- 1Faculty of Geosciences and Environmental Engineering, Southwest Jiaotong University, Chengdu, China
- 2Institute of Hydrogeology and Environmental Geology, Chinese Academy of Geological Science, Shijiazhuang, China
- 3Yibin Research Institute, Southwest Jiaotong University, Yibin, China
- 4School of Water Resources and Environment, Hebei GEO University, Shijiazhuang, China
Phreatic groundwater plays a significant role in the water supply for human communities, especially in water scarce regions. This research was conducted to investigate the hydrochemistry and contamination of phreatic groundwater and their constraints on drinking water supply in a semiarid region of the North China Plain. A total of 49 phreatic groundwaters were collected for the hydrochemical analysis. The results suggested the phreatic groundwater had a slightly alkaline nature. Although the groundwater had a large variation of salinity with the TDS of 172–1,875 mg/l, most groundwater was moderately-hard fresh and of HCO3-Ca type. Contaminants of NO3−, NO2−, NH4+, Fe, F−, Zn, Mn were detected, but only NO3−, NO2−, NH4+, and Fe were found exceeding the drinking water desirable limits at some sites. The nitrogen contaminants were from anthropogenic inputs (urban sewage effluents and agricultural fertilizers) and mainly distributed in the central study area. The anthropogenic inputs of nitrogen contaminants also elevated groundwater salinity. Among the exceeding contaminants, only NO3− would pose a potential health risk to water consumers. The existing health risks by NO3− ranged from medium risk (HQ of 1–4) to high risk (hazard quotient > 4) for all populations, and most risks were categorized into the medium risk rank. Groundwater F− was also found with health risk at some sporadic sites although it did not exceed, only approached, the desirable limit. Its health risk was only to infants and categorized into the medium risk with the maximum HQ of 1.55. Most phreatic groundwater (83.67%) in the study area had relatively good quality and was suitable for domestic usage with the EWQI value below 150. Others belonged to the poor (EWQI of 150–200) and extremely poor (EWQI > 200) quality categories, and mainly distributed in the urban Gaocheng. Thus, it is recommended to pay attentions to the anthropogenic contaminants inputs into phreatic aquifers in human activities areas.
Introduction
Surface water resources have poor capabilities against global climate change in recent years, and as a result, presents a short supply performance (Li J. et al., 2020). Because of the wide distribution and easy accessibility, groundwater resources have become the dominant and even only water resource supplying human society development (Gu et al., 2018; Adimalla et al., 2020; Vaiphei et al., 2020; Rahimi-Feyzabad et al., 2021). This is more significant in arid and semiarid regions due to the scarcity of surface water and precipitation (Hao et al., 2018; Xiao et al., 2018; Wang Q. et al., 2020; Herrera et al., 2021; Wang et al., 2021).
The availability of groundwater resources is not only determined by the quantity but also restricted by the quality (Satheeskumar et al., 2020). Concerns on groundwater chemistry and quality have been raised worldwide due to the worsening quality of groundwater in recent decades (Li et al., 2019; Alqahtani et al., 2020; Keesari et al., 2020; Liu et al., 2020). The poor quality of groundwater would greatly constrain the suitability of subsurface water for various uses by human society (Rao et al., 2013; Li P. et al., 2018; Qu et al., 2021a). Especially, some elements (such as fluoride, arsenic, nitrate, and heavy metals) in groundwater would pose adverse effects on the health of water consumers and induce many water-borne diseases like fluorosis, diabetes, cholera, dysentery, etc. (Adimalla, 2020; He et al., 2021). Comprehensive understanding of groundwater chemistry and quality status is the critical prerequisite for human society accessing clean and safe water (Marghade et al., 2021).
In fact, groundwater hydrochemical components are generally originated from geogenic origins and can be also from anthropogenic sources (Reynolds and Johnson, 2019; Gao et al., 2020). The hydrochemical composition of natural groundwater is governed by many factors including recharge water, rock/medium types, and hydrochemical processes along the flow paths (Helena et al., 2000; Venkatramanan et al., 2017). In natural conditions, some substances such as fluoride, arsenic, and iodine may release from the rocks/mediums into groundwater and result in geogenic poor quality groundwater (Liu et al., 2015; Zhang et al., 2018; Zhi et al., 2019; Wang Y. et al., 2020). Natural evaporation maybe significantly strong and lead to the salinity of groundwater, especially in an arid environment (Li C. et al., 2020). Furtherly, various human activities would potentially alter the circumstance of groundwater, as a result, modify the hydrochemical composition of groundwater (Gao et al., 2020; Solgi and Jalili, 2021). This could be implemented by indirect disturbance on natural hydrochemical processes and also direct contaminant inputs (Xue et al., 2019; Li C. et al., 2020; Xiao et al., 2022). With the rapid development of human society and dramatic climate change, groundwater quality has undergone more and more pressure (Houéménou et al., 2020). Groundwater contamination has become a major challenge faced by many regions where people rely on groundwater resources for water supply (Gu et al., 2017; Li Z. et al., 2018; Gu et al., 2018; Yin et al., 2019; Adimalla et al., 2020; Satheeskumar et al., 2020; Qu et al., 2021b; Li et al., 2021; Marghade et al., 2021).
Compared with the groundwater in confined aquifers (i.e., confined groundwater), that in the unconfined aquifers (i.e., phreatic groundwater) is the more favored and main exploited groundwater resources by human community, especially in rural area, due to its cheaper exploitation cost (Zhao et al., 2021) and better renewal capability. A great many researchers have argued that phreatic groundwater could be threatened by contaminants from both geogenic and anthropogenic sources (Rajmohan and Prathapar, 2016; Ali et al., 2019; Reynolds and Johnson, 2019; Li C. et al., 2020; Alqahtani et al., 2020). For example, Su et al. (2021) studied the fluoride-enriched groundwater in the Tarim Basin, and found the unconfined aquifers in both the piedmont plain and alluvial plain were with geogenic high fluoride groundwater. Ali et al. (2019) obtained similar conclusions for the fluoride contaminants in phreatic groundwater, and also pointed out that the arid climatic conditions may potentially aggravate the geogenic fluoride contamination through promoting the evaporation process, etc. Other minor elements such as arsenic, iodine, and heavy metals in phreatic aquifers were also reported as being able to originate from geogenic sources and be regulated by external factors (Taylor, 1996; Arslan et al., 2017; Voutchkova et al., 2017; Zeng et al., 2018; Zhi et al., 2019; Duan et al., 2020). With the rapid development of industry, agriculture, and urbanization, groundwater contamination induced by human activities has become more and more severe in recent decades (Baalousha, 2011; Souid et al., 2017; Houéménou et al., 2020). In addition, the shallow buried feature of phreatic groundwater makes it more prone to be impacted on its hydrochemical quality by external factors than confined groundwater (Inglis et al., 1986; Okkonen and Kløve, 2012; Rajmohan and Prathapar, 2016; Almanza Tovar et al., 2020). Thus, more attention should be paid to the hydrochemistry features and quality of phreatic groundwater in water shortage regions to safeguard the health of residents.
The North China Plain (NCP) is one of the largest alluvial plains in Asia, and also one of the most important socio-economic and agricultural regions in China (Dun et al., 2014). Many cities are located in the piedmont regions of the NCP and rely on phreatic groundwater resources for various purposes. The safety of groundwater is significant for the sustainability of the area. While due to the densely agricultural practices and rapid urbanization in recent decades, the availability of groundwater is challenged in terms of the hydrochemical quality (Li et al., 2016; Hao et al., 2020; Zhou et al., 2020). This research focuses on the contamination and hydrogeochemical accessibility of phreatic groundwater in a typical area from the piedmont of North China Plain. The main objectives of the present research are to 1) characterize the physicochemical features and contamination of phreatic groundwater, 2) understand the origin of groundwater contaminants and regulating hydrochemical processes, 3) assess the health threats of aqueous contaminants to residents, 4) estimate the overall hydrochemical quality of groundwater for domestic purposes. The present study will provide a better understanding of hydrochemistry, quality, and genesis of phreatic groundwater in arid and semiarid regions, and will be definitely helpful for rational phreatic groundwater resource management and exploitation.
Materials and Methods
Study Site
The Gaocheng-Wuji region is situated in the central part of Shijiazhuang, North China Plain (NCP) (Figure 1). It extends between 37°51′21.29″N and 38°18′39″N in latitudes and from 114°8′33″E to 115°8′30″E in longitudes, with a covering area of ∼1,296 km2. Geomorphologically, this area belongs to the piedmont plain of the NCP. This region belongs to the semiarid continental climate. The average annual rainfall approximates 500 mm and more than 50% occurs in July and August. There are three rivers, namely Hutuo River, Ci River, and Wangyang River, running through the study area from the west towards the east (Figure 1C). While rivers in this area usually dry up due to the reservoirs construction in the upstream, resulting in surface water scarcity. Groundwater plays an irreplaceable role in water supply for human society in this area. The land use type in the study area is dominantly separated into three types, i.e., agricultural land, urban land, and industrial land. The urban lands are mainly distributed in the central area with the name of Gaocheng and in the northeast with the name of Wuji (Figure 1C). Industrial lands are concentratedly distributed in the west, namely the economic development zone of Shijiazhuang. Besides, there are many sporadic towns and villages distributing in the whole study area. However, agricultural land is the most dominant land use type and with a long history of agriculture and fertilizer practices.
Geologically, the present study area mainly belongs to the Hutuo river alluvial fan and is comprised of Quaternary alluvial deposits with the depth of 150–300 m (Cheng et al., 2017). These deposits were formed during the Pleistocene and Holocene with the lithology of pebble, gravel, coarse sand, sand, and fine sand. Thus, aquifers in this area are mainly unconfined with relatively high hydraulic conductivity (K = 27–70 m/day) (Chen et al., 2006) and it is easy for water and contaminants to move horizontally and vertically. Groundwater is recharged vertically by precipitation, irrigation water, and surface water seepage and horizontally by lateral flow in, and discharged mainly through anthropogenic pumping and lateral flow out. Regionally, groundwater flows from the northwest to the southeast (Li et al., 2016).
