Continuous Cover Forestry and Cost of Carbon Abatement on Mineral Soils and Peatlands
- 1Natural Resources Institute Finland, Turku, Finland
- 2Natural Resources Institute Finland, Helsinki, Finland
- 3Natural Resources Institute Finland, Oulu, Finland
- 4Institute of Physicochemical and Biological Problems in Soil Science of the Russian Academy of Sciences, Moscow, Russia
- 5Center for Forest Ecology and Productivity, Russian Academy of Sciences, Moscow, Russia
Continuous cover forestry (CCF) has proven to financially outperform rotation forestry (RF) with low or even moderate social price of carbon in mineral soils. However, to date there are no studies to compare financial performance of joint production (timber and carbon sequestration) between mineral soils and peatlands when CCF is applied. A vast variety of harvest intervals and intensity (expressed as post-harvest basal area) for a mature spruce-dominated [Picea abies (L.) Karst.] stand on both mineral and peat soils was simulated with process-based ecosystem model, EFIMOD. In addition, four levels of carbon price (0, 25, 50 and 75€/tCO2) were applied in assessing the profitability of joint production (timber and carbon sequestration) associated with CCF. Mineral soil turned out to be superior to peatland in cost-efficiency of carbon sequestration. For instance, the cost of additional ton of CO2 was only €2/tCO2 with a carbon price of €25/tCO2 for a private forest owner (through carbon trading), while on peatland it fluctuated between €30 and €39.5/tCO2, depending on the carbon price applied for a private forest owner (€25-€75/tCO2). In general, mineral soil was more sensitive to harvest interval and intensity than peatland, with respect to cost-efficiency in climate change mitigation.
Introduction
Climate change is basically driven by an imbalance in the global energy budget (Lintunen et al., 2021). The imbalance is mainly due to carbon dioxide (CO2) emissions increased by human activity, i.e., anthropogenic emissions (e.g., Stern et al., 2006; IPCC, 2021). In brief, anthropogenic emissions cause radiative forcing which further creates global warming corresponding to the gradual increase in global surface temperature (IPCC, 2014). It is a well-demonstrated fact that these anthropogenic emissions of greenhouse gases (GHG) have damaging impacts on earth’s atmosphere (e.g., IPCC 2014; Gren and Aklilu, 2016). Thus, recognition of the need to stabilize (or even decrease) the C content in the atmosphere has been manifested in various international and national agreements and policies—such as the Kyoto Protocol (e.g., Lutz and Meyer, 2009), the Paris Climate Agreement (e.g., Juutinen et al., 2018a), and the EU climate action and the European Green Deal (e.g., Bieroza et al., 2021).
Reaching climate mitigation objectives requires immediate action (Masson-Delmotte et al., 2018), and the forest sector (incl. forests) is seen as an important contributor (Eriksson, 2015; Riahi et al., 2017; Jhariya et al., 2019; Raj and Jhariya 2021; Riviere and Caurla, 2021). Forest ecosystems account for app. 80% of aboveground terrestrial C and 70% of soil organic C (Dixon et al., 1994; Hui et al., 2017), presenting a positive net C balance (Lal, 2005), i.e., a net C sink (Pan et al., 2011). Boreal forests play a key role in C uptake because they are estimated to contain the highest C stocks per area unit after wetlands (IPCC, 2001), and the soil C stock in boreal forests represents a distinctively dominant proportion of total C stored (Mayer et al., 2020). In practice, the accumulated C can be stored in the forest to increase the C stock and/or harvested for consumption and further to substitute for fossil origin products reducing fossil C in the atmosphere (Lundmark et al., 2018). As a rule of thumb, less intensive thinnings maintaining higher growing stock volumes and applying longer rotation periods both increase C stocks in forests (Liski et al., 2001; Pukkala et al., 2011; Zubizarreta-Gerendiain et al., 2016). Recently, continuous cover forestry (CCF) has been demonstrated to enhance C storage in boreal forests (Assmuth and Tahvonen, 2018; Kellomäki et al., 2019; Shanin et al., 2021). Contrary to rotation forestry (RF), CCF applies partial selection cuttings (i.e., thinnings) without clearcutting and relies on continuous natural regeneration (Kuuluvainen et al., 2012).
Regarding possible differences between forests on mineral soils and peatland forests, C stock in the peat layer of boreal forested peatlands can be up to ten times larger compared to mineral soil forests (Weishampel et al., 2009). The difference is due to the naturally high ground water level (WL) of peatlands which slows down the decomposition of organic matter and favours accumulation of partly decomposed material as peat (Ojanen et al., 2010). Drainage for forestry lowers the WL exposing deeper peat layers to decomposition, which, in turn, create CO2 emissions from soil with a magnitude that might turn the drained sites into net sources of CO2 to the atmosphere—unlike the pristine (unmanaged) peatlands and mineral soils (Nieminen et al., 2018; Shanin et al., 2021). In drained peatlands the depth of the WL is affected by microclimate and the forest stand’s evapotranspiration capacity (Leppä et al., 2020a) and also by forest management (e.g., ditch network maintenance, DNM (Lauren et al., 2021). In brief, in drained peatlands WL affects the whole ecosystem C balance in the long term, and all ditching operations likely result in enhanced peat decomposition in deeper layers with corresponding increase of GHG fluxes (Ojanen and Minkkinen, 2019). Significant net C emissions may release from the most productive nutrient-rich site types (Ojanen et al., 2013). The WL can also be maintained at a desired level by utilizing the evapotranspiration of trees through controlling the stand density (Leppä et al., 2020b). For both site categories (mineral soils and peatlands) CCF may enable more efficient C sequestration due to the absence of treeless stage—compared to traditional RF with clearcutting and DNM (Shanin et al., 2021). Further, avoiding DNM by applying CCF on peatlands decrease or may even dispose all the detrimental impacts resulting from RF on several ecosystem services by peatlands (Nieminen et al., 2018).
The social cost of C (SCC) is a measure to monetize the damage from releasing a ton of CO2, and pricing C according to SCC provides the correct economic incentive for reducing current emissions (van den Bijgaard et al., 2016). Further, a price on C might help shift the burden for the damage from GHG emissions back to those who are responsible for it and who can avoid it (The World Bank, 2020). Despite criticism in general on C pricing1, scholars and academia share a common view that some measure (price) is needed in order to provide an incentive for households, firms and governments to reduce emission cost-effectively. Principally and in the long run, the prospect of continuing and possibly rising C prices also provide a motivation for innovations to lower the cost of reducing emissions (Boyce, 2018).
In environmental policy, C services can be administered in different ways (Pohjola and Valsta, 2007; Verkerk et al., 2020). For instance, governments may use tax and subsidy-based instruments in forest ecosystems to incentivise meeting emission targets (Pohjola and Valsta, 2007; Evison, 2017; Juutinen et al., 2018b). In short, the amount of C sequestration within a forest stand depends on the level of growing stock, which in turn is controlled by thinnings and harvest(s) (Pohjola and Valsta, 2007; Pyörälä et al., 2014). This applies for CCF as well as for RF (Assmuth and Tahvonen, 2018). Then the rate of soil C sequestration is driven by litter input of growing stock, natural mortality and amount of harvest residues (Jhariya 2017a; Jhariya 2017b). Introducing C sequestration objectives affects optimal forest management in various ways by imposing changes to business-as-usual management and/or optimal management regimes (van Kooten et al., 1995; Olschewski and Benitez, 2010; Matthies and Valsta, 2016; Zengin and Unal, 2019). Basically, it is a question whether these changes are financially viable for a landowner to apply, and at the same time whether they generate a net amount of sequestered C in excess of status quo, a predetermined baseline C stock - thus creating an additionality (Asante and Armstrong, 2016).
