
Conclusion
Our study contributes to a better understanding of the effects that microplastics have on soil microbial activities, which can be linked among others, to the changes in soil pH. Likewise, our results suggest that microplastics can affect soil enzymes with potential consequences on C, N and P cycles. We found that in addition to including shape and polymer type as microplastics properties that affect soil systems (Lehmann et al., 2020; Rillig and Lehmann 2020; Wiedner and Polifka 2020; Lozano et al., 2021b), the exposure time of soil to the microplastics is another experimental parameter to consider, especially when studies report diverging effects. As the presence of plants, the type of soil and its content of organic matter (Lozano et al., 2021b; Liang et al., 2021) would influence the effects of microplastics on soil pH, respiration, and enzymatic activities, future specific research on this area is needed.
Data Availability Statement
The original contributions presented in the study are included in the article/Supplementary Material, further inquiries can be directed to the corresponding author.
Author Contributions
TZ, YL, and MR conceived the ideas and designed methodology; TZ established and maintained the experiment; TZ and YL analyzed the data. TZ wrote the first draft of this manuscript. All authors contributed to the final version and gave final approval for publication.
Funding
TZ acknowledges the China Scholarship Council for a scholarship (CSC No. 201608260012). MR acknowledges support from an ERC Advanced Grant (No. 694368).
Conflict of Interest
The authors declare that the research was conducted in the absence of any commercial or financial relationships that could be construed as a potential conflict of interest.
Acknowledgments
TZ acknowledges the China Scholarship Council for a scholarship (CSC No. 201608260012). MR acknowledges support from an ERC Advanced Grant (No. 694368). We thank Gaowen Yang and Guanlin Li for suggestions on the experimental design. We thank Nikita Deoghare and Jenny M. Ospina for their assistance in the experimental setup. We thank Yun Liang for helpful comments on data analysis. We also thank our technicians, Sabine Buchert, Anja Wulf, and Gabriele Erzigkeit, for their help throughout the experiment. We acknowledge support by the Open Access Publication Initiative of Freie Universität Berlin.
References
Adetunji, A. T., Lewu, F. B., Mulidzi, R., and Ncube, B. (2017). The Biological Activities of β-glucosidase, Phosphatase and Urease as Soil Quality Indicators: a Review. J. Soil Sci. Plant Nutr. 17, 794–807. doi:10.4067/S0718-95162017000300018
Anyanwu, I. N., and Semple, K. T. (2016). Assessment of the Effects of Phenanthrene and its Nitrogen Heterocyclic Analogues on Microbial Activity in Soil. SpringerPlus 5, 579. doi:10.1186/s40064-016-1918-x
Bläsing, M., and Amelung, W. (2018). Plastics in Soil: Analytical Methods and Possible Sources. Sci. Total Environ. 612, 422–435. doi:10.1016/j.scitotenv.2017.08.086
Boots, B., Russell, C. W., and Green, D. S. (2019). Effects of Microplastics in Soil Ecosystems: Above and below Ground. Environ. Sci. Technol. 53 (19), 11496–11506. doi:10.1021/acs.est.9b03304
Brennecke, D., Duarte, B., Paiva, F., Caçador, I., and Canning-Clode, J. (2016). Microplastics as Vector for Heavy Metal Contamination from the marine Environment. Estuarine, Coastal Shelf Sci. 178, 189–195. doi:10.1016/j.ecss.2015.12.003
Bronick, C. J., and Lal, R. (2005). Soil Structure and Management: a Review. Geoderma 124, 3–22. doi:10.1016/j.geoderma.2004.03.005
Butterly, C., Baldock, J., and Tang, C. X. (2010). Chemical Mechanisms of Soil pH Change by Agricultural Residues. in 19th World Congress of Soil Science, Soil Solutions for a Changing World conference paper.