Sample Collection and Data Generation
A total of 49 samples were collected from the phreatic aquifers across the study area (Figure 1C) from July to August in 2018. The sampling sites were selected based on the availability and even spatial distribution of boreholes/wells. All groundwater samples were obtained from boreholes/wells with depths in the range of 60–120 m. Boreholes and wells were pumped for more than 15 min to remove the stagnant water before conducting sampling strategies. The pH and electrical conductivity (EC) of target groundwater were monitored in this process, and the sampling was performed until these two in-situ physicochemical parameters became stable. Clean high-density polyethylene bottles were prewashed using the target groundwater in the field and used for groundwater sampling. All samples were stored under the condition of 4°C with the aid of incubators. Samples were sent to the laboratory of groundwater sciences and engineering of the institute of hydrogeology and environmental geology, Chinese Academy of Geological Sciences for hydrochemical analysis.
In-situ parameters of groundwater including water temperature (T), pH, EC were measured in the field using a portable device (Multi 350i/SET, Munich, Germany). Trace elements (Zn, Fe, Mn) and major cations (K+, Na+, Ca2+, Mg2+) were measured by inductively coupled plasma-mass spectrometry (Agilent 7500ce ICP-MS, Tokyo, Japan). NH4+ and anions of SO42−, Cl−, NO3−, NO2−, F− were determined using ion chromatography (Shimadzu LC-10ADvp, Kyoto, Japan). Acid–base titration was performed for analyzing HCO3− of groundwater. The quality assurance of analyzed results was ensured by checking the consistency and accuracy of hydrochemical analysis. The consistency of analyzed parameters were checked by conducting triplicate analyses. The accuracy of laboratory analysis was checked using the ionic charge balance error (ICBE) percentage using Eq. 1. All samples were with the ICBE within ±5%, suggesting the analysis results of all groundwaters were reasonably good.
Where
Human Health Risk Assessment
The potential hazards of aqueous contaminants in groundwater can be quantitatively evaluated with the aid of human health risk assessment (HHRA) model established by the United States Environmental Protection Agency (USEPA, 1989). Although contaminants can threaten the health of consumers through many pathways such as inhalation, dermal contact, and oral intake, the oral pathway is evidenced to be the harmful one (Yin et al., 2020). Therefore, the non-carcinogenic risk of contaminants via the oral pathway was the concern of the present study. Four populations were divided and considered according to the age and gender, i.e., infants (0–6 months), children (7 months–17 years), adult females (≥18 years) and adult males (≥18 years).
The non-carcinogenic risk posed by a specific aqueous contaminant can be expressed by the hazard quotient (HQ) and calculated as follows:
Where, CDI represents the chronic daily ingestion dose of the concerned contaminant via oral pathway; C refers to the aqueous contaminant concentration; IR is the water oral ingestion rate; ED and EF express the duration and exposure frequency, respectively; AT denotes the average exposure time; BW signifies the average body weight of concerned population; RfD is the reference dosage via oral pathway. Parameters that used in the HHRA were presented in Table 1 as below:
Entropy-Weighted Water Quality Index
The quality of groundwater can be comprehensively understood with the aid of water quality index (WQI). It has been widely used over the world and proved to be robust and effective. However, its accuracy heavily relies on the weights assigned to each physicochemical parameter involved in the WQI appraisal. The entropy-weighted water quality index (EWQI) method introduces the information entropy to objectively assign the weights and can greatly improve the accuracy of assessment results (Xiao et al., 2020).
For the EWQI procedure, firstly, the eigenvalue matrix “X” should be constructed using “m” groundwater samples and “n” physicochemical parameters (Eq. 5). Secondly, the “X” is standardized to “Y” (Eq. 6) using the normalizing function (Eq. 7). Thirdly, the entropy weight “wj” is calculated (Eqs 8–10). Fourthly, the rating scale is quantitatively determined (Eq. 11). Finally, the EWQI value is computed (Eq. 12).
Where, “i” and “j” denote the sample no. and index no., respectively; “ej” represnts the information entropy; “Cj” and “Sj” are the measured physicochemical parameter value and the drinking water standard of the physicochemical parameter, respectively.
The quality of groundwater can be classified into five categories, i.e. Rank 1: Excellent quality (EWQI < 50), Rank 2: Good quality (EWQI, 50–100), Rank 3: Medium quality (EWQI, 100–150), Rank 4: Poor quality (EWQI, 150–200), Rank 5: Extremely poor quality (EWQI, >200) (Amiri et al., 2014; Luo et al., 2021).
Results
Major Physicochemical Composition of Groundwater
The physicochemical parameters of groundwater can provide preliminary insights into the overall geochemical characteristics of sampled groundwaters and have been statistically presented in Table 2. The related desirable limits recommended by the Chinese guidelines (GAQS, 2017) and the World Health Organization (WHO, 2017) were also listed in the table for comparison.
The sampled phreatic groundwaters in the study were with the temperature ranging from 14.1°C to 22.5°C, averaging at 16.7°C, which was very close to the annual average air temperature of 14.2°C in the study area. The pH of groundwater ranged from 7.11 to 8.07 with an average of 7.52 (Table 2), demonstrating a slightly alkaline nature. All sampled groundwaters had the pH value within the desirable limit range of 6.5–8.5 recommended by Chinese Guidelines for drinking purposes. The EC values were in the range of 329–2,224 μS/cm with a mean of 851 μS/cm. The TDS varied from 172–1,875 mg/l and averages at 510 mg/l. Both of EC and TDS suggested the phreatic groundwater in the study area had a relatively large variation of salinity. The total hardness of sampled groundwater ranged from 100 to 1,166 mg/l with an average of 356 mg/l. As shown in Figure 2A, the sampled groundwaters in the study area were predominantly moderately-hard fresh water, with a small portion of soft fresh, hard fresh, very-hard fresh and very-hard brackish water.
The concentration of major cations and anions was also in relatively wide range (Table 2). Ca2+ is the dominant cation in the phreatic groundwater (Figure 2B) with its concentration in the range of 30.29–280.70 mg/l and averaging at 85.7 mg/l. Na+ and Mg2+ ranked the second and third in the major cations, with the concentration varying from 12.63 to 185.80 mg/l (a mean of 40.05 mg/l) and between 5.98 and 113.00 mg/l (a mean of 34.54 mg/l), respectively. While the K+ was the least abundant ion in major cations and ranged from 1.14 to 12.24 mg/l with an average of 2.52 mg/l. For major anions, HCO3− ranked the first, and followed by SO42− and Cl− (Figure 2B). The concentration of major anions was in the range of 158.00–693.10 mg/l with an average of 288.52 mg/l for HCO3−, 4.81–459.00 mg/l with a mean of 81.98 mg/l for SO42−, 5.25–570.40 mg/l with an average of 67.86 mg/l for Cl−. The hydrogeochemical facies of phreatic groundwater were dominated by HCO3-Ca type, and only a small portion belonged to Mixed Cl-Mg·Ca, Mixed HCO3-Na·Ca and Cl-Ca type (Figure 2B), demonstrating an overall fresh nature for phreatic groundwater in the study area. The relatively salty groundwaters are mainly distributed in the middle area of the study area along the Hutuo River, extremely in the urban Gaocheng (Figure 3A).
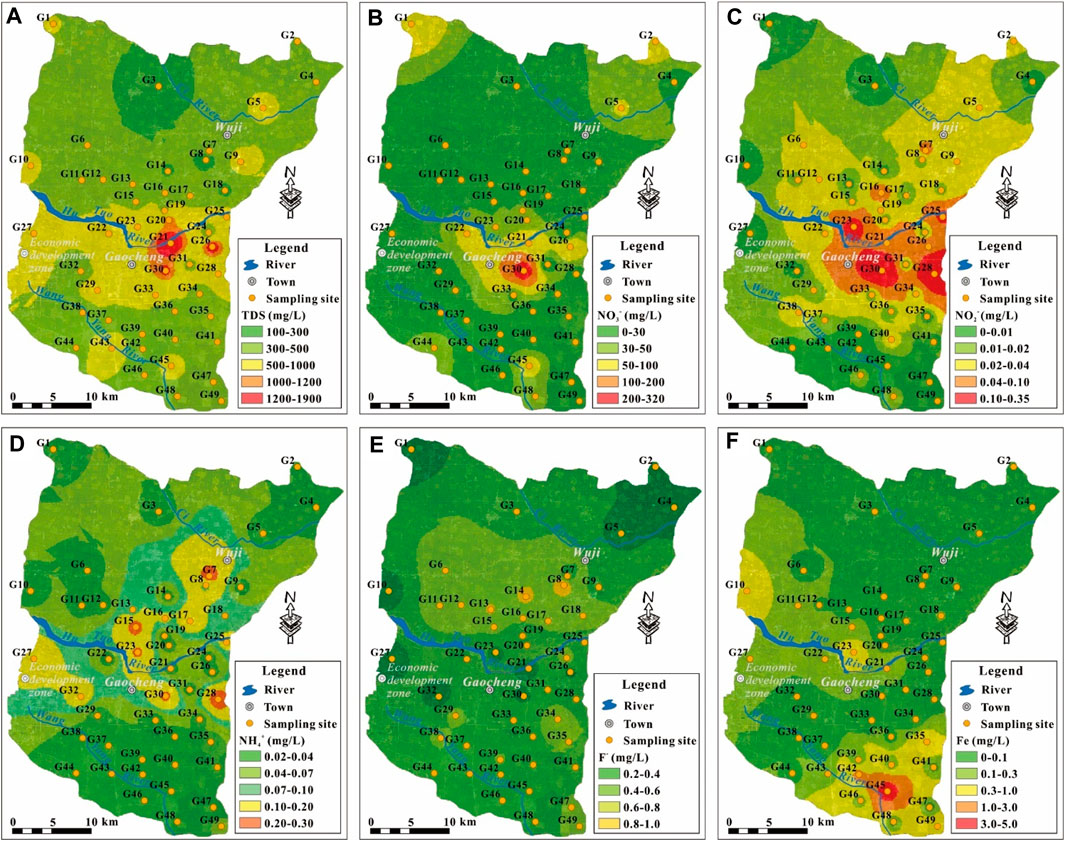
FIGURE 3. Geospatial distribution of (A) TDS, (B) NO3−, (C) NO2−, (D) NH4+, (E) F, (F) Fe of groundwater in the study area.