Recent studies on CCF have focused on comparing the financial performance of CCF and RF on mineral soils with either one dominant tree species (e.g., Tahvonen, 2016; Tahvonen and Rämö, 2016; Parkatti et al., 2019) or mixed-species (Tahvonen et al., 2019; Parkatti and Tahvonen, 2020), but there is a lack of papers tackling with CCF on peatlands (Juutinen et al., 2021). Further, to our knowledge there are no articles assessing joint production of timber and carbon sequestration on peatlands except Shanin et al. (2021) that analyses the effect of CCF on the CO2 and methane (CH4) emissions from nutrient-rich drained peatland sites in southern Finland. This study is the first attempt to compare financial performance of joint production (timber and carbon sequestration) between mineral soils and peatlands when CCF is applied as a management regime. Then, special emphasis is laid on alternative C prices to discover trade-offs between timber production and C sequestration, and to find out whether it would be cheaper (more cost-efficient) to mitigate emissions in forests on mineral soils or on peatlands. Our hypotheses are: 1) Mineral soil and peatland differ from each other with respect to how cost-efficient the C sequestration through CCF would be, 2) peatland soil is a C source and therefore C pricing on peatland has negative effect on private forest owners’ incomes, and 3) C pricing leads to less intensive harvests in CCF compared to absence of C pricing.
Materials and Methods
Input Data
Analyses were based on a virtual forest plot obtained by aggregating the empirical plot-wise tree stand data from two experimental sites in the southern boreal zone of Finland (for further details, see Juutinen et al., 2021; Shanin et al., 2021). These sites were dominated by mature Norway spruce forests with admixture of downy birch (Betula pubescens Ehrh.). The initial stand characteristics were identical for mineral soil and peatland case in terms of size-class distribution and number of trees. The generated simulation plot represented a mixed uneven-aged stand with 1,212 trees ha−1 and basal area (BA) of 24.6 m2 ha−1. The diameter at breast height (DBH) of trees varied between ca. 0.3–38.5 cm (mean 13.5 cm), and the tree height fluctuated between 1.3 and 27.2 m (mean 12.9 m). The resulting distribution of trees among DBH classes was bimodal, peaked at middle (15–20 cm) and smaller (<5 cm) size classes. Then, 89% was spruce and 11% was birch, and the size-class distribution was similar for both tree species (Juutinen et al., 2021; Shanin et al., 2021). The initial soil C for the mineral soil site was set to 8.16 kg m−2 (1.34 kg m−2 in forest floor, and 6.82 kg m−2 in mineral horizons), and the initial C stock in the peat layer of peatland site was set to 147.36 kg m−2 (the thickness of peat layer is 2 m).
EFIMOD Model and Stand Projections
For producing stand projections in the generated simulation plot the EFIMOD model (Komarov et al., 2003) was applied. The EFIMOD is a spatially explicit, individual-tree based model that simulates the biological turnover in tree-soil systems. EFIMOD operates with an annual time step. A simulated stand consists of individual trees which interact with neighbouring trees. Each tree forms a shadow zone and nutrition zone, the sizes of which depend on the tree size. The shape of the rooting zone and crown of an individual tree is defined by the availability of resource (nitrogen in the soil and light) and interaction of an individual tree with neighbouring trees (Shanin et al., 2015; Shanin et al., 2020). The biomass increment of each tree is calculated on the basis of consumed soil nitrogen and intercepted solar radiation. This biomass increment is allocated between above- (stem, branches and foliage) and below- (coarse and fine roots) ground compartments, and the corresponding litter inputs are calculated based on the annual mortality rate of each compartment. Total litter production at the stand level also included the litter input from dead trees, as well as felling residues. Then, the three main components of C balance (C sequestration to living biomass and to dead organic matter), litter production and C losses due to decomposition of litter and peat layer) were included in the EFIMOD simulations (for further details, see Shanin et al., 2021). Briefly, on mineral soil the rates of decomposition of soil organic matter (both humification and mineralization) were simulated with process-based model Romul_Hum (Komarov et al., 2017), according to which they are dependent on temperature and moisture of the forest floor and the mineral soil, as well as on the nitrogen and ash content in the litter. The main outputs of the Romul_Hum model are C stocks in forest floor, labile and stable humus of mineral soil horizons, and CO2 emission from decomposition. The model does not explicitly consider the soil horizons, but the simulated pools can be considered as organic layer and topsoil. However, this model has some limitations for application on peatlands, and therefore for this site only the decomposition of fresh litter was simulated with the Romul_Hum model, while the net CO2 and CH4 emissions from decomposing peat layer was calculated with the empirical equations, which quantify the relationship between emissions and soil water table (Shanin et al., 2021 for more details). However, the export of dissolved organic C was excluded from the analysis, because it may be transferred from organic layer but will accumulate on topmost mineral soil layer just below organic layer (Lindroos et al., 2008), and therefore this process has minor importance. With regard to a specific feature on peatland forestry, namely the ground WL the stand dynamics were linked to the WL level by specific models (Juutinen et al., 2021). Further, the influence of the WL on the net primary production and stand regeneration was also modelled with the EFIMOD system (Shanin et al., 2021).
The simulation scenarios of CCF (produced by EFIMOD simulations) were identical to those applied in Juutinen et al. (2021) and Shanin et al. (2021) and consisted of a series of selection cuttings initiated at the first step of simulation, where trees were removed, following the principles of CCF, mainly from the dominant and intermediate tree layers. The period between two consecutive selection cuttings was defined by the harvest interval R, which was set at 10, 15, 20, and 30 years, and the harvest intensity was defined by the post-harvest stand BA with values of 4, 6, 8, 10, 12, 14, and 16 m2 ha−1. Each cutting action assumed harvest of the larger trees but with retention of 5% of largest ones as seed trees. Harvesting was simulated as roundwood outturn of trees selected for cutting, while the harvesting residues, i.e., branches and foliage, stumps and belowground parts were assumed to be left on site. The length of the simulation period was set at 240 years. For peatland case management each scenario was simulated as two variants: with and without ditch network maintenance (DNM) since the gradual deterioration of the ditches overgrown by vegetation in time can result in rising WL levels. Further, in the variant with DNM, the DNM was carried out every 60 years in the cases of post-harvest BA of 4 and 6 m2 ha−1 and every 120 years in the case of post-harvest BA of 8 m2 ha−1 to maintain the WL below 0.35 m, at which levels it is assumed not to have significant effects on the growth and regeneration of trees (Juutinen et al., 2021). At the BAs higher than 8 m2 ha−1, due to high evapotranspiration capacity of growing trees, WL remained below 0.35 m without DNM (Sarkkola et al., 2009; Juutinen et al., 2021).
Financial Performance
Recall we simulated 28 different CCF scenarios with varying harvest intervals and post-harvest BAs. The profitability of each scenario was determined by calculating the net present value (NPV) of harvest revenues. Denote the harvest interval (years) in scenario s by
The roadside prices, unit costs, and the time consumption models used to calculate the variable unit harvest costs were the same as in Juutinen et al. (2021). Variable unit harvesting costs were €95 h−1 and €80 h−1 for cutting and haulage, respectively, and time consumption both for CCF and RT were based on Väätäinen et al. (2010). For planting costs, the cost of site preparation was €382.8 ha−1, €633.2 ha−1 for planting (including labor and material costs), €374.8 ha−1 for tending, and €429.6 ha−1for pre-commercial thinning. Costs for DNM was €201.6 ha−1 based on Juutinen et al. (2021) where ditches were assumed to be parallel, with 50 m between their mid-lines, and using deflated (according to cost-of-living index) average unit prices of years 2013–2017. Finally, roadside prices for sawlog (pulp) for spruce were €57.73 m−3 (€30.40 m−3) and for birch €47.39 m−3 (€29.58 m−3). Climate subsidies are based on EU ETS prices. 25 €/t CO2eq. is close to long-term average, 50 €/t CO2eq is close to pricing in spring 2021, and 75 €/t CO2eq. a potential future pricing.