Chen, S., and Wu, J. (2019). The Sensitivity of Soil Microbial Respiration Declined Due to Crop Straw Addition but Did Not Depend on the Type of Crop Straw. Environ. Sci. Pollut. Res. 26, 30167–30176. doi:10.1007/s11356-019-06185-9
de Souza Machado, A. A., Lau, C. W., Kloas, W., Bergmann, J., Bachelier, J. B., Faltin, E., et al. (2019). Microplastics Can Change Soil Properties and Affect Plant Performance. Environ. Sci. Technol. 53 (10), 6044–6052. doi:10.1021/acs.est.9b01339
de Souza Machado, A. A., Lau, C. W., Till, J., Kloas, W., Lehmann, A., Becker, R., et al. (2018). Impacts of Microplastics on the Soil Biophysical Environment. Environ. Sci. Technol. 52 (17), 9656–9665. doi:10.1021/acs.est.8b02212
Fei, Y., Huang, S., Zhang, H., Tong, Y., Wen, D., Xia, X., et al. (2020). Response of Soil Enzyme Activities and Bacterial Communities to the Accumulation of Microplastics in an Acid Cropped Soil. Sci. Total Environ. 707, 135634. doi:10.1016/j.scitotenv.2019.135634
Fred-Ahmadu, O. H., Bhagwat, G., Oluyoye, I., Benson, N. U., Ayejuyo, O. O., and Palanisami, T. (2020). Interaction of Chemical Contaminants with Microplastics: Principles and Perspectives. Sci. Total Environ. 706, 135978. doi:10.1016/j.scitotenv.2019.135978
Geyer, R., Jambeck, J. R., and Law, K. L. (2017). Production, Use, and Fate of All Plastics Ever Made. Sci. Adv. 3 (7), e1700782. doi:10.1126/sciadv.1700782
Hargreaves, S. K., and Hofmockel, K. S. (2014). Physiological Shifts in the Microbial Community Drive Changes in Enzyme Activity in a Perennial Agroecosystem. Biogeochemistry 117, 67–79. doi:10.1007/s10533-013-9893-6
Helmberger, M. S., Tiemann, L. K., and Grieshop, M. J. (2020). Towards an Ecology of Soil Microplastics. Funct. Ecol. 34, 550–560. doi:10.1111/1365-2435.13495
Hendershot, W. H., and Lalande, H. (2007). in Soil Reaction and Exchangeable Acidity. Soil Sampling and Methods of Analysis. Editors M. R. Carter, and E. G. Gregorich 2nd Edition (Canadian Society of Soil Science), 173–174. doi:10.1201/9781420005271
Higashida, S., and Takao, K. (1986). Relations between Soil Microbial Activity and Soil Properties in Grassland. Soil Sci. Plant Nutr. 32 (4), 587–597. doi:10.1080/00380768.1986.10557540
Huang, Y., Zhao, Y., Wang, J., Zhang, M., Jia, W., and Qin, X. (2019). LDPE Microplastic Films Alter Microbial Community Composition and Enzymatic Activities in Soil. Environ. Pollut. 254 (PtA), 112983. doi:10.1016/j.envpol.2019.112983
Jackson, C. R., Tyler, H. L., and Millar, J. J. (2013). Determination of Microbial Extracellular Enzyme Activity in Waters, Soils, and Sediments Using High Throughput Microplate Assays. JoVE (80), 50399. doi:10.3791/50399
Jiang, J., Wang, Y.-P., Yu, M., Cao, N., and Yan, J. (2018). Soil Organic Matter Is Important for Acid Buffering and Reducing Aluminum Leaching from Acidic forest Soils. Chem. Geology. 501 (30), 86–94. doi:10.1016/j.chemgeo.2018.10.009