Groundwater Contaminants and Geospatial Distribution
Elements (including nitrogen, fluoride and heavy metals) that may threat the quality of water supply were detected. Most of these elements were found with very low concentrations and even below the detection limits. The detected elements were summarized in Table 2.
Nitrogen is the common and widely distributed contaminant in groundwater across the world, and also found with relative high concentration for its three forms, i.e., NO3−, NO2−, NH4+, in the study area. The concentration of NO3− was in a significant large range, and varied from 2.00 mg/l to 313.40 mg/l with an average of 30.23 mg/l. Although the mean concentration of NO3− in the phreatic aquifer was far below the desirable limit of 50 mg/l recommended by WHO (2017) and most groundwaters (83.67%) were within this limit, some groundwaters were detected with extremely high NO3− concentration (up to 313.40 mg/l, more than 6 times of the recommended desirable limit) yet. The concentration of NO2− and NH4+ ranged from 0.001 to 0.320 mg/l and from 0.02 to 0.30 mg/l, respectively, averaging at 0.033 and 0.06 mg/l. About 28.57 and 10.20% of groundwaters were found with the NO2− and NH4+ concentration exceeding the drinking water desirable limits (Table 2). The high NO3− groundwaters were dominantly distributed in the urban Gaocheng (Figure 3B), and the high NO2− groundwaters distributed in the area from the urban Gaocheng to the urban Wuji (Figure 3C). The exceeding NH4+ in groundwater is sporadic in spatial (Figure 3D).
Fluoride is a common contaminant in groundwater. Its concentration varied from 0.22 to 0.99 mg/l with an average of 0.54 mg/l in phreatic groundwater in the study area. All groundwaters are with the F− concentration within the desirable limit of 1 mg/l recommended by WHO (2017). But it should be noted that the maximum concentration of F− in sampled groundwater approximated the desirable limit. As shown in Figure 3E, the phreatic groundwater was with the F− contaminant in a relatively low level, only very limited sites were observed with the F− concentration approximating the desirable limit. For heavy metals, only Fe was found beyond the drinking water desirable limit, while others were all within the desirable limits (Table 2). The concentration of Fe in phreatic groundwater varied between 0.005 and 4.980 mg/l with an average of 0.230 mg/l, and about 14.29% of sampled groundwater exceeded the desirable limit of 0.3 mg/l.
Discussion
Formation Mechanisms of Groundwater Chemistry
The hydrogeochemical components of groundwater can originate from various sources and be regulated by many processes (Rajmohan and Prathapar, 2016; Gao et al., 2020; Satheeskumar et al., 2020; Luo et al., 2021). Natural processes, usually in three categories of precipitation, rock, and evaporation dominance, lay the foundation of groundwater hydrochemical composition. Gibbs diagrams, i.e. the cation weight ratio of Na+/(Na++Ca2+) and Cl−/(Cl− + HCO3−) versus TDS and anion weight ratio of Cl−/(Cl− + HCO3−) versus TDS (Gibbs, 1970), are effective tools to visibly reveal the main processes governing groundwater chemistry in nature and have been widely used worldwide (Xiao et al., 2018; Luo et al., 2021; Xiao et al., 2021). As demonstrated in Figure 4, all sampled groundwaters were plotted in the rock dominance rather than precipitation and evaporation dominance, suggesting that the hydrochemistry of phreatic groundwater in the study area is significantly and dominantly controlled by water-rock interaction and out of the influence of evaporation.
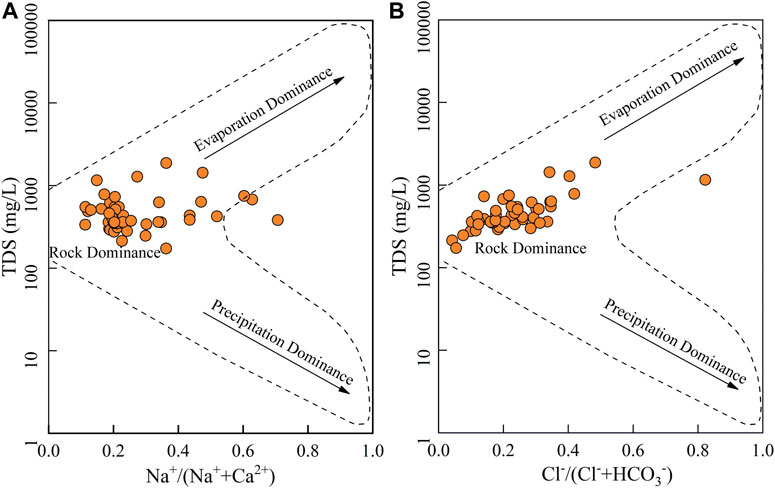
FIGURE 4. Gibbs diagrams illustrating the natural processes controlling the chemical compositions of phreatic groundwater in the study area.
For further illustrate the water-rock interaction processes, the end-member diagrams were employed to constrain the specific rocks involved in these natural mechanisms. Three main categories of rocks, namely evaporates, silicates and carbonates, can be identified from the end-member diagrams of Mg2+/Na+ versus Ca2+/Na+ and HCO3−/Na+ versus HCO3−/Na+ (Venkatramanan et al., 2017; Adeyeye et al., 2021; Xiao et al., 2021). As demonstrated in Figure 5, the phreatic groundwaters were dominantly situated in the silicates dominance and the transition between silicates and carbonates, implying silicates weathering and carbonates dissolution were the main sources of ionic substances for phreatic groundwater in the study area. In addition, a few groundwaters were observed plotting in the transition between silicates and evaporites, demonstrating that evaporites dissolution also contributed to the hydrochemical components of phreatic groundwater at some sites to some degree.
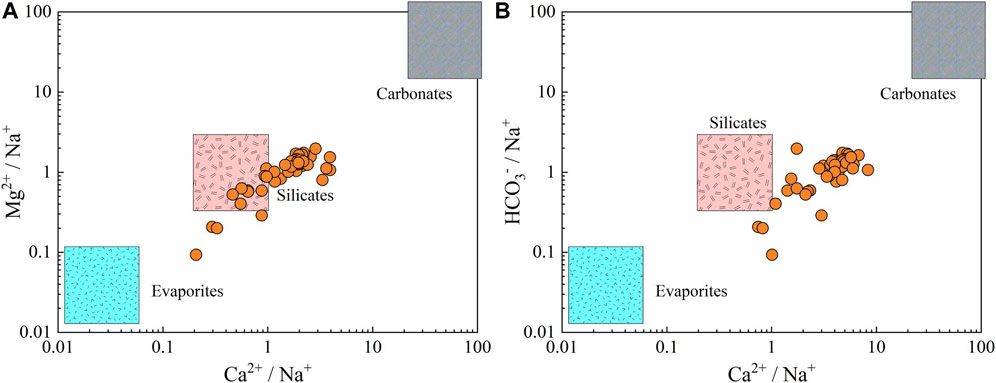
FIGURE 5. Groundwater end-member diagrams constructed by (A) Mg2+/Na+ versus Ca2+/Na+ and (B) HCO3−/Na+ versus Ca2+/Na+.
The correlation matrix and saturation status modeling were employed to further illustrate the contribution of specific minerals to the natural chemical components of groundwater. As shown in Table 3, a positive relation coefficient was observed between Na+ and Cl−, indicating halite dissolution (NaCl) was the potential source of Na+ and Cl− in groundwater. This was confirmed by the halite saturation index computing results (SI < 0, implying unsaturation) presented in Figure 6. But it should be noted that its relation coefficient was below the significant standard of 0.5, suggesting limited contribution from the halite dissolution. This was coincided with the conclusion implied by end-member diagrams (Figure 5). While SO42− was observed having significant positive relation with Na+ (relation coefficient = 0.89), Ca2+ (relation coefficient = 0.71) and Mg2+ (relation coefficient = 0.69), indicating the dissolution of sulfate minerals (Na2SO4, CaSO4, CaSO4·2H2O, MgSO4, etc.) were the dominant process contributing to the chemical components of groundwater. These processes were confirmed by the saturation index (SI) results that all sampled groundwater were under unsaturated states (SI < 0) of sulfate minerals (such as anhydrite, gypsum, etc.) (Figure 6). Additionally, significant positive relations were observed between HCO3− and Ca2+ (relation coefficient = 0.70), Mg2+ (relation coefficient = 0.76), suggesting carbonate minerals including calcite, aragonite, dolomite were potential natural sources of groundwater chemical components (Table 3). While the saturation index results showed that only part of groundwaters were under unsaturated status of carbonate minerals, thus, the dissolution of calcite, aragonite, dolomite was the dominant processes contributing hydrochemical components of part groundwaters rather than all groundwaters. The contribution of carbonate dissolution is especially significant for groundwaters that with low TDS (Figure 6).