Results
Mineral Soils
On mineral soils, in baseline with 3% interest rate and no C subsidies, the best scenario with the highest NPV is the most intensive option with 10-year interval and 4 m2 ha−1 post-harvest BA (Figure 1). When C subsidy is increased from zero to 25 €/t CO2eq., the best scenario shifts to one with harvesting interval of 20 years and post-harvest BA of 16 m2 ha−1. Increasing C price further to 50 or 75 €/t CO2eq. increases the harvesting interval further to 30 years.
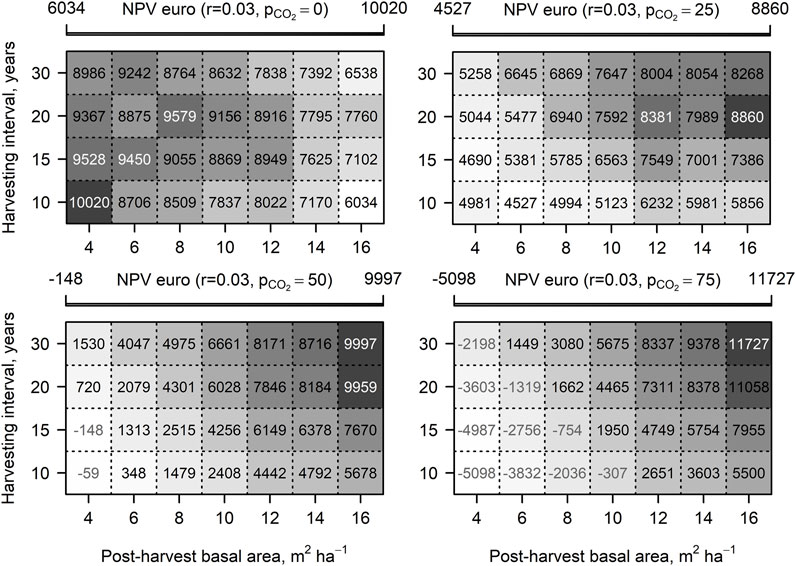
FIGURE 1. Net present value (€/ha) of each scenario on mineral soil with 3% interest rate (r) and C price (pCO2) of 0, 25, 50, and 75 €/t CO2eq.
When the C price is increased, the effect on NPV of each scenario depends on the intensity of the harvests; the more intense the harvests, the more negative the subsidies’ effect on the NPV. If the harvests are light enough, the effect of the subsidy system on the NPV is positive. To see how dependent the results are on the interest rate, we set the C subsidy to 50 €/t CO2eq. and vary the interest rate between 1 and 4%. As expected, due to discounting, the higher is the interest rate, the lower is the NPV of all scenarios (Figure 2). Increasing interest rate also decreases the differences between scenarios since harvests occurring far later in time are less relevant the higher the interest rate, and when increasing interest rate from 3 to 4% the best scenarios shift to shorter intervals. For instance, from 30-year interval with to 20-year interval, both with 16 m2 ha−1 post-harvest BA from 1 to 3% does not change the best scenario: 30-year interval with 16 m2 ha−1 post-harvest BA produces the highest NPV.
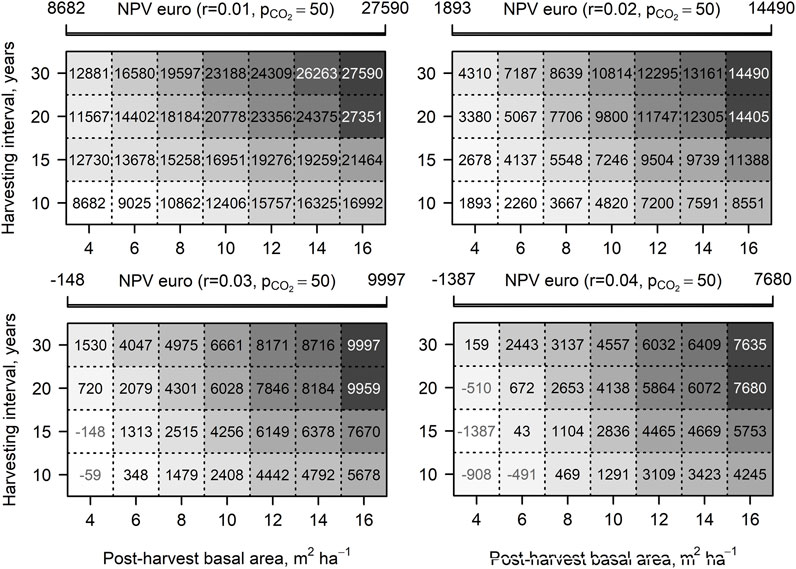
FIGURE 2. Net present value (€/ha) of each scenario on mineral soil with 50 €/CO2eq. C subsidy (pCO2) and interest rates (r) of 1, 2, 3, and 4%.
On mineral soil, average stand carbon storage varies between 21 t C and 78 t C ha−1 (Table 1). Differences in average total soil C (sum of organic layer and topsoil) are roughly the same, varying between 51 t C and 101 t C ha−1. Total discounted carbon fluxes on mineral soil vary from −55 t C ha−1 to 19 t C ha−1 (Figure 3).

TABLE 1. Average stand and soil carbon storage (t C) of each scenario on mineral soil. Stand carbon storage is the first number and soil the latter. Harvest interval, HI (in years) in rows, post-harvest basal area, BA (m2 ha−1) in columns. The highest combined carbon storage presented in bold.
Peatland
On peatland, with 3% interest rate and no C pricing the best scenario is with 15-year harvesting interval and 10 m2 ha−1 post-harvest BA (Figure 4). The effect of increasing C price is similar to mineral soil; as C price is increased, the scenario with highest NPV has less intensive harvests. Increasing C price to 25 €/t CO2eq, the scenario with highest NPV has interval of 15-year, but the post-harvest BA is increased to 16 m2 ha−1. Increasing C price further increases the harvesting interval, with the least intensive scenario being the best when C price is 50 €/t CO2eq or higher.
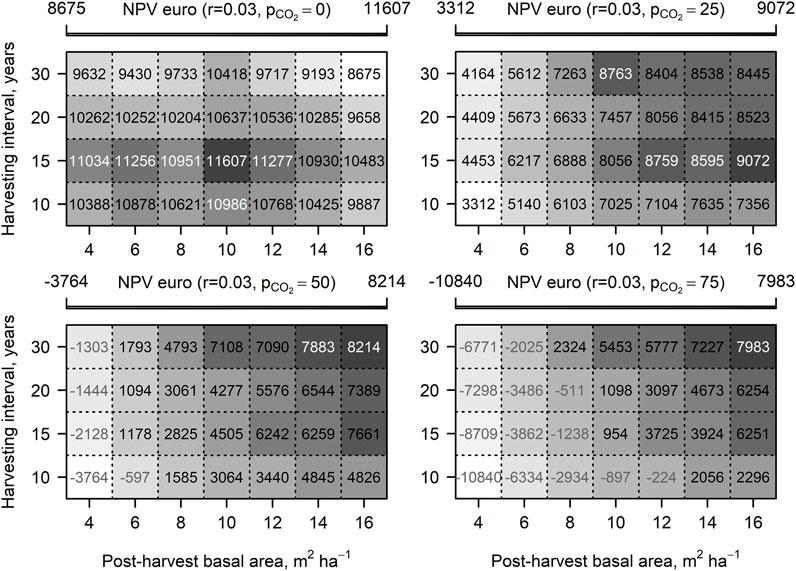
FIGURE 4. Net present value (€/ha) of each scenario on peatland with 3% interest rate (r) and CO2 price (pCO2) of 0, 25, 50, and 75 €/t CO2eq.