Kim, S. W., Waldman, W. R., Kim, T.-Y., and Rillig, M. C. (2020). Effects of Different Microplastics on Nematodes in the Soil Environment: Tracking the Extractable Additives Using an Ecotoxicological Approach. Environ. Sci. Technol. 54 (21), 13868–13878. doi:10.1021/acs.est.0c04641
Lehmann, A., Leifheit, E. F., Gerdawischke, M., and Rillig, M. C. (2020). Microplastics Have Shape- and Polymer-dependent Effects on Soil Processes. BioRxiv 12, 339–344. doi:10.1101/2020.06.02.130054
Liang, Y., Lehmann, A., Ballhausen, M.-B., Muller, L., and Rillig, M. C. (2019). Increasing Temperature and Microplastic Fibers Jointly Influence Soil Aggregation by Saprobic Fungi. Front. Microbiol. 10, 2018. doi:10.3389/fmicb.2019.02018
Liang, Y., Lehmann, A., Yang, G., Leifheit, E. F., and Rillig, M. C. (2021). Effects of Microplastic Fibers on Soil Aggregation and Enzyme Activities Are Organic Matter Dependent. Front. Environ. Sci. 9, 650155. doi:10.3389/fenvs.2021.650155
Lithner, D., Larsson, Å., and Dave, G. (2011). Environmental and Health hazard Ranking and Assessment of Plastic Polymers Based on Chemical Composition. Sci. Total Environ. 409, 3309–3324. doi:10.1016/j.scitotenv.2011.04.038
Liu, H., Yang, X., Liu, G., Liang, C., Xue, S., Chen, H., et al. (2017). Response of Soil Dissolved Organic Matter to Microplastic Addition in Chinese Loess Soil. Chemosphere 185, 907–917. doi:10.1016/j.chemosphere.2017.07.064
Lozano, Y. M., Aguilar‐Trigueros, C. A., Onandia, G., Maaß, S., Zhao, T., and Rillig, M. C. (2021b). Effects of Microplastics and Drought on Soil Ecosystem Functions and Multifunctionality. J. Appl. Ecol. 58, 988–996. doi:10.1111/1365-2664.13839
Lozano, Y. M., Lehnert, T., Linck, L. T., Lehmann, A., and Rillig, M. C. (2021a). Microplastic Shape, Polymer Type, and Concentration Affect Soil Properties and Plant Biomass. Front. Plant Sci. 12. 616645 doi:10.3389/fpls.2021.616645
Luo, Y., and Zhou, X. (2006). Soil Respiration and the Environment. 1st edition. Norman, Oklahoma: Academic Press.
Mai, L., Bao, L.-J., Wong, C. S., and Zeng, E. Y. (2018). “Microplastics in the Terrestrial Environment,” in Microplastics in the Terrestrial Environment. Microplastic Contamination in Aquatic EnvironmentsEddy Zeng. 1st edition (Elsevier), 365–378. doi:10.1016/B978-0-12-813747-5.00012-6
Pathan, S. I., Arfaioli, P., Bardelli, T., Ceccherini, M. T., Nannipieri, P., and Pietramellara, G. (2020). Soil Pollution from Micro- and Nanoplastic Debris: a Hidden and Unknown Biohazard. Sustainability 12, 7255. doi:10.3390/su12187255
Piehl, S., Leibner, A., Löder, M. G. J., Dris, R., Bogner, C., and Laforsch, C. (2018). Identification and Quantification of Macro- and Microplastics on an Agricultural farmland. Sci. Rep. 8, 17950. doi:10.1038/s41598-018-36172-y
Qi, Y., Ossowicki, A., Yang, X., Huerta Lwanga, E., Dini-Andreote, F., Geissen, V., et al. (2020). Effects of Plastic Mulch Film Residues on Wheat Rhizosphere and Soil Properties. J. Hazard. Mater. 387, 121711. doi:10.1016/j.jhazmat.2019.121711
R Core Team (2020). R: A Language and Environment for Stastical Computing. Vienna, Austria: R Foundation for Statistical Computing. https://www.r-project.org/.