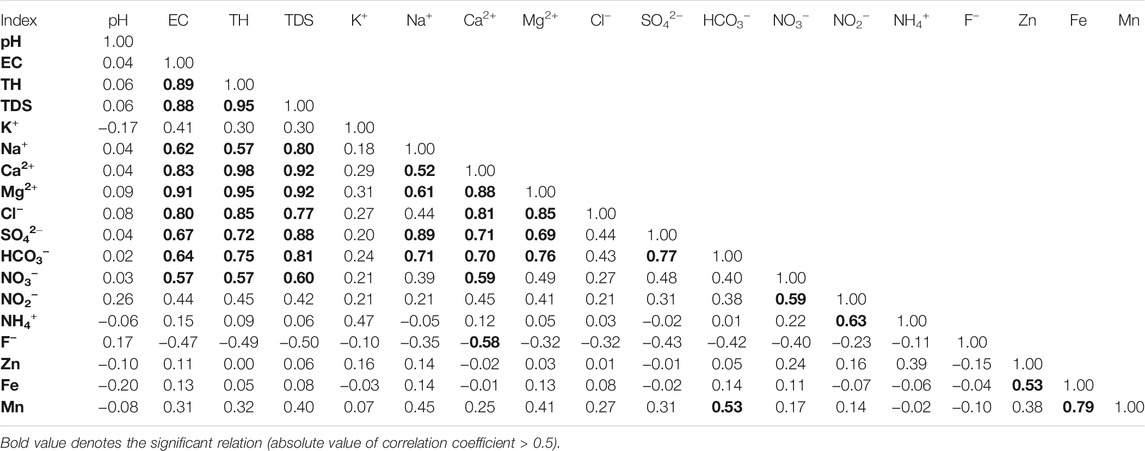
TABLE 3. The correlation matrix between various hydrochemical parameters of phreatic groundwater in the study area.
NO3− is a common contaminant for human society. Its natural limit is 10 mg/l. Any groundwater with NO3− concentration beyond this limit implies being impacted by anthropogenic contamination. The molar ratio diagram of NO3−/Na+ versus SO42−/Ca2+ can demonstrate a detailed picture of the source of anthropogenic contaminants (Gao et al., 2021). As shown in Figure 7A, groundwaters at G1, G2, G5, G26, G30, and G45 were presented being contaminated by nitrate-containing fertilizers or domestic wastewater. It can be clearly seen that except G45 situated in the agricultural land, the other 5 groundwaters are located in the human settlements, suggesting that the NO3− in groundwater at G1, G2, G5, G26, G30 originated from domestic wastewater, while that at G45 was from nitrate-containing fertilizers. The correlation matrix suggested that NO3− in groundwater had significant positive relation with EC, TH and TDS (Table 3), indicating the inputs of anthropogenic NO3− also brought some other chemical components (such as Ca2+, Mg2+, SO42−, etc.) and increased the salinity of groundwater. This was evidenced by the similar distribution of high TDS groundwater (Figure 3A) and high NO3− groundwater (Figure 3B). Besides, a significant positive relationship was also observed between NO3− and NO2− and between NO2− and NH4+, implying the existence of nitrosification and nitrification processes that transformed the NH4+ into NO2− and finally into NO3−. This conforms to the real condition of unsaturated zone and phreatic aquifers that with relatively good permeability and rich oxygen. Figure 7A also suggested groundwater at G29 and G43 were impacted by sulfur-containing fertilizers or industrial wastewater. Considering their agricultural land use type (Figure 1C), groundwater at these two sites were contaminated by the sulfur-containing fertilizers rather than industrial wastewater. Groundwaters at G29, G43 were found with higher TDS than background value, thus, the inputs of sulfur-containing fertilizers contaminants also increased the salinity of groundwater.
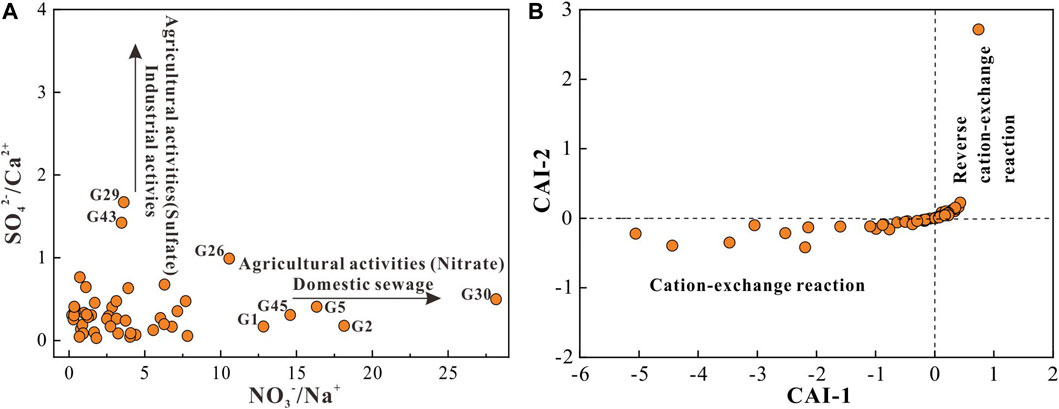
FIGURE 7. Plots of (A) molar ratio NO3−/Na+ versus molar ratio SO42−/Ca2+ and (B) CAI-1 versus CAI-2 for phreatic groundwater in the study area.
Ion exchange is usually one of the important natural processes regulating groundwater chemistry. It is especially significant for aquifers that with fine mediums. These kinds of hydrochemical processes can be clearly revealed by the chloro-alkaline indices including CAI-1 (Eq. 12) and CAI-2 (Eq. 13).
If the aqueous Ca2+ or Mg2+ in groundwater exchanges the absorbed Na+ or K+ on sediments, i.e., cation-exchange reaction, the aforementioned two chloro-alkaline indices would be negative. While if the aqueous Na+ or K+ in groundwater exchanges the absorbed Ca2+ or Mg2+ on sediments, i.e., reverse cation-exchange reaction, both CAI-1 and CAI-2 are expected to be positive. Generally, cation-exchange reaction occurs in the groundwater with relatively low TDS and benefits groundwater mineralization. On the contrary, reverse cation-exchange reaction is highly possible to occur in the high TDS groundwater, in which the rich aqueous Na+ in groundwater tends to exchange Ca2+ or Mg2+ on sediments. As demonstrated in Figure 7B, most sampled groundwaters were with the two chloro-alkaline indices below 0, indicating that cation-exchange reaction was the dominant hydrochemical process and favored the enrichment of Na+ and K+ in groundwater. Additionally, a part of sampled groundwater was observed having the positive CAI-1 and CAI-2 value, implying occurred reverse cation-exchange reaction for these groundwaters. According to the statistics, groundwaters with negative chloro-alkaline indices had the TDS of 447 mg/l on average, and that with positive chloro-alkaline indices were with the TDS of 566 mg/l in average, suggesting the elevation of TDS had led to the ion exchange process transformed from cation-exchange to reverse cation-exchange for phreatic groundwater in the study area.
For the minor elements of F−, Zn, Fe, Mn, no significant relations were observed between them and the nitrogen contaminants (Table 3), indicating that they were not originated from the nitrogen-containing anthropogenic contamination. As discussed before, Zn and Mn were all in relatively low concentration and far below the guideline limits, thus, it is highly possible they originated from the natural dissolution of Zn-bearing and Mn-bearing minerals. This was confirmed by the simulation results of the saturation index that all Zn-bearing and Mn-bearing minerals were under unsaturated states for sampled phreatic groundwaters. For F− contaminant, its concentration in all sampled groundwaters were within the guideline limit of 1 mg/l (Table 2). Although it presented a relatively high concentration (approaching 1 mg/l) sporadically in the study area (Figure 3E), no relation was observed between it and high salinity/other contaminants in terms to the spatial distribution and statistics. Consequently, it was highly possible that the F− contaminant in groundwater may have come from the natural release of fluoride-bearing minerals. This was also evidenced by the saturation indexes simulation presented in Figure 6. For Fe in groundwater, it has no significant relationship with the salinity and nitrogen contaminants (Table 3), indicating it was not from the anthropogenic source. The saturation index simulation showed all Fe-bearing minerals were under unsaturated status and can potentially contribute aqueous Fe to groundwater if there are existing Fe-bearing minerals. Thus, the sporadic high Fe in groundwater of the study area were from the release of natural Fe-bearing minerals.
Appraisal of Groundwater Quality for Domestic Purposes
Health Threats From Groundwater Contaminants
The contaminants, such as nitrogen, fluoride, and heavy metals, in drinking water would potentially threaten the health of consumers, thus, should be a concern when found in water supply. In the present study, all exceeding contaminants (i.e., NO3−, NO2−, NH4+, Fe) and other detected contaminants including F− and heavy metals of Zn, Mn were included in the human health risk assessment.
The hazard quotient (HQ) results of various contaminants were statistically presented in Figure 8. The recommended safe limit of HQ for non-carcinogenic health risk is 1 by USEPA (1989). Any HQ value greater than 1 is regarded existing potential non-carcinogenic health threat, while that less than 1 implies as safe. It can be clearly seen that the HQ values of NO2−, NH4+, Zn, Fe, Mn were all below the safe limit of 1 for all populations (Figure 8), indicating negligible chronic risk. As discussed before, three of these contaminants, i.e., NO2−, NH4+ and Fe, were found beyond the desirable limits of drinking purpose (Table 2) in parts of the study area, but their low HQ values implied negligible non-carcinogenic health risk when serving as drinking water. While the HQ value of NO3− and F− were observed exceeding the permissible limit of 1 at some locations. For NO3− contaminant, the HQ value varied from 0.12 to 18.35 (mean = 1.77) for infants, between 0.07 and 11.34 (mean = 1.09) for children, from 0.05 to 8.14 (mean = 0.79) for adult females, between 0.06 and 9.71 (mean = 0.94) for adult males. Accordingly, the health of all populations would be potentially threatened by the high NO3− contaminant. The box plots (Figure 8A) demonstrated that the susceptibility of population to NO3− contaminant was in the order of Infants > Children > Adult males > Adult females. The HQ value of F− contaminant was in the range of 0.34–1.55 (mean = 0.84) for infants, 0.21–0.96 (mean = 0.52) for children, 0.15–0.69 (mean = 0.37) for adult females, 0.18–0.82 (mean = 0.45) for adult males, indicating the aqueous F− contaminant would pose potential health threats to infants at some locations, but not to other three populations. It should be noted that all sampled groundwaters in the study area were with the F− concentration within the desirable limit of drinking purpose, but still existing potential health risk to infants. Thus, attentions should be paid to the potential health risk to infants posed by relatively high F− contaminant even within desirable limit of drinking purpose.