While the C pricing has, similarly to mineral soils, the stronger effect the more intensive the harvests are, the effect on NPV on peatlands is solely negative. Difference in the results between the soil types is due to the soil GHG emissions on peatlands, especially early in the scenarios when ground water level is low.
Increasing interest rate has similar effect as C pricing; the higher the interest rate, the better is the relative profitability of the scenarios of less intensive harvests (Figure 5). With 1% interest rate, the best scenario uses 15-year rotation with 16 m2 ha−1 post-harvest BA. Increasing the interest rate to 2%, shifts the best scenario to 30-year rotation. Increasing interest rate further strengthens the superiority of the 30-year rotation with 16 m2 ha−1 post-harvest BA.
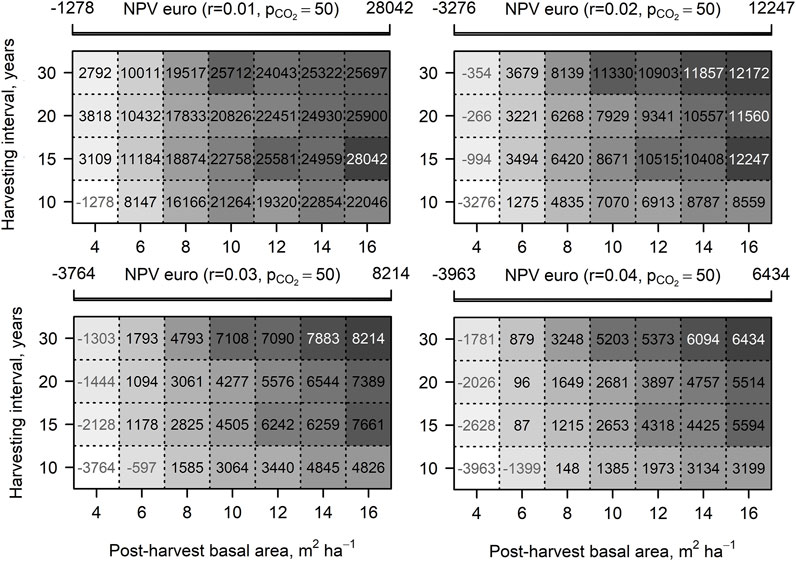
FIGURE 5. Net present value (€/ha) of each scenario on peatland with 50 €/t CO2eq. C subsidy (pCO2) and interest rates (r) of 1, 2, 3, and 4%.
On peatland, due to the peat C content, the soil C storage vastly exceeds that of stand C (Table 2). Average total C storage varies between 1,426 t C and 1,543 t C ha−1, of which soil C accounts for over 95%. While the soil C storage is massive, the differences in the storage between the scenarios are roughly the same as in mineral soils. Total discounted C fluxes on peatland soil vary from −87.5 t C ha−1 to −2.6 t C ha−1 (Figure 6). Due to the heavy first harvest and soil emissions especially early in the scenario when ground water level is low, total discounted changes in C storage is negative for all scenarios.

TABLE 2. Average stand and soil carbon storage (t C) of each scenario on peatland. Stand carbon storage is the first number and soil the latter. Harvest interval, HI (in years) in rows, post-harvest basal area, BA (m2 ha−1) in columns. The highest combined carbon storage presented in bold.
Discussion
Recent studies (Assmuth and Tahvonen, 2018; Parkatti and Tahvonen, 2021) suggest that CCF outperforms rotation forestry (RF) with low or even moderate social price of C in mineral soils. Further, in RF carbon payments tend to increase optimal rotation period (van Kooten et al., 1995; Pohjola and Valsta, 2007; Juutinen et al., 2018a) while in CCF the transition phase is prolonged (Assmuth and Tahvonen, 2018). In addition, C pricing affects thinnings both in RF and CCF. To date, however, there have not been any studies at stand level comparing the financial performance associated with CCF to mitigate climate change between mineral soils and peatlands. Our simulation study is the first attempt to focus on cost-efficiency of CCF in mitigating climate change both on mineral soils and peatlands. We discovered whether managing forests according to identical CCF either in mineral soils or on peatlands would make any difference with respect to financial incentives for private forest owners to mitigate climate change.
The economic cost of additional C storage (i.e., cost of C abatement) is usually measured as lost timber income (Assmuth and Tahvonen, 2018)2. In this study, mineral soil was superior to peatland in cost-efficiency of C abatement. For instance, in peatland the cost of additional ton of CO2 fluctuated between €30 and €39.5/tCO2, depending on the C price applied for a private forest owner (€25-€75/tCO2) while in mineral soil the cost of additional ton of CO2 was only €2/tCO2 with a C price of €25/tCO2, and even turned into a benefit (negative cost of C abatement) when C price was €50 or €75/tCO2. This indicates a win-win situation in which a private forest owner and society both gain when C pricing is being introduced. The former can improve the profitability at stand level and the latter can contribute climate change mitigation by increased C sequestration of trees with costs less than the initial C price paid/subsidized for private forest owners.
Partially the poorer economic performance on peatlands can be explained by initial state. At the beginning of each scenario, the stand is dense and ground water level very low (Juutinen et al., 2021). Because of this, there are large GHG emissions from the soil as the peat decomposes (Shanin et al., 2021). As a result, the C pricing has negative effect of NPV on all peatland scenarios. However, the initial state also harms the C subsidies’ effect on NPV on mineral soils, since the first harvest has significant impact also on changes in C storage and discounted C on both site types.
Further, because of this, on peatland the sole timber production (without carbon pricing) was the best choice for a private forest owner while in mineral soil the higher C pricing improved the financial outcome for a private forest owner, with interest rate of 3%. However, while from private forest owner’s perspective C pricing has negative effect on economy on peatlands, for society it is still beneficial, as C pricing changes the best scenario to less intensive management, leading to significant avoided emissions (Figure 8).
An earlier study (Assmuth and Tahvonen, 2018) in mineral soil demonstrated that with increasing C price CCF becomes more profitable than RF, and this holds for interest rates between app. 1.5–6%, regeneration costs ranging from ca. 1,000 to 2,500 € ha−1. Another discrepancy between the results of mineral soil and peatland was that with C price at €50/tCO2, in mineral soil the best or second-best performer was always—regardless of the interest rate—a CCF with 30-year harvesting interval and intensity of 16 m2 ha−1 (post-harvest BA) while in peatland the best performer with low interest rates (1 and 2%) was a CCF with 15-year harvesting interval and intensity of 16 m2 ha−1. These results with relatively mild or moderate harvest intensity (post-harvest BA > 14 m2 ha−1) are contradicting to earlier studies of financial performance related to CCF on mineral soil (Juutinen et al., 2018b) and on peatland (Juutinen et al., 2021). The difference between this study and earlier studies, however, is due to the inclusion of C pricing which is shown to increase on average the growing stock in optimal CCF management (Parkatti and Tahvonen, 2021).
C pricing has been identified as the most effective strategy to reduce GHG emissions (World Bank Group, 2019), or pricing emissions is at least superior to financial subsidies as long as the C price is high enough to unfold its abatement potential (Gugler et al., 2021). Then, a commonly used cap-and-trade scheme provides a powerful system through which a limited amount of tradable and priced C allowances (cap) is considered for distribution among emitters, and they are further permitted to trade allowances with other emitters, government agencies or brokers (Ji et al., 2017). However, current C pricing mechanism addresses only a small portion of global emission, app. 20% (World Bank Group, 2019) leaving for instance private forests outside the cap-and-trade regulation (Dong et al., 2021). On the other hand, forest could provide a significant contribution to the grand effort needed to mitigate climate change and meet the Paris Agreement targets (e.g., Ekholm, 2020). Therefore, assessing the economic incentives for private forest owners to take part into climate change mitigation is a crucial action prior to initializing a C pricing mechanism to apply private forests. In this study three different C prices were used, namely 25, 50 and 75€/t CO2eq.—these values can be considered to capture the recent fluctuation of clearing prices for auctions of general allowances of the European Union Emissions Trading System, EU ETS (European Commission, 2021). Furthermore, in the light of recent studies (Ricke et al., 2018; Hintermayer, 2020) future C prices would rise rather than decline—suggesting that our results are cautious with respect to the level of financial incentives.