Rillig, M. C., Ingraffia, R., and de Souza Machado, A. A. (2017). Microplastic Incorporation into Soil in Agroecosystems. Front. Plant Sci. 8, 1805. doi:10.3389/fpls.2017.01805
Rillig, M. C., and Lehmann, A. (2020). Microplastic in Terrestrial Ecosystems. Science 368 (6498), 1430–1431. doi:10.1126/science.abb5979
Rillig, M. C., Lehmann, A., Ryo, M., and Bergmann, J. (2019a). Shaping up: Toward Considering the Shape and Form of Pollutants. Environ. Sci. Technol. 53, 7925–7926. doi:10.1021/acs.est.9b03520
Rillig, M. C., Lehmann, A., Souza Machado, A. A., and Yang, G. (2019b). Microplastic Effects on Plants. New Phytol. 223, 1066–1070. doi:10.1111/nph.15794
Rillig, M. C. (2012). Microplastic in Terrestrial Ecosystems and the Soil?. Environ. Sci. Technol. 46 (12), 6453–6454. doi:10.1021/es302011r
Rochman, C. M., Manzano, C., Hentschel, B. T., Simonich, S. L. M., and Hoh, E. (2013). Polystyrene Plastic: a Source and Sink for Polycyclic Aromatic Hydrocarbons in the marine Environment. Environ. Sci. Technol. 47 (24), 13976–13984. doi:10.1021/es403605f
Rousk, J., Bååth, E., Brookes, P. C., Lauber, C. L., Lozupone, C., Caporaso, J. G., et al. (2010). Soil Bacterial and Fungal Communities across a pH Gradient in an Arable Soil. Isme J. 4, 1340–1351. doi:10.1038/ismej.2010.58
Rousk, J., Brookes, P. C., and Bååth, E. (2009). Contrasting Soil pH Effects on Fungal and Bacterial Growth Suggest Functional Redundancy in Carbon Mineralization. Appl. Environ. Microbiol. 75 (6), 1589–1596. doi:10.1128/AEM.02775-08
Scheurer, M., and Bigalke, M. (2018). Microplastics in Swiss Floodplain Soils. Environ. Sci. Technol. 52, 3591–3598. doi:10.1021/acs.est.7b06003
Six, J., Bossuyt, H., Degryze, S., and Denef, K. (2004). A History of Research on the Link between (Micro)aggregates, Soil Biota, and Soil Organic Matter Dynamics. Soil Tillage Res. 79, 7–31. doi:10.1016/j.still.2004.03.008
Teuten, E. L., Saquing, J. M., Knappe, D. R. U., Barlaz, M. A., Jonsson, S., Björn, A., et al. (2009). Transport and Release of Chemicals from Plastics to the Environment and to Wildlife. Phil. Trans. R. Soc. B 364, 2027–2045. doi:10.1098/rstb.2008.0284
Ullah, S., Ai, C., Huang, S., Zhang, J., Jia, L., Ma, J., et al. (2019). The Responses of Extracellular Enzyme Activities and Microbial Community Composition under Nitrogen Addition in an upland Soil. PLoS ONE 14 (9), e0223026. doi:10.1371/journal.pone.0223026
Waldman, W. R., and Rillig, M. C. (2020). Microplastic Research Should Embrace the Complexity of Secondary Particles. Environ. Sci. Technol. 54 (13), 7751–7753. doi:10.1021/acs.est.0c02194
Wan, Y., Wu, C., Xue, Q., and Hui, X. (2019). Effects of Plastic Contamination on Water Evaporation and Desiccation Cracking in Soil. Sci. Total Environ. 654, 576–582. doi:10.1016/j.scitotenv.2018.11.123
Wang, F., Wang, F., and Zeng, E. Y. (2018). “Sorption of Toxic Chemicals on Microplastics,” in Microplastic Contamination in Aquatic EnvironmentsEddy Zeng. 1st edition (Elsevier), 225–247. doi:10.1016/B978-0-12-813747-5.00007-22018
Wang, F., Zhang, X., Zhang, S., Zhang, S., and Sun, Y. (2020). Interactions of Microplastics and Cadmium on Plant Growth and Arbuscular Mycorrhizal Fungal Communities in an Agricultural Soil. Chemosphere 254, 126791. doi:10.1016/j.chemosphere.2020.126791
Wang, Q., He, N., Yu, G., Gao, Y., Wen, X., Wang, R., et al. (2016). Soil Microbial Respiration Rate and Temperature Sensitivity along a north-south forest Transect in Eastern China: Patterns and Influencing Factors. J. Geophys. Res. Biogeosci. 121 (2), 399–410. doi:10.1002/2015JG003217