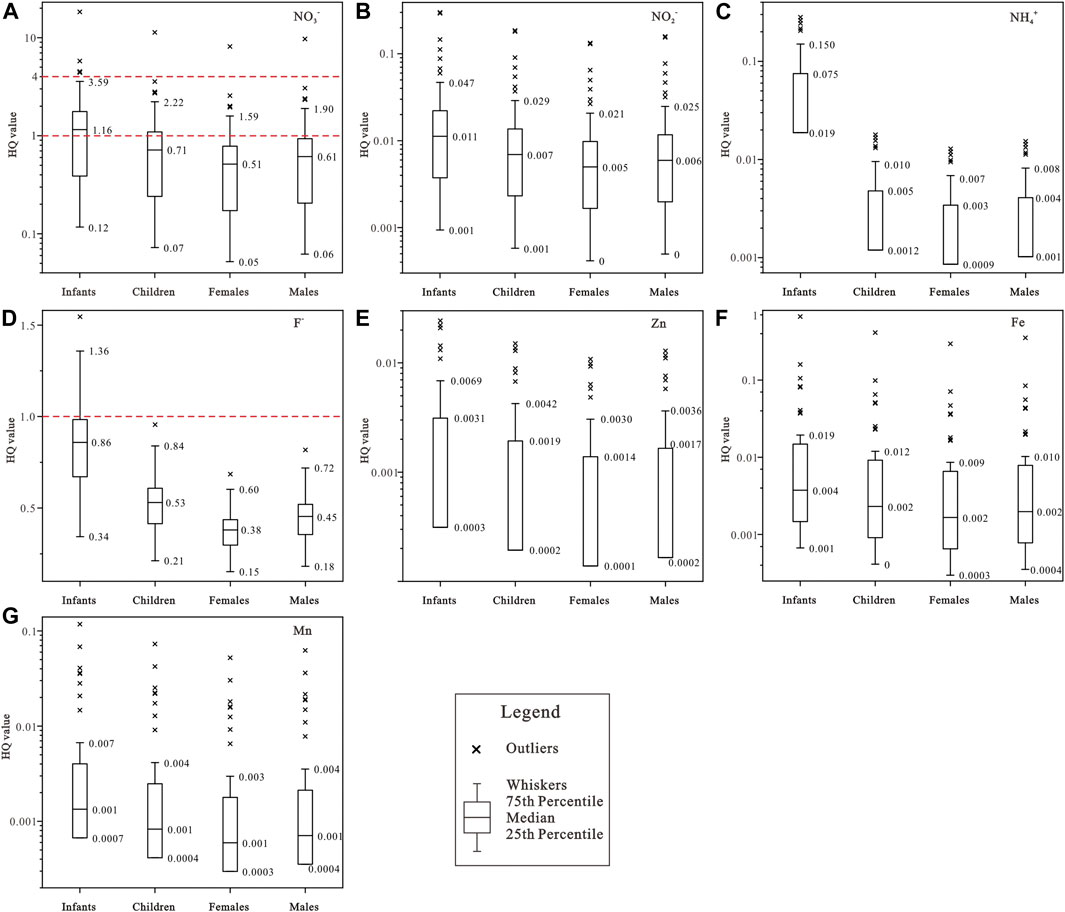
FIGURE 8. Box plots of the hazard quotient of noncarcinogenic risk posed by (A) NO3−, (B) NO2−, (C) NH4+, (D) F−, (E) Zn, (F) Fe, (G) Mn via oral pathway.
The potential non-carcinogenic health risks from NO3− and F− contaminant in spatial were presented Figure 9. It can be seen that the NO3− risk mainly distributed in the central area (i.e., the urban area of Gaocheng), the northwest and northeast area, as well as some sporadic areas in the south of the study area. Generally, the human health risk can be classified into three categories based on the HQ value, i.e., negligible health risk for HQ < 1, medium health risk for HQ in the range of 1–4, high health risk for HQ > 4. Accordingly, most groundwater in the study area had the negligible NO3− health risk. The existing NO3− health risks were mainly concentrated situating in the medium risk category. Although all populations were found threaten by NO3− contaminant at high health risk, the high NO3− risk groundwaters were limited (Figure 9A) and dominantly distributed in the central study area (i.e. the urban area of Gaocheng) (Figures 9A–D). For F− risk, as discussed above, only infants were potentially threatened. While the existing health risks for infants were all belonged to the medium risk category (Figure 8D) and mainly distributed in the northern study area and some sporadic sites of the southern study area (Figure 9E).
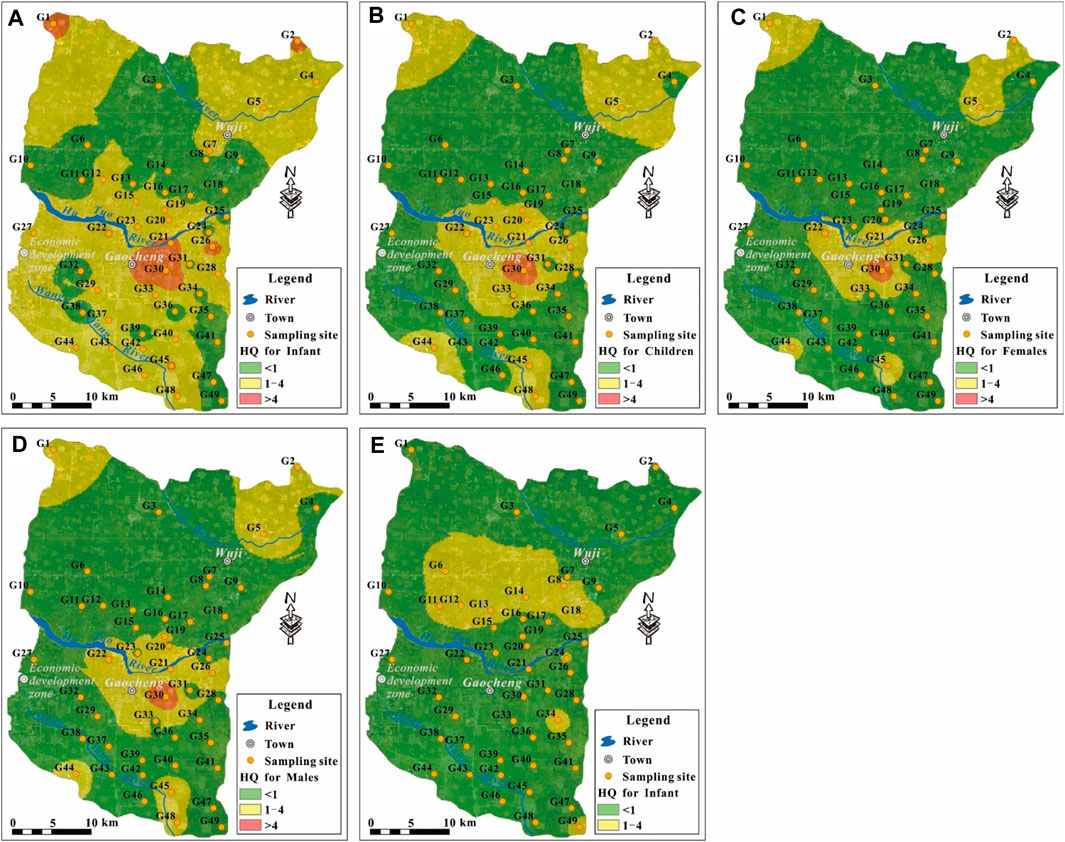
FIGURE 9. Geospatial distribution of the HQ value posed by NO3− for (A) Infants, (B) Children, (C) Adult females, (D) Adult males, and by F− for (E) Infants.
Overall, the aqueous contaminant health risk to residents were predominantly posed from NO3− in groundwater. Special attention should be paid to the groundwater quality safety for domestic usage in the urban Gaocheng. The health threats from aqueous F− contaminant should be concerned for infants in the northern study area and sporadic sites of G34, G49 in the southern study area.
Overall Quality of Groundwater Based on EWQI
Besides the contaminant’s health risk, the quality of phreatic groundwater in the study area was also comprehensively revealed using the Entropy-weighted water quality index (EWQI) approach. A total of 18 physicochemical parameters, i.e., the parameters listed in Table 1 except water temperature (T), were involved in the EWQI assessment. The results suggested that the phreatic groundwater in the study had a wide range of EWQI value from 31 to 419, indicating a large variation of groundwater quality. As demonstrated in Figure 10, most sampled groundwaters (approximately 71.44%) had the EWQI value below 100, suggesting excellent to good water quality (Rank 1 and Rank 2). Additionally, about 12.24% of sampled groundwaters were observed with the EWQI value between 100 and 150, suggesting medium water quality (Rank 3). All these 83.67% of sampled groundwaters were suitable for domestic purposes. Meanwhile, approximately 6.12 and 10.20% of sampled groundwaters were with the EWQI value in the range of 150–200 and beyond 200, respectively, implying poor water quality (Rank 4) and extremely poor water quality. These 16.32% of sampled groundwater were not suitable for directly serving as domestic water.