Prior to concluding the assumptions and constraints applied in this study need to be revealed and discussed. First, the starting point of the analyses was set to a point corresponding to a mature stand with beneficial features for CCF. In other words, the initial state for simulations represents favourable conditions to apply CCF straight away. This reduces generalizability of the results (Sinha et al., 2019 for “dependence of initial state”). On the other hand, such conditions (i.e., mature stands with features favourable for uneven-aged management) are quite common both in mineral soils and on peatlands in Finland. For instance, according to National Forest Inventory (NFI) 12 there are ca. 8.8 and 1.2 million hectares of spruce-dominated forests on mineral soils and peatlands in Southern Finland, respectively (Statistics database 2021, accessed 5 October 2021). Further, approximately 5.6 million hectares in Southern Finland are at least advanced thinning stands (Finnish forest statistics, 2020, Table 1.9) suggesting that there is a considerable amount of—perhaps tens of thousands of hectares—corresponding stands to the initial stand of this study. Second, one limitation of this study is the use of virtual, generated stands instead of replicated empirical stands. However, such an approach (applying virtual or generated stand structure) is commonly applied in stand-level simulation-based economic studies (e.g., Miettinen et al., 2020; Parkatti and Tahvonen, 2021). Furthermore, the key element here is to create as representative stands as possible for simulations (Honkaniemi et al., 2019) rather than rely on a vast number of repeated experiments to cover all possible growth conditions (cf. Tian et al., 2020). Anyway, our results cannot be generalized to stands that have an initial even-aged structure. In this case, it may be optimal to first conduct a clear-cut and then start a conversion towards CCF (Tahvonen and Rämö, 2016). In addition, our results cannot be generalized to poorer sites, in particular, stands dominated by pine trees that typically benefit from longer harvest intervals than spruce stands in CCF (Parkatti et al., 2019). Then we did not apply a stand-level optimization. This was mainly due to the primary goal of the study: to compare the financial incentives of identical CCF scenarios to mitigate climate change both on mineral soils and on peatlands. In a recent study (Juutinen et al., 2021) similar approach with various combinations of harvest interval and intensity (expressed as post-harvest BA) was applied to discover the profitability of CCF on peatland. Further another recent study (Serrano-Leon et al., 2021) assessed the financial impact of using genetically improved seed material in artificial regeneration in three European countries without applying stand-level optimization. A systematic comparison of alternative potential management regimes by using simulations is therefore a valuable option in many contexts. Finally, stand-level optimization would have resulted most likely different optimal management regimes for mineral soils and peatlands, thus making the comparison between mineral soils and peatlands difficult or even futile.
We conclude that the C pricing encourages milder harvesting in the CCF for both mineral soils and peatlands. However, the effect is stronger on mineral soils, causing significant changes in management already in low C prices. On peatlands, the interplay between stand growth, ground water level and soil GHG balance (Juutinen et al., 2021; Shanin et al., 2021) causes the harvests to be less intensive compared to mineral soils already at 0 C price, thus also the changes from C pricing are less drastic. Then, according to study results C sequestration in mature stands by applying CCF might not be financially lucrative, but in young and developing stands CCF provides financial incentives for private forest owners to participate in climate change mitigation, given the C price stays at low or moderate level. In current status quo—in the absence of functioning C credit system—authorities could advise private forest owners how to mitigate climate change cost-efficiently by introducing and providing management alternatives to traditional rotation forestry. Such alternatives, for instance CCF has been proven to be quite beneficial for both climate and private forest owners’ finance (Kellomäki et al., 2008; Zubizarreta-Gerendiain et al., 2015).
Data Availability Statement
The raw data supporting the conclusion of this article will be made available by the authors, without undue reservation.
Author Contributions
Conceptualization: AA, AJ, JR, VS and RM. Data curation: VS and JR. Formal analysis: AA, JR and AJ. Investigation: AA, AJ and JR. Methodology: AA, JR, AJ and VS. Supervision: RM and AJ. Validation: JR and VS. Visualization: JR. Roles/writing—original draft: AA, AJ and JR. Writing—review and editing: AA, AJ, JR, VS and RM.
Conflict of Interest
The authors declare that the research was conducted in the absence of any commercial or financial relationships that could be construed as a potential conflict of interest.
Publisher’s Note
All claims expressed in this article are solely those of the authors and do not necessarily represent those of their affiliated organizations, or those of the publisher, the editors and the reviewers. Any product that may be evaluated in this article, or claim that may be made by its manufacturer, is not guaranteed or endorsed by the publisher.
Acknowledgments
We acknowledge the Academy of Finland Flagship Programme and the Strategic Research Council for financial support (Projects 337655 and 336570).
Footnotes
1For a concise literature on C pricing shortfalls, see Stoll and Mehling (2021). Regarding unsolved issues related to SCC, see Belfiori’s (2017) demonstration on the discrepancy between optimal C tax and SCC, Nordhaus and Boyer (2003), Weitzman (2007), and Stern (2008) for the applied discount rate in assessing and Ricke et al. (2018) for heterogenous geography of climate damage and global SCC.
2In this study we compared the cost of C abatement between mineral soil and peatland. Technically this was done by first assessing the cost of additional C (expressed as €/tC02) related to a particular CCF with C pricing, compared to the best financial CCF without C pricing.
References
Asante, P., and Armstrong, G. (2016). Carbon Sequestration and the Optimal forest Harvest Decision under Alternative Baseline Policies. Can. J. For. Res. 46, 656–665. doi:10.1139/cjfr-2015-0222
Assmuth, A., and Tahvonen, O. (2018). Optimal Carbon Storage in Even- and Uneven-Aged Forestry. For. Pol. Econ. 87, 93–100. doi:10.1016/j.forpol.2017.09.004
Belfiori, M. E. (2017). Carbon Pricing, Carbon Sequestration and Social Discounting. Eur. Econ. Rev. 96, 1–17. doi:10.1016/j.euroecorev.2017.03.015
Bieroza, M. Z., Bol, R., and Glendell, M. (2021). What Is the deal with the Green Deal: Will the New Strategy Help to Improve European Freshwater Quality beyond the Water Framework Directive? Sci. Total Environ. 791, 148080. doi:10.1016/j.scitotenv.2021.148080
Boyce, J. K. (2018). Carbon Pricing: Effectiveness and Equity. Ecol. Econ. 150, 52–61. doi:10.1016/j.ecolecon.2018.03.030
Dixon, R. K., Solomon, A. M., Brown, S., Houghton, R. A., Trexier, M. C., and Wisniewski, J. (1994). Carbon Pools and Flux of Global forest Ecosystems. Science 263, 185–190. doi:10.1126/science.263.5144.185
Dong, L., Bettinger, P., and Liu, Z. (2021). Estimating the Optimal Internal Carbon Prices for Balancing forest wood Production and Carbon Sequestration: The Case of Northeast China. J. Clean. Prod. 281, 125342. doi:10.1016/j.jclepro.2020.125342
Ekholm, T. (2020). Optimal forest Rotation under Carbon Pricing and forest Damage Risk. For. Pol. Econ. 115, 102131. doi:10.1016/j.forpol.2020.102131
Eriksson, M. (2015). The Role of the forest in an Integrated Assessment Model of the Climate and the Economy. Clim. Change Econ. 06, 1550011. doi:10.1142/s2010007815500116
European Commission (2021). Report from the Commission to the European Parliament and the Council, on the Functioning of the European Carbon Market in 2020 Pursuant to Articles 10(5) and 21(2) of Directive 2003/87/EC (As Amended by Directive 2009/29/EC and Directive (EU) 2018/410). Available from: https://ec.europa.eu/clima/system/files/2021-10/com_2021_962_en.pdf (Accessed November 4, 2021).