Wang, R., Dorodnikov, M., Yang, S., Zhang, Y., Filley, T. R., Turco, R. F., et al. (2015). Responses of Enzymatic Activities within Soil Aggregates to 9-year Nitrogen and Water Addition in a Semi-arid Grassland. Soil Biol. Biochem. 81, 159–167. doi:10.1016/j.soilbio.2014.11.015
Wang, W., and Wang, J. (2018). Different Partition of Polycyclic Aromatic Hydrocarbon on Environmental Particulates in Freshwater: Microplastics in Comparison to Natural Sediment. Ecotoxicology Environ. Saf. 147, 648–655. doi:10.1016/j.ecoenv.2017.09.029
Wiedner, K., and Polifka, S. (2020). Effects of Microplastic and Microglass Particles on Soil Microbial Community Structure in an Arable Soil (Chernozem). Soil 6, 315–324. doi:10.5194/soil-6-315-2020
Xue, B., Zhang, L., Li, R., Wang, Y., Guo, J., Yu, K., et al. (2020). Underestimated Microplastic Pollution Derived from Fishery Activities and “Hidden” in Deep Sediment. Environ. Sci. Technol. 54 (4), 2210–2217. doi:10.1021/acs.est.9b04850
Yi, M., Zhou, S., Zhang, L., and Ding, S. (2020). The Effects of Three Different Microplastics on Enzyme Activities and Microbial Communities in Soil. Water Environ. Res. 93 (1), 24–32. doi:10.1002/wer.1327
You, J., Liu, X., Zhang, B., Xie, Z., Hou, Z., and Yang, Z. (2015). Seasonal Changes in Soil Acidity and Related Properties in Ginseng Artificial Bed Soils under a Plastic Shade. J. Ginseng Res. 39 (1), 81–88. doi:10.1016/j.jgr.2014.08.002
Yu, H., Fan, P., Hou, J., Dang, Q., Cui, D., Xi, B., et al. (2020). Inhibitory Effect of Microplastics on Soil Extracellular Enzymatic Activities by Changing Soil Properties and Direct Adsorption: an Inverstigation at the Agregate-Fraction Level. Environ. Pollut. 267, 11554. doi:10.1016/j.envpol.2020.115544
Zhalnina, K., Dias, R., de Quadros, P. D., Davis-Richardson, A., Camargo, F. A. O., Clark, I. M., et al. (2015). Soil pH Determines Microbial Diversity and Composition in the Park Grass Experiment. Microb. Ecol. 69, 395–406. doi:10.1007/s00248-014-0530-2
Zhang, G. S., and Liu, Y. F. (2018). The Distribution of Microplastics in Soil Aggregate Fractions in Southwestern China. Sci. Total Environ. 642, 12–20. doi:10.1016/j.scitotenv.2018.06.004
Zhou, B., Wang, J., Zhang, H., Shi, H., Fei, Y., Huang, S., et al. (2020). Microplastics in Agricultural Soils on the Coastal plain of Hangzhou Bay, east China: Multiple Sources Other Than Plastic Mulching Film. J. Hazard. Mater. 388, 121814. doi:10.1016/j.jhazmat.2019.121814
Keywords: fibers, films, foams, fragments, pH, soil respiration, soil enzymatic activities
Citation: Zhao T, Lozano YM and Rillig MC (2021) Microplastics Increase Soil pH and Decrease Microbial Activities as a Function of Microplastic Shape, Polymer Type, and Exposure Time. Front. Environ. Sci. 9:675803. doi: 10.3389/fenvs.2021.675803
Received: 03 March 2021; Accepted: 11 June 2021;
Published: 23 June 2021.
Edited by:
Christophe Darnault, Clemson University, United StatesReviewed by:
Bahar S. Razavi, University of Kiel, GermanyShaoliang Zhang, Northeast Agricultural University, China
Haibo Zhang, Zhejiang Agriculture and Forestry University, China
Copyright © 2021 Zhao, Lozano and Rillig. This is an open-access article distributed under the terms of the Creative Commons Attribution License (CC BY). The use, distribution or reproduction in other forums is permitted, provided the original author(s) and the copyright owner(s) are credited and that the original publication in this journal is cited, in accordance with accepted academic practice. No use, distribution or reproduction is permitted which does not comply with these terms.
*Correspondence: Yudi M. Lozano, eXVkeWphQGdtYWlsLmNvbQ==
†ORCID: Yudi M. Lozano, orcid.org/0000-0002-0967-8219; Matthias C. Rillig, orcid.org/0000-0003-3541-7853