The geospatial distribution of phreatic groundwater quality rank based on EWQI was visually presented in Figure 11. It can be clearly seen that the study area was dominated with excellent (Rank 1) and good quality (Rank 2) phreatic groundwater. Phreatic groundwater with relatively poor quality (Rank 4 and Rank 5) was concentratedly distributed in the urban area of Gaocheng and its surrounding areas (Figure 11). Besides, groundwater at the sampling site G45 in the south was also found with poor quality (Rank 4). For the medium quality groundwater, it was found mainly in the area surrounding the urban Gaocheng, and also some other sporadic areas across the study area. Comparing the results from human health risk assessment (Figure 9) and EWQI appraisal (Figure 11), it can be clearly known that the poor quality groundwater was predominantly distributed in the urban and surrounding area of Gaocheng. Thus, the development of groundwater resource for domestic usage should be treated with care there, and avoid directly supplying drinking water. However, phreatic groundwater in most areas beyond the urban Gaocheng was great in quality, and suitable and safe for domestic purposes.
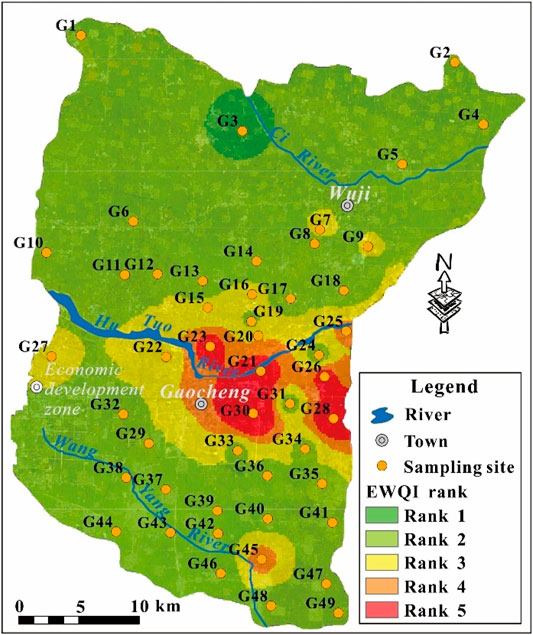
FIGURE 11. Geospatial distribution of the EWQI water quality rank for the phreatic groundwater in the study area.
Conclusion
Phreatic groundwater is the dominant water source supplying human communities worldwide, especially in arid and semiarid regions like the present study area. Hydrochemistry and contamination were investigated and focused on in the present study to get insight into the constrains of poor water quality on the accessibility of phreatic groundwater resources in the Central Shijiazhuang of North China Plain. The main conclusions are as follows:
1) Phreatic groundwater in the study area was slightly alkaline in nature and had relatively large variations of salinity with the TDS varying from 172 to 1875 mg/l. Most groundwaters were moderately-hard fresh water. The hydrogeochemical facies were dominated by HCO3-Ca type, and only a small portion belonged to much saltier types of Mixed Cl-Mg·Ca, Mixed HCO3-Na·Ca and Cl-Ca. Except nitrogen (including NO3−, NO2−, NH4+), F−, Zn, Fe, Mn, and other minor elements were in very low concentration and even below the detection limits. Only NO3− (16.33%), NO2− (28.57%), NH4+ (10.20%), and Fe (14.29%) were found exceeding the desirable limits of drinking water. F− had the concentration approaching the desirable limit of 1 mg/l at some sporadic sites.
2) The hydrochemical composition of phreatic groundwater was naturally governed by water-rock interaction but out of the influence of evaporation. Silicates weathering, sulfates (calcite, aragonite), and carbonates (calcite, aragonite, dolomite) dissolution as well as ion exchange (cation exchange, reverse cation exchange) were the predominant contribution of natural ions. The minor elements of F−, Zn, Fe, Mn were from the dissolution of related minerals in nature. Anthropogenic inputs were responsible for the nitrogen contamination (NO3−, NO2−, NH4+) in the study area, and also resulted in groundwater salinity.
3) Among the detected minor elements, only NO3− and F− would potentially pose non-carcinogenic health risk to residents who rely on phreatic groundwater as drinking water. The susceptibility of population to NO3− contaminant was in the order of Infants > Children > Adult males > Adult females. The health risks from aqueous NO3− in phreatic groundwater were mainly distributed in the urban Gaocheng, and dominated by medium risk for all populations, only a small portion of high risk. Although the F− concentration did not exceed the desirable limit of drinking water, a potential health risk existed in the groundwater in which the F− concentration was approaching the drinking water limit. But this health risk was only to infants rather than other populations, and all in medium risk category.
4) The phreatic groundwater had a large variation of overall water quality from excellent quality to extremely poor quality with the EWQI value of 31–419. While predominant phreatic groundwaters (83.67%) were with the EWQI value below 150 and suitable for domestic purposes. The poor (6.12%) and extremely poor quality (10.20%) groundwater was dominantly distributed in the urban Gaocheng and its surrounding areas, which coincided with the distribution of NO3− risk. Thus, phreatic groundwater in the urban Gaocheng should be careful when exploiting for domestic use. The sporadic threats of F− contaminant to infants should also be concerning. Overall, measures should be taken to protect phreatic groundwater out of the contaminated by sewage effluents in urban and excessive fertilizers in agricultural lands.
Data Availability Statement
The raw data supporting the conclusions of this article will be made available by the authors, without undue reservation.
Author Contributions
Conceptualization: QH, YZ, RW; Methodology: YX, KL, DX; Formal Analysis: YX, QH, DX, XH, XL; Investigation: YX, QH, KL, RW; Data Curation: QH, DX, XH; Software: DX, KL, XH; Writing-Original Draft: YX; Writing-Review and Editing: QH, YZ; Supervision: YZ, RW, XL.
Funding
This research was funded by the Natural Science Foundation of China (grant number 42007183), the S&T Program of Hebei (grant number D2019403193), the Fundamental Research Funds for the Central Universities (grant number 2682021ZTPY063), the Student Research Training Program of Southwest Jiaotong University (grant number 210815), and the Research Project on Teaching Reform of Southwest Jiaotong University (20201023-04).
Conflict of Interest
The authors declare that the research was conducted in the absence of any commercial or financial relationships that could be construed as a potential conflict of interest.
Publisher’s Note
All claims expressed in this article are solely those of the authors and do not necessarily represent those of their affiliated organizations, or those of the publisher, the editors and the reviewers. Any product that may be evaluated in this article, or claim that may be made by its manufacturer, is not guaranteed or endorsed by the publisher.
Acknowledgments
The authors appreciate the editors and reviewers for their critical comments and suggestions which greatly helped us to improve the present manuscript.
References
Adeyeye, O. A., Xiao, C., Zhang, Z., Yawe, A. S., and Liang, X. (2021). Groundwater Fluoride Chemistry and Health Risk Assessment of Multi-Aquifers in Jilin Qianan, Northeastern China. Ecotoxicology Environ. Saf. 211, 111926–112014. doi:10.1016/j.ecoenv.2021.111926
Adimalla, N. (2020). Assessment and Mechanism of Fluoride Enrichment in Groundwater from the Hard Rock Terrain: A Multivariate Statistical Approach. Geochem. Int. 58 (4), 456–471. doi:10.1134/S0016702920040060
Adimalla, N., Qian, H., and Li, P. (2020). Entropy Water Quality index and Probabilistic Health Risk Assessment from Geochemistry of Groundwaters in Hard Rock Terrain of Nanganur County, South India. Geochemistry 80 (4), 125544. doi:10.1016/j.chemer.2019.125544
Ali, W., Aslam, M. W., Junaid, M., Ali, K., Guo, Y., Rasool, A., et al. (2019). Elucidating Various Geochemical Mechanisms Drive Fluoride Contamination in Unconfined Aquifers along the Major Rivers in Sindh and Punjab, Pakistan. Environ. Pollut. 249, 535–549. doi:10.1016/j.envpol.2019.03.043
Almanza Tovar, O. G., Ramos Leal, J. A., Tuxpan Vargas, J., de Jesús Hernández García, G., and De Lara Bashulto, J. (2020). Contrast of Aquifer Vulnerability and Water Quality Indices between a Unconfined Aquifer and a Deep Aquifer in Arid Zones. Bull. Eng. Geol. Environ. 79 (9), 4579–4593. doi:10.1007/s10064-020-01884-x
Alqahtani, F. Z., DaifAllah, S. Y., Alaryan, Y. F., Elkhaleefa, A. M., and Brima, E. I. (2020). Assessment of Major and Trace Elements in Drinking Groundwater in Bisha Area, Saudi Arabia. J. Chem. 2020, 1–10. doi:10.1155/2020/5265634
Amiri, V., Rezaei, M., and Sohrabi, N. (2014). Groundwater Quality Assessment Using Entropy Weighted Water Quality index (EWQI) in Lenjanat, Iran. Environ. Earth Sci. 72 (9), 3479–3490. doi:10.1007/s12665-014-3255-0
Arslan, Ş., Yücel, Ç., Çallı, S. S., and Çelik, M. (2017). Assessment of Heavy Metal Pollution in the Groundwater of the Northern Develi Closed Basin, Kayseri, Turkey. Bull. Environ. Contam. Toxicol. 99 (2), 244–252. doi:10.1007/s00128-017-2119-1
Baalousha, H. M. (2011). Mapping Groundwater Contamination Risk Using GIS and Groundwater Modelling. A Case Study from the Gaza Strip, Palestine. Arab J. Geosci. 4 (3), 483–494. doi:10.1007/s12517-010-0135-0
Chen, J., Tang, C., and Yu, J. (2006). Use of 18O, 2H and 15N to Identify Nitrate Contamination of Groundwater in a Wastewater Irrigated Field Near the City of Shijiazhuang, China. J. Hydrol. 326 (1), 367–378. doi:10.1016/j.jhydrol.2005.11.007
Cheng, Z., Zhang, Y., Su, C., and Chen, Z. (2017). Chemical and Isotopic Response to Intensive Groundwater Abstraction and its Implications on Aquifer Sustainability in Shijiazhuang, China. J. Earth Sci. 28 (3), 523–534. doi:10.1007/s12583-017-0729-5
Duan, L., Wang, W., Sun, Y., Zhang, C., and Sun, Y. (2020). Hydrogeochemical Characteristics and Health Effects of Iodine in Groundwater in Wei River Basin. Expo. Health 12, 369–383. doi:10.1007/s12403-020-00348-7
Dun, Y., Tang, C., and Shen, Y. (2014). Identifying Interactions between River Water and Groundwater in the North China Plain Using Multiple Tracers. Environ. Earth Sci. 72 (1), 99–110. doi:10.1007/s12665-013-2989-4
Gao, X., Li, X., Wang, W., and Li, C. (2020). Human Activity and Hydrogeochemical Processes Relating to Groundwater Quality Degradation in the Yuncheng Basin, Northern China. Ijerph 17 (3), 867. doi:10.3390/ijerph17030867
Gao, Z., Han, C., Yuan, S., Liu, J., Peng, Y., and Li, C. (2021). Assessment of the Hydrochemistry, Water Quality, and Human Health Risk of Groundwater in the Northwest of Nansi Lake Catchment, north China. Environ. Geochem. Health. doi:10.1007/s10653-021-01011-z
GAQS (2017). Standards for Groundwater Quality (GB/T 14848-2017). Beijing: General Administration of Quality Supervision.