Evison, D. (2017). The New Zealand Forestry Sector's Experience in Providing Carbon Sequestration Services under the New Zealand Emissions Trading Scheme, 2008 to 2012. For. Pol. Econ. 75, 89–94. doi:10.1016/j.forpol.2016.10.003
Finnish forest statistics (2020). Forest Resources. Available from: https://stat.luke.fi/sites/default/files/suomen_metsatilastot_2020_verkko.pdf (Accessed November 3, 2021).
Gren, I.-M., and Aklilu, A. Z. (2016). Policy Design for forest Carbon Sequestration: A Review of the Literature. For. Pol. Econ. 70, 128–136. doi:10.1016/j.forpol.2016.06.008
Gugler, K., Haxhimusa, A., and Liebensteiner, M. (2021). Effectiveness of Climate Policies: Carbon Pricing vs. Subsidizing Renewables. J. Environ. Econ. Manag. 106, 102405. doi:10.1016/j.jeem.2020.102405
Hintermayer, M. (2020). A Carbon price Floor in the Reformed EU ETS: Design Matters! Energy Policy 147, 111905. doi:10.1016/j.enpol.2020.111905
Honkaniemi, J., Ahtikoski, A., and Piri, T. (2019). Financial Incentives to Perform Stump Treatment against Heterobasidion Root Rot in Norway spruce Dominated Forests, the Case of Finland. For. Pol. Econ. 105, 1–9. doi:10.1016/j.forpol.2019.05.015
Hui, D., Deng, Q., Tian, H., and Luo, Y. (2017). Climate Change and Carbon Sequestration in forest Ecosystems. Handb. Clim. Chang. Mitig. Adapt. 2017, 555–594. doi:10.1007/978-3-319-14409-2_13
IPCC (2001). “Climate Change 2001: Mitigation,” in Contribution of Working Group III to the Third Assessment Report of the Intergovernmental Panel on Climate Change. Editors B. Metz, O. Davidson, R. Swart, and J. Pan (UK: Cambridge University Press), 305.
IPCC (2014). “Climate Change 2014: Synthesis Report,” in Contribution of Working Groups I, II and III to the Fifth Assessment Report of the Intergovernmental Panel on Climate Change Core Writing Team. Editors R. K. Pachauri, and L. A. Meyer (Geneva, Switzerland: IPCC), 151.
IPCC (2021). “Climate Change 2021: The Physical Science Basis,” in Contribution of Working Group I to the Sixth Assessment Report of the Intergovernmental Panel on Climate Change. Editors V. Masson-Delmotte, P. Zhai, A. Pirani, S. L. Connors, C. Péan, S. Bergeret al. (Cambridge University Press). In Press Available from: https://www.ipcc.ch/report/ar6/wg1/. In Press Available from: https://www.ipcc.ch/sr15/.
Jhariya, M. K., Banerjee, A., Meera, R. S., and Yadav, D. K. (2019). Sustainable Agriculture, forest and Environmental Management. Springer Nature Singapore. 978-981-13-6830-1.
Jhariya, M. K. (2017a). Vegetation Ecology and Carbon Sequestration Potential of Shrubs in Tropics of Chhattisgarh, India. Environ. Monit. Assess. 189 (10), 518. doi:10.1007/s10661-017-6246-2
Jhariya, M. K. (2017b). Influences of forest Fire on forest Floor and Litterfall Dynamics in Bhoramdeo Wildlife Sanctuary (C.G.), India. J. For. Environ Sci 33 (4), 330–341. doi:10.7747/JFES.2017.33.4.330
Ji, J., Zhang, Z., and Yang, L. (2017). Comparisons of Initial Carbon Allowance Allocation Rules in an O2O Retail Supply Chain with the Cap-And-Trade Regulation. Int. J. Prod. Econ. 187, 68e84. doi:10.1016/j.ijpe.2017.02.011
Juutinen, A., Ahtikoski, A., Lehtonen, M., Mäkipää, R., and Ollikainen, M. (2018a). The Impact of a Short-Term Carbon Payment Scheme on forest Management. For. Pol. Econ. 90, 115–127. doi:10.1016/j.forpol.2018.02.005
Juutinen, A., Ahtikoski, A., Mäkipää, R., and Shanin, V. (2018b). Effect of Harvest Interval and Intensity on the Profitability of Uneven-Aged Management of Norway spruce Stands. Forestry 91 (5), 589–602. doi:10.1093/forestry/cpy018
Juutinen, A., Shanin, V., Ahtikoski, A., Rämö, J., Mäkipää, R., Laiho, R., et al. (2021). Profitability of Continuous-Cover Forestry in Norway spruce Dominated Peatland forest and the Role of Water Table. Can. J. For. Res. 51, 859–870. doi:10.1139/cjfr-2020-0305
Kellomäki, S., Peltola, H., Nuutinen, T., Korhonen, K. T., and Strandman, H. (2008). Sensitivity of Managed Boreal Forests in Finland to Climate Change, with Implications for Adaptive Management. Phil. Trans. R. Soc. B 363, 2339–2349. doi:10.1098/rstb.2007.2204
Kellomäki, S., Strandman, H., and Peltola, H. (2019). Effects of Even-Aged and Uneven-Aged Management on Carbon Dynamics and Timber Yield in Boreal Norway spruce Stands: a forest Ecosystem Model Approach. Forestry 92, 635–647. doi:10.1093/forestry/cpz040
Komarov, A., Chertov, O., Zudin, S., Nadporozhskaya, M., Mikhailov, A., Bykhovets, S., et al. (2003). EFIMOD 2 – A Model of Growth and Elements Cycling of Boreal forest Ecosystems. Ecol. Model. 170 (2–3), 373–392. doi:10.1016/S0304-3800(03)00240-0
Komarov, A., Chertov, O., Bykhovets, S., Shaw, C., Nadporozhskaya, M., Frolov, P., et al. (2017). Romul_Hum Model of Soil Organic Matter Formation Coupled with Soil Biota Activity. I. Problem Formulation, Model Description, and Testing. Ecol. Model. 345, 113–124. doi:10.1016/j.ecolmodel.2016.08.007
Kuuluvainen, T., Tahvonen, O., and Aakala, T. (2012). Even-aged and Uneven-Aged forest Management in Boreal Fennoscandia: a Review. AMBIO 41, 720–737. doi:10.1007/s13280-012-0289-y
Lal, R. (2005). Forest Soils and Carbon Sequestration. For. Ecol. Manag. 220, 242–258. doi:10.1016/j.foreco.2005.08.015
Laurén, A., Palviainen, M., Launiainen, S., Leppä, K., Stenberg, L., Urzainki, I., et al. (2021). Drainage and Stand Growth Response in Peatland Forests-Description, Testing, and Application of Mechanistic Peatland Simulator SUSI. Forests 12 (3), 293. doi:10.3390/f12030293
Leppä, K., Hökkä, H., Laiho, R., Launiainen, S., Lehtonen, A., Mäkipää, R., et al. (2020a). Selection Cuttings as a Tool to Control Water Table Level in Boreal Drained Peatland Forests. Front. Earth Sci. 8, 576510. doi:10.3389/feart.2020.576510
Leppä, K., Korkiakoski, M., Nieminen, M., Laiho, R., Hotanen, J.-P., Kieloaho, A.-J., et al. (2020b). Vegetation Controls of Water and Energy Balance of a Drained Peatland forest: Responses to Alternative Harvesting Practices. Agric. For. Meteorol. 295, 108198. doi:10.1016/j.agrformet.2020.108198
Lindroos, A.-J., Derome, J., Mustajärvi, K., Nöjd, P., Beuker, E., and Helmisaari, H.-S. (2008). Fluxes of Dissolved Organic Carbon in Stand Throughfall and Percolation Water in 12 Boreal Coniferous Stands on mineral Soils in Finland. Boreal Environ. Res. 13 (Suppl. B), 22–34. http://hdl.handle.net/10138/235259
Lintunen, J., Rautiainen, A., and Uusivuori, J. (2021). Which Is More Important, Carbon or Albedo? Optimizing Harvest Rotations for Timber and Climate Benefits in a Changing Climate. Am. J Agri Econ. 104, 134–160. doi:10.1111/ajae.12219
Liski, J., Pussinen, A., Pingoud, K., Mäkipää, R., and Karjalainen, T. (2001). Which Rotation Length Is Favourable to Carbon Sequestration? Can. J. For. Res. 31, 2004–2013. doi:10.1139/x01-140
Lundmark, T., Poudel, B. C., Stål, G., Nordin, A., and Sonesson, J. (2018). Carbon Balance in Production Forestry in Relation to Rotation Length. Can. J. For. Res. 48, 672–678. doi:10.1139/cjfr-2017-0410
Lutz, C., and Meyer, B. (2009). Environmental and Economic Effects of post-Kyoto Carbon Regimes: Results of Simulations with the Global Model GINFORS. Energy Policy 37, 1758–1766. doi:10.1016/j.enpol.2009.01.015
Masson-Delmotte, V., Zhai, P., Pörtner, H.-O., Roberts, D., Skea, J., Shukla, P. R., et al. (2018). Global Warming of 1.5◦C an IPCC Special Report on the Impacts of Global Warming of 1.5◦C above Preindustrial Levels and Related Global Greenhouse Gas Emission Pathways, in the Context of Strengthening the Global Response to the Threat of Climate Change, Sustainable Development, and Efforts to Eradicate Poverty Summary for Policymakers. Science Officer Science Assistant Graphics Officer Working Group I Technical Support Unit.