Gibbs, R. J. (1970). Mechanisms Controlling World Water Chemistry. Science 170 (3962), 1088–1090. doi:10.1126/science.170.3962.1088
Gu, X., Xiao, Y., Yin, S., Hao, Q., Liu, H., Hao, Z., et al. (2018). Hydrogeochemical Characterization and Quality Assessment of Groundwater in a Long-Term Reclaimed Water Irrigation Area, North China Plain. Water 10 (9), 1209. doi:10.3390/w10091209
Gu, X., Xiao, Y., Yin, S., Pan, X., Niu, Y., Shao, J., et al. (2017). Natural and Anthropogenic Factors Affecting the Shallow Groundwater Quality in a Typical Irrigation Area with Reclaimed Water, North China Plain. Environ. Monit. Assess. 189 (10), 514. doi:10.1007/s10661-017-6229-3
Hao, Q., Lu, C., Zhu, Y., Xiao, Y., and Gu, X. (2018). Numerical Investigation into the Evolution of Groundwater Flow and Solute Transport in the Eastern Qaidam Basin since the Last Glacial Period. Geofluids 2018. doi:10.1155/2018/9260604
Hao, Q., Xiao, Y., Chen, K., Zhu, Y., and Li, J. (2020). Comprehensive Understanding of Groundwater Geochemistry and Suitability for Sustainable Drinking Purposes in Confined Aquifers of the Wuyi Region, Central North China Plain. Water 12 (11), 3052. doi:10.3390/w12113052
He, X., Li, P., Wu, J., Wei, M., Ren, X., and Wang, D. (2021). Poor Groundwater Quality and High Potential Health Risks in the Datong Basin, Northern China: Research from Published Data. Environ. Geochem. Health 43 (2), 791–812. doi:10.1007/s10653-020-00520-7
Helena, B., Pardo, R., Vega, M., Barrado, E., Fernandez, J. M., and Fernandez, L. (2000). Temporal Evolution of Groundwater Composition in an Alluvial Aquifer (Pisuerga River, Spain) by Principal Component Analysis. Water Res. 34 (3), 807–816. doi:10.1016/s0043-1354(99)00225-0
Herrera, C., Godfrey, L., Urrutia, J., Custodio, E., Jordan, T., Jódar, J., et al. (2021). Recharge and Residence Times of Groundwater in Hyper Arid Areas: The Confined Aquifer of Calama, Loa River Basin, Atacama Desert, Chile. Sci. Total Environ. 752, 141847. doi:10.1016/j.scitotenv.2020.141847
Houéménou, H., Tweed, S., Dobigny, G., Mama, D., Alassane, A., Silmer, R., et al. (2020). Degradation of Groundwater Quality in Expanding Cities in West Africa. A Case Study of the Unregulated Shallow Aquifer in Cotonou. J. Hydrol. 582, 124438. doi:10.1016/j.jhydrol.2019.124438
Inglis, J. M., Matisoff, G., and Kelly, W. R. (1986). Pollutant Transport in a Shallow Unconfined Aquifer in Perry, Ohio. Environ. Geol. Water Sci. 8 (4), 237–245. doi:10.1007/BF02524951
Keesari, T., Roy, A., Pant, D., Sinha, U. K., Kumar, P. V. N., and Rao, L. V. (2020). Major Ion, Trace Metal and Environmental Isotope Characterization of Groundwater in Selected Parts of Uddanam Coastal Region, Andhra Pradesh, India. J. Earth Syst. Sci. 129 (1), 205. doi:10.1007/s12040-020-01467-0
Li, C., Gao, X., Li, S., and Bundschuh, J. (2020a). A Review of the Distribution, Sources, Genesis, and Environmental Concerns of Salinity in Groundwater. Environ. Sci. Pollut. Res. 27 (33), 41157–41174. doi:10.1007/s11356-020-10354-6
Li, J., Shi, Z., Liu, M., Wang, G., Liu, F., and Wang, Y. (2021). Identifying Anthropogenic Sources of Groundwater Contamination by Natural Background Levels and Stable Isotope Application in Pinggu basin, China. J. Hydrol. 596, 126092. doi:10.1016/j.jhydrol.2021.126092
Li, J., Shi, Z., Wang, G., and Liu, F. (2020b). Evaluating Spatiotemporal Variations of Groundwater Quality in Northeast Beijing by Self-Organizing Map. Water 12 (5), 1382. doi:10.3390/w12051382
Li, P., He, S., Yang, N., and Xiang, G. (2018a). Groundwater Quality Assessment for Domestic and Agricultural Purposes in Yan'an City, Northwest China: Implications to Sustainable Groundwater Quality Management on the Loess Plateau. Environ. Earth Sci. 77 (23). doi:10.1007/s12665-018-7968-3
Li, Y., Liu, J., Gao, Z., Wang, M., and Yu, L. (2019). Major Ion Chemistry and Water Quality Assessment of Groundwater in the Shigaze Urban Area, Qinghai-Tibetan Plateau, China. Water Supply 20 (1), 335–347. doi:10.2166/ws.2019.167
Li, Y., Zhang, Z., Fei, Y., Chen, H., Qian, Y., and Dun, Y. (2016). Investigation of Quality and Pollution Characteristics of Groundwater in the Hutuo River Alluvial Plain, North China Plain. Environ. Earth Sci. 75 (7), 1–10. doi:10.1007/s12665-016-5366-2
Li, Z., Wang, G., Wang, X., Wan, L., Shi, Z., Wanke, H., et al. (2018b). Groundwater Quality and Associated Hydrogeochemical Processes in Northwest Namibia. J. Geochemical Exploration 186, 202–214. doi:10.1016/j.gexplo.2017.12.015
Liu, H., Guo, H., Yang, L., Wu, L., Li, F., Li, S., et al. (2015). Occurrence and Formation of High Fluoride Groundwater in the Hengshui Area of the North China Plain. Environ. Earth Sci. 74 (3), 2329–2340. doi:10.1007/s12665-015-4225-x
Liu, J., Wang, M., Gao, Z., Chen, Q., Wu, G., and Li, F. (2020). Hydrochemical Characteristics and Water Quality Assessment of Groundwater in the Yishu River basin. Acta Geophys. 68 (3), 877–889. doi:10.1007/s11600-020-00440-1
Luo, Y., Xiao, Y., Hao, Q., Zhang, Y., Zhao, Z., Wang, S., et al. (2021). Groundwater Geochemical Signatures and Implication for Sustainable Development in a Typical Endorheic Watershed on Tibetan Plateau. Environ. Sci. Pollut. Res. 28 (35), 48312–48329. doi:10.1007/s11356-021-14018-x
Marghade, D., Malpe, D. B., Duraisamy, K., Patil, P. D., and Li, P. (2021). Hydrogeochemical Evaluation, Suitability, and Health Risk Assessment of Groundwater in the Watershed of Godavari basin, Maharashtra, Central India. Environ. Sci. Pollut. Res. 28 (15), 18471–18494. doi:10.1007/s11356-020-10032-7
Okkonen, J., and Kløve, B. (2012). Assessment of Temporal and Spatial Variation in Chemical Composition of Groundwater in an Unconfined Esker Aquifer in the Cold Temperate Climate of Northern Finland. Cold Regions Sci. Tech. 71, 118–128. doi:10.1016/j.coldregions.2011.10.003
Qu, S., Shi, Z., Liang, X., Wang, G., and Han, J. (2021a). Multiple Factors Control Groundwater Chemistry and Quality of Multi-Layer Groundwater System in Northwest China coalfield - Using Self-Organizing Maps (SOM). J. Geochemical Exploration 227, 106795. doi:10.1016/j.gexplo.2021.106795
Qu, S., Shi, Z., Liang, X., Wang, G., and Jin, X. (2021b). Origin and Controlling Factors of Groundwater Chemistry and Quality in the Zhiluo Aquifer System of Northern Ordos Basin, China. Environ. Earth Sci. 80 (12), 439. doi:10.1007/s12665-021-09735-y
Rahimi-Feyzabad, F., Yazdanpanah, M., Gholamrezai, S., and Ahmadvand, M. (2021). Institutional Constraints to Groundwater Resource Management in Arid and Semi-arid Regions: a Straussian Grounded Theory Study. Hydrogeol J. 29 (3), 925–947. doi:10.1007/s10040-020-02283-y
Rajmohan, N., and Prathapar, S. A. (2016). Assessment of Geochemical Processes in the Unconfined and Confined Aquifers in the Eastern Ganges Basin: a Geochemical Approach. Environ. Earth Sci. 75 (17), 1212. doi:10.1007/s12665-016-6017-3
Rao, G. T., Rao, V. V. S. G., and Ranganathan, K. (2013). Hydrogeochemistry and Groundwater Quality Assessment of Ranipet Industrial Area, Tamil Nadu, India. J. Earth Syst. Sci. 122 (3), 855–867. doi:10.1007/s12040-013-0295-x
Reynolds, S., and Johnson, J. (2019). Exploring Earth Science. Second Edition. New York: McGraw-Hill Education.