Matthies, B. D., and Valsta, L. T. (2016). Optimal forest Species Mixture with Carbon Storage and Albedo Effect for Climate Change Mitigation. Ecol. Econ. 123, 95–105. doi:10.1016/j.ecolecon.2016.01.004
Mayer, M., Prescott, C. E., Abaker, W. E. A., Augusto, L., Cécillon, L., Ferreira, G. W. D., et al. (2020). Tamm Review: Influence of forest Management Activities on Soil Organic Carbon Stocks: A Knowledge Synthesis. For. Ecol. Manag. 466, 118127. doi:10.1016/j.foreco.2020.118127
Miettinen, J., Ollikainen, M., Aroviita, J., Haikarainen, S., Nieminen, M., Turunen, J., et al. (2020). Boreal Peatland Forests: Ditch Network Maintenance Effort and Water protection in a forest Rotation Framework. Can. J. For. Res. 50, 1025–1038. doi:10.1139/cjfr-2019-0339
Nieminen, M., Hökkä, H., Laiho, R., Juutinen, A., Ahtikoski, A., Pearson, M., et al. (2018). Could Continuous Cover Forestry Be an Economically and Environmentally Feasible Management Option on Drained Boreal Peatlands? For. Ecol. Manag. 424, 78–84. doi:10.1016/j.foreco.2018.04.046
Nordhaus, W. D., and Boyer, J. (2003). Warming the World: Economic Models of Global Warming. MIT Press.
Ojanen, P., Minkkinen, K., Alm, J., and Penttilä, T. (2010). Soil-atmosphere CO2, CH4 and N2O Fluxes in Boreal Forestry-Drained Peatlands. For. Ecol. Manag. 260 (3), 411–421. doi:10.1016/j.foreco.2010.04.036
Ojanen, P., Minkkinen, K., and Penttilä, T. (2013). The Current Greenhouse Gas Impact of Forestry-Drained Boreal Peatlands. For. Ecol. Manag. 289, 201–208. doi:10.1016/j.foreco.2012.10.008
Ojanen, P., and Minkkinen, K. (2019). The Dependence of Net Soil CO2 Emissions on Water Table Depth in Boreal Peatlands Drained for Forestry. Mires Peat 24, 1–27. doi:10.19189/MaP.2019.OMB.StA.1751
Olschewski, R., and Benítez, P. C. (2010). Optimizing Joint Production of Timber and Carbon Sequestration of Afforestation Projects. J. For. Econ. 16, 1–10. doi:10.1016/j.jfe.2009.03.002
Pan, Y., Birdsey, R. A., Fang, J., Houghton, R., Kauppi, P. E., Kurz, W. A., et al. (2011). A Large and Persistent Carbon Sink in the World's Forests. Science 333, 988–993. doi:10.1126/science.1201609
Parkatti, V.-P., Assmuth, A., Rämö, J., and Tahvonen, O. (2019). Economics of Boreal conifer Species in Continuous Cover and Rotation Forestry. For. Pol. Econ. 100, 55–67. doi:10.1016/j.forpol.2018.11.003
Parkatti, V.-P., and Tahvonen, O. (2020). Optimizing Continuous Cover and Rotation Forestry in Mixed-Species Boreal Forests. Can. J. For. Res. 50, 1138–1151. doi:10.1139/cjfr-2020-0056
Parkatti, V.-P., and Tahvonen, O. (2021). Economics of Multifunctional Forestry in the Sámi People homeland Region. J. Environ. Econ. Manag. 110, 102542. doi:10.1016/j.jeem.2021.102542
Pohjola, J., and Valsta, L. (2007). Carbon Credits and Management of Scots pine and Norway spruce Stands in Finland. For. Pol. Econ. 9, 789–798. doi:10.1016/j.forpol.2006.03.012
Pukkala, T., Lähde, E., Laiho, O., Salo, K., and Hotanen, J.-P. (2011). A Multifunctional Comparison of Even-Aged and Uneven-Aged forest Management in a Boreal Region. Can. J. For. Res. 41 (4), 851–862. doi:10.1139/x11-009
Pyörälä, P., Peltola, H., Strandman, H., Antti, K., Antti, A., Jylhä, K., et al. (2014). Effects of Management on Economic Profitability of forest Biomass Production and Carbon Neutrality of Bioenergy Use in Norway spruce Stands under the Changing Climate. Bioenerg. Res. 7 (1), 279–294. doi:10.1007/s12155-013-9372-x
Raj, A., and Jhariya, M. K. (2021). Carbon Storage, Flux and Mitigation Potential of Tropical Sal Mixed Deciduous forest Ecosystem in Chhattisgarh, India. J. Environ. Manage. 293 (1), 112829. doi:10.1016/j.jenvman.2021.112829
Riahi, K., van Vuuren, D. P., Kriegler, E., Edmonds, J., O’Neill, B. C., Fujimori, S., et al. (2017). The Shared Socioeconomic Pathways and Their Energy, Land Use, and Greenhouse Gas Emissions Implications: An Overview. Glob. Environ. Change 42, 153–168. doi:10.1016/j.gloenvcha.2016.05.009
Ricke, K., Drouet, L., Caldeira, K., and Tavoni, M. (2018). Country-level Social Cost of Carbon. Nat. Clim Change 8, 895–900. doi:10.1038/s41558-018-0282-y
Riviere, M., and Caurla, S. (2021). Landscape Implications of Managing Forests for Carbon Sequestration. Forestry 94, 70–85. doi:10.1093/forestry/cpaa015
Sarkkola, S., Koivusalo, H., Laurén, A., Kortelainen, P., Mattsson, T., Palviainen, M., et al. (2009). Trends in Hydrometeorological Conditions and Stream Water Organic Carbon in Boreal Forested Catchments. Sci. Total Environ. 408 (1), 92–101. doi:10.1016/j.scitotenv.2009.09.008
Serrano-León, H., Ahtikoski, A., Sonesson, J., Fady, B., Lindner, M., Meredieu, C., et al. (2021). From Genetic Gain to Economic Gain: Simulated Growth and Financial Performance of Genetically Improved Pinus Sylvestris and Pinus pinaster Planted Stands in France, Finland and Sweden. Forestry 94 (4), 512–525. doi:10.1093/forestry/cpab004
Shanin, V., Mäkipää, R., Shashkov, M., Ivanova, N., Shestibratov, K., Moskalenko, S., et al. (2015). New Procedure for the Simulation of Belowground Competition Can Improve the Performance of forest Simulation Models. Eur. J. For. Res 134 (6), 1055–1074. doi:10.1007/s10342-015-0909-8
Shanin, V., Grabarnik, P., Shashkov, M., Ivanova, N., Bykhovets, S., Frolov, P., et al. (2020). Crown Asymmetry and Niche Segregation as an Adaptation of Trees to Competition for Light: Conclusions from Simulation Experiments in Mixed Boreal Stands. MCFNRS 12 (1), 26–49. doi:10.5281/zenodo.3759256
Shanin, V., Juutinen, A., Ahtikoski, A., Frolov, P., Chertov, O., Rämö, J., et al. (2021). Simulation Modelling of Greenhouse Gas Balance in Continuous-Cover Forestry of Norway spruce Stands on Nutrient-Rich Drained Peatlands. For. Ecol. Manag. 496, 119479. doi:10.1016/j.foreco.2021.119479
Sinha, A., Rämö, J., Malo, P., Kallio, M., and Tahvonen, O. (2019). Optimal Management of Naturally Regenerating Uneven-Aged Forests. Eur. J. Oper. Res. 256, 886–900. doi:10.1016/j.ejor.2016.06.071
Statistics database (2021). Forest Resources. Available from: http://statdb.luke.fi/PXWeb/pxweb/en/LUKE/LUKE__04%20Metsa__06%20Metsavarat/1.02_Kankaat_ja_suot_metsatalousmaalla.px/table/tableViewLayout2/(Accessed November 5, 2021).
Stern, N., Peters, S., Bakhshi, V., Bowen, A., Cameron, C., Catovsky, S., et al. (2006). Stern Review on the Economics of Climate Change. Cambridge: Cambridge University Press.
Stern, N. (2008). The Economics of Climate Change. Am. Econ. Rev. 98 (2), 1–37. doi:10.1257/aer.98.2.1
Stoll, C., and Mehling, M. A. (2021). Climate Change and Carbon Pricing: Overcoming Three Dimensions of Failure. Energ. Res. Soc. Sci. 77, 102062. doi:10.1016/j.erss.2021.102062
Tahvonen, O. (2016). Economics of Rotation and Thinning Revisited: the Optimality of Clearcuts versus Continuous Cover Forestry. For. Pol. Econ. 62, 88–94. doi:10.1016/j.forpol.2015.08.013
Tahvonen, O., Rämö, J., and Mönkkönen, M. (2019). Economics of Mixed-Species Forestry with Ecosystem Services. Can. J. For. Res. 49 (10), 1219–1232. doi:10.1139/cjfr-2018-0514
Tahvonen, O., and Rämö, J. (2016). Optimality of Continuous Cover vs. clear-cut Regimes in Managing forest Resources. Can. J. For. Res. 46, 891–901. doi:10.1139/cjfr-2015-0474
The World Bank (2020). What Is Carbon Pricing. Available from: https://carbonpricingdashboard.worldbank.org/what-carbon-pricing (Accessed July 6, 2021).
Tian, X., Sun, S., Mola-Yudego, B., and Cao, T. (2020). Predicting Individual Tree Growth Using Stand-Level Simulation, Diameter Distribution, and Bayesian Calibration. Ann. For. Sci. 77, 57. doi:10.1007/s13595-020-00970-0
Väätäinen, K., Lamminen, S., Siren, M., Ala-Ilomäki, J., and Asikainen, A. (2010). Ympärivuotisen Puunkorjuun Kustannusvaikutukset Ojitetuilla Turvemailla—Korjuuyrittäjän Simulointitutkimus [All-Year Logging Costs for Drained Peatland Forests — Simulation Study Based on Entrepreneurs]. Working Pap. Finnish For. Res. Inst. 184, 1–57. Available from: http://www.metla.fi/julkaisut/workingpapers/2010/mwp184.pdf (Accessed May 6, 2020).
van den Bijgaart, I., Gerlagh, R., and Liski, M. (2016). A Simple Formula for the Social Cost of Carbon. J. Environ. Econ. Manag. 77, 75–94. doi:10.1016/j.jeem.2016.01.005
van Kooten, G., Binkley, C., and Delcourt, G. (1995). Effect of Carbon Taxes and Subsidies on Optimal forest Rotation Age and Supply of Carbon Services. Am. J. Agric. Econ. 77 (2), 365–374. doi:10.2307/1243546
Verkerk, P. J., Costanza, R., Hetemäki, L., Kubiszewski, I., Leskinen, P., Nabuurs, G. J., et al. (2020). Climate-Smart Forestry: the Missing Link. For. Pol. Econ. 115, 102164. doi:10.1016/j.forpol.2020.102164
Weishampel, P., Kolka, R., and King, J. Y. (2009). Carbon Pools and Productivity in a 1-km2 Heterogeneous forest and Peatland Mosaic in Minnesota, USA. For. Ecol. Manag. 257 (2), 747–754. doi:10.1016/j.foreco.2008.10.008
Weitzman, M. L. (2007). A Review of the Stern Review on the Economics of Climate Change. J. Econ. Lit. 45 (3), 703–724. doi:10.1257/jel.45.3.703
Zengin, H., and Ünal, M. E. (2019). Analyzing the Effect of Carbon Prices on wood Production and Harvest Scheduling in a Managed forest in Turkey. For. Pol. Econ. 103, 28–35. doi:10.1016/j.forpol.2017.10.017
Zubizarreta-Gerendiain, A., Garcia-Gonzalo, J., Strandman, H., Jylhä, K., and Peltola, H. (2016). Regional Effects of Alternative Climate Change and Management Scenarios on Timber Production, Economic Profitability, and Carbon Stocks in Norway spruce Forests in Finland. Can. J. For. Res. 46, 274–283. doi:10.1139/cjfr-2015-0218
Keywords: continuous cover forestry, cost of carbon abatement, mineral soil, peatland, net present value, rotation forestry
Citation: Ahtikoski A, Rämö J, Juutinen A, Shanin V and Mäkipää R (2022) Continuous Cover Forestry and Cost of Carbon Abatement on Mineral Soils and Peatlands. Front. Environ. Sci. 10:837878. doi: 10.3389/fenvs.2022.837878
Received: 17 December 2021; Accepted: 28 March 2022;
Published: 14 April 2022.
Edited by:
Faik Bilgili, Erciyes University, TurkeyReviewed by:
Manoj Kumar Jhariya, Sant Gahira Guru Vishwavidyalaya, IndiaIngo Schöning, Max Planck Institute for Biogeochemistry, Germany
Copyright © 2022 Ahtikoski, Rämö, Juutinen, Shanin and Mäkipää. This is an open-access article distributed under the terms of the Creative Commons Attribution License (CC BY). The use, distribution or reproduction in other forums is permitted, provided the original author(s) and the copyright owner(s) are credited and that the original publication in this journal is cited, in accordance with accepted academic practice. No use, distribution or reproduction is permitted which does not comply with these terms.
*Correspondence: Anssi Ahtikoski, anssi.ahtikoski@luke.fi