Satheeskumar, V., Subramani, T., Lakshumanan, C., Roy, P. D., and Karunanidhi, D. (2020). Groundwater Chemistry and Demarcation of Seawater Intrusion Zones in the Thamirabarani delta of South India Based on Geochemical Signatures. Environ. Geochem. Health 43, 757–770. doi:10.1007/s10653-020-00536-z
Solgi, E., and Jalili, M. (2021). Zoning and Human Health Risk Assessment of Arsenic and Nitrate Contamination in Groundwater of Agricultural Areas of the Twenty Two Village with Geostatistics (Case Study: Chahardoli Plain of Qorveh, Kurdistan Province, Iran). Agric. Water Manag. 255, 107023. doi:10.1016/j.agwat.2021.107023
Souid, F., Agoubi, B., and Kharroubi, A. (2017). “Assessing the Groundwater Pollution Problem by Nitrate and Faecal Bacteria: Case of Djerba Unconfined Aquifer (Southeast Tunisia),” in Water and Land Security in Drylands: Response to Climate Change. Editors M. Ouessar, D. Gabriels, A. Tsunekawa, and S. Evett (Cham: Springer International Publishing), 87–96. doi:10.1007/978-3-319-54021-4_9
Su, H., Kang, W., Kang, N., Liu, J., and Li, Z. (2021). Hydrogeochemistry and Health Hazards of Fluoride-Enriched Groundwater in the Tarim Basin, China. Environ. Res. 200, 111476. doi:10.1016/j.envres.2021.111476
Taylor, M. P. (1996). The Variability of Heavy Metals in Floodplain Sediments: A Case Study from Mid Wales. CATENA 28 (1), 71–87. doi:10.1016/S0341-8162(96)00026-4
USEPA (1989). Risk Assessment Guidance for Superfund, Volume I: Human Health Evaluation Manual (Part A), Interim Final. Washington, DC.
USEPA (2008). User’s Guide: Human Health Risk Assessment. Washington, DC, USA: United States Environmental Protection Agency.
Vaiphei, S. P., Kurakalva, R. M., and Sahadevan, D. K. (2020). Water Quality index and GIS-Based Technique for Assessment of Groundwater Quality in Wanaparthy Watershed, Telangana, India. Environ. Sci. Pollut. Res. 27 (36), 45041–45062. doi:10.1007/s11356-020-10345-7
Venkatramanan, S., Chung, S. Y., Selvam, S., Lee, S. Y., and Elzain, H. E. (2017). Factors Controlling Groundwater Quality in the Yeonjegu District of Busan City, Korea, Using the Hydrogeochemical Processes and Fuzzy GIS. Environ. Sci. Pollut. Res. 24 (30), 23679–23693. doi:10.1007/s11356-017-9990-5
Voutchkova, D. D., Ernstsen, V., Kristiansen, S. M., and Hansen, B. (2017). Iodine in Major Danish Aquifers. Environ. Earth Sci. 76 (13), 447. doi:10.1007/s12665-017-6775-6
Wang, Q., Dong, S., Wang, H., Yang, J., Huang, H., Dong, X., et al. (2020a). Hydrogeochemical Processes and Groundwater Quality Assessment for Different Aquifers in the Caojiatan Coal Mine of Ordos Basin, Northwestern China. Environ. Earth Sci. 79 (9), 199. doi:10.1007/s12665-020-08942-3
Wang, W., Zhang, Z., Yin, L., Duan, L., and Huang, J. (2021). Topical Collection: Groundwater Recharge and Discharge in Arid and Semi-arid Areas of China. Hydrogeol J. 29 (2), 521–524. doi:10.1007/s10040-021-02308-0
Wang, Y., Li, J., Ma, T., Xie, X., Deng, Y., and Gan, Y. (2020b). Genesis of Geogenic Contaminated Groundwater: as, F and I. Crit. Rev. Environ. Sci. Tech., 1–39. doi:10.1080/10643389.2020.1807452
WHO (2017). Guidelines for Drinking-Water Quality. 4th edition. Geneva: Incorporating the First AddendumWorld Health Organization.
Xiao, Y., Hao, Q., Zhang, Y., Zhu, Y., Yin, S., Qin, L., et al. (2022). Investigating Sources, Driving Forces and Potential Health Risks of Nitrate and Fluoride in Groundwater of a Typical Alluvial Fan plain. Sci. Total Environ. 802, 149909. doi:10.1016/j.scitotenv.2021.149909
Xiao, Y., Liu, K., Hao, Q., Li, J., Zhang, Y., Cui, W., et al. (2021). Hydrogeochemical Features and Genesis of Confined Groundwater and Health Perspectives for Sustainable Development in Urban Hengshui, North China Plain. J. Chem. 2021, 1–15. doi:10.1155/2021/5578192
Xiao, Y., Shao, J., Frape, S. K., Cui, Y., Dang, X., Wang, S., et al. (2018). Groundwater Origin, Flow Regime and Geochemical Evolution in Arid Endorheic Watersheds: a Case Study from the Qaidam Basin, Northwestern China. Hydrol. Earth Syst. Sci. 22 (8), 4381–4400. doi:10.5194/hess-22-4381-2018
Xiao, Y., Yin, S., Hao, Q., Gu, X., Pei, Q., and Zhang, Y. (2020). Hydrogeochemical Appraisal of Groundwater Quality and Health Risk in a Near-Suburb Area of North China. J. Water Supply: Res. Technology-Aqua 69 (1), 55–69. doi:10.2166/aqua.2019.101
Xue, X., Li, J., Xie, X., Qian, K., and Wang, Y. (2019). Impacts of Sediment Compaction on Iodine Enrichment in Deep Aquifers of the North China Plain. Water Res. 159, 480–489. doi:10.1016/j.watres.2019.05.036
Yin, S., Xiao, Y., Gu, X., Hao, Q., Liu, H., Hao, Z., et al. (2019). Geostatistical Analysis of Hydrochemical Variations and Nitrate Pollution Causes of Groundwater in an Alluvial Fan plain. Acta Geophys. 67 (4), 1191–1203. doi:10.1007/s11600-019-00302-5
Yin, S., Xiao, Y., Han, P., Hao, Q., Gu, X., Men, B., et al. (2020). Investigation of Groundwater Contamination and Health Implications in a Typical Semiarid Basin of North China. Water 12 (4), 1137. doi:10.3390/w12041137
Zeng, Y., Zhou, Y., Zhou, J., Jia, R., and Wu, J. (2018). Distribution and Enrichment Factors of High-Arsenic Groundwater in Inland Arid Area of P. R. China: A Case Study of the Shihezi Area, Xinjiang. Expo. Health 10 (1), 1–13. doi:10.1007/s12403-016-0241-7
Zhang, Y., Wu, Y., Sun, J., Hu, S., Zhang, Y., and Xiang, X. (2018). Controls on the Spatial Distribution of Iodine in Groundwater in the Hebei Plain, China. Environ. Sci. Pollut. Res. Int. 25 (3), 16702–16709. doi:10.1007/s11356-018-1843-3
Zhao, X., Guo, H., Wang, Y., Wang, G., Wang, H., Zang, X., et al. (2021). Groundwater Hydrogeochemical Characteristics and Quality Suitability Assessment for Irrigation and Drinking Purposes in an Agricultural Region of the North China plain. Environ. Earth Sci. 80 (4), 162. doi:10.1007/s12665-021-09432-w
Zhi, C., Chen, H., Li, P., Ma, C., Zhang, J., Zhang, C., et al. (2019). Spatial Distribution of Arsenic along Groundwater Flow Path in Chaobai River Alluvial-Proluvial Fan, North China Plain. Environ. Earth Sci. 78 (8), 259. doi:10.1007/s12665-019-8260-x
Keywords: phreatic groundwater, hydrochemistry, water quality, health risk assessment, nitrate, heavy metal, fluoride
Citation: Xiao Y, Xiao D, Hao Q, Liu K, Wang R, Huang X, Liao X and Zhang Y (2021) Accessible Phreatic Groundwater Resources in the Central Shijiazhuang of North China Plain: Perspective From the Hydrogeochemical Constraints. Front. Environ. Sci. 9:747097. doi: 10.3389/fenvs.2021.747097
Received: 25 July 2021; Accepted: 05 October 2021;
Published: 27 October 2021.
Edited by:
Brindha Karthikeyan, Freie Universität Berlin, GermanyCopyright © 2021 Xiao, Xiao, Hao, Liu, Wang, Huang, Liao and Zhang. This is an open-access article distributed under the terms of the Creative Commons Attribution License (CC BY). The use, distribution or reproduction in other forums is permitted, provided the original author(s) and the copyright owner(s) are credited and that the original publication in this journal is cited, in accordance with accepted academic practice. No use, distribution or reproduction is permitted which does not comply with these terms.
*Correspondence: Qichen Hao, aGFvcWljaGVuX2loZWdAMTYzLmNvbQ==