- 1Institut Des Géosciences De l'Environnement (IGE), University Grenoble Alpes, CNRS, IRD, Grenoble INP, Grenoble, France
- 2GEOTOP/ Université du Québec à Montréal (UQAM), Montréal, ON, Canada
Air pollution, a complex cocktail of different components, exerts an influence on climate/human; health both locally and away from source regions. The issue of air pollution is often closely linked; to carbonaceous aerosols, the assessment of climate/air quality/health impact of which remains associated with large uncertainties. Black carbon (BC)—a product of incomplete combustion—is a potent climate warming agent and one of the central components to this issue. An accurate; knowledge of BC emitting sources is necessary for devising appropriate mitigation strategies and; policies to reduce the associated climate/environmental burden. The radiocarbon isotope (14C or carbon-14) fingerprinting allows for an unambiguous and quantitative constraining of the BC sources and is therefore a well-popularized method. Here, we review the existing analytical techniques for the isolation of BC from a filter matrix for conducting 14C-based investigations. This work summarizes the protocols in use, provides an overarching perspective on the state-of- the-art and recommendations for certain aspects of future method development.
Introduction
The World Health Organization recognizes air pollution as the single largest global environmental health threat accounting for millions of premature deaths annually (Lelieveld et al., 2015; World Health Organization, 2021). One of the criteria for reducing air pollution is to incorporate policies targeting certain components e.g., particulate matter, PM (PM2.5 for aerodynamic size ≤ 2.5 µm) (Liang et al., 2016). While the meta-analysis of PM carried out in various forms provides much needed information about possible origins and sources, albeit with high uncertainties, it is limited in providing only a “bird’s-eye view” (Liang et al., 2016). Given that a complex cocktail of noxious pollutants makes up the PM, a microscopic approach is often needed.
Carbonaceous aerosols constitute between 20 and 40% of PM2.5 depending on the location (Pöschl, 2005). The overall carbon content or total carbon (TC) in aerosols can be grouped as 1) a fraction referred to as carbonate carbon (CC) or inorganic carbon, existing mostly as calcium carbonate. CC can contribute up to 40% of the PM10 mass (Sillanpaä et al., 2005), depending upon the area of study or the meteorological conditions (e.g., soil types, dust events). CC decomposes at relatively high temperatures and therefore can affect the estimation of the other fractions of the TC depending on the thermal protocol used (Karanasiou et al., 2011), 2) a fraction referred to as organic carbon (OC), consisting mostly of low-molecular- weight hydrocarbons and their derivatives, which is largely colourless, thermally unstable, soluble in water and organic solvents, 3) a fraction referred to as elemental carbon (EC), that is made up of small spherules, which is refractory (vaporization temperature ∼4000 K), strongly light-absorbing, and insoluble in water and organic solvents (Petzold et al., 2013). While a generally agreed usage of the term “EC” is restricted to thermal methods, the term “black carbon (BC)” is employed when speaking strictly in terms of optical properties. The semantics of the term “BC” is still very much debated (Petzold et al., 2013), yet BC remains the more popularized umbrella term. Hereafter, we stick to this term whenever feasible.
BC is a product of the incomplete combustion of fossil fuels (e.g., from traffic, coal and petroleum-fuelled powerplant emissions) or biomass (including biofuel e.g., wood burning, agricultural waste burning) and is ubiquitous in the Earth system (Bond et al., 2013). By directly absorbing the short-wave radiation, BC exerts a warming effect on the atmosphere, and by deposition in the cryosphere affects the albedo leading to an enhanced melting of snow and ice (direct effects). Other than that, BC also affects cloud processes e.g., by changing the number of cloud droplets, particle number in liquid and ice clouds (indirect effects). The location of BC within/below/above clouds alters the cloud distribution (semidirect effects) (Myhre et al., 2013). BC has also been implicated in a multitude of additional regional effects such as disturbance of precipitation patterns, surface dimming, affecting agriculture and freshwater supply thereby the socio-economic sphere (Ramanathan and Carmicheal, 2008; Bond et al., 2013).
Despite several advancements in the measurement techniques for BC (Lack et al., 2014), the amplitude of BC-related perturbations on climate remains associated with large uncertainties and more so at regional levels with high BC loadings e.g., S Asia (Rana et al., 2019). The uncertain contributions from different emission sources (e.g., fossil fuel combustion vs biomass burning) in currently employed bottom-up emission inventories—serving as input for models and policies—is one of the likely suspects for modelling uncertainties (Zhao et al., 2011; Dasari et al., 2020). Several methods have been developed over the years for the source apportionment of BC, however, each with its own challenges (Briggs and Long, 2016). The most commonly used methods for BC source apportionment include the macro-tracer method, Chemical Mass Balance (CMB), Positive Matrix Factorization (PMF), the aethalometer method, and various specialized models (Briggs and Long, 2016). Each method has its own strengths and limitations. While some (e.g., the aethalometer method, macro-tracer method) can only apportion major BC sources but not the different source classes, others (e.g., CMB, PMF) require relatively vast datasets, and analyses involving a multitude of ancillary measurements of e.g., trace gases/organic compounds/speciated PM. In contrast, methods such as the aethalometer and macro-tracer methods have minimal data requirements. While these aspects relate to the mechanistic side of the method, the performance aspect also has to be considered. In general, the best-performing BC source apportionment methods are not consistent between studies as the performance varies between sites and/or time periods and depends highly on the available input data (Briggs and Long, 2016). As such, the uncertainties associated with the source estimation of BC remain high irrespective of the chosen method.
In contrast, the radiocarbon (reported as Δ14C) isotope-based source apportionment has proven to be useful in quantitatively constraining with high-precision the fossil fuel combustion-vs biomass burning-derived BC fractions and as such has been used in a wide variety of environments (Szidat et al., 2006; Zencak et al., 2007; Gustafsson et al., 2009; Fushimi et al., 2011; Heal et al., 2011; Bernardoni et al., 2013; Dusek et al., 2013; Bosch et al., 2014; Andersson et al., 2015; Li et al., 2016; Winiger et al., 2016).
While the unambiguous distinction between fossil fuel combustion-derived and biomass burning derived-BC remains the prime strength of radiocarbon-isotope analysis, the analytical techniques for isolating BC from the aerosol filter matrix to conduct such an analysis span a wide range, mostly lacking commonality in approach (Zhang et al., 2021). This is highly evidenced in the results from recent inter-comparison exercises of 14C in carbonaceous aerosols wherein a good agreement for OC and TC has been reported between the different laboratories, but a high variability was found for EC (Szidat et al., 2013; Zenker et al., 2017). While it has been suggested on several different occasions that observation-based 14C constrained BCFossil vs BCBiomass can be compared directly with modelled BCFossil vs BCBiomass estimates (e.g., Winiger et al., 2016; Dasari et al., 2020), this may simply not represent a “realistic” comparison when conducted on a global dataset—the lack of commonality among the different analytical techniques affects the isolated/recovered amount of BC for 14C analysis and with the employed post-corrections on the 14C results, together, the observed 14C constrained BCFossil vs BCBiomass (Zhang et al., 2012; Zenker et al., 2017). Furthermore, the differences can be pronounced if the charring of OC is not thoroughly investigated or addressed (Yu et al., 2002; Elmquist et al., 2006; Zhang et al., 2012). It is therefore imperative to probe the similarities and differences in the analytical techniques presently in use for the isolation of BC. Hence, we conducted a review of all such analytical techniques with the sole intention of making the user aware of their associated caveats to be able to make an informed decision regarding the choice of a technique. Here, we review approaches that build on the commercially available thermo-optical OC-EC analyzer (Model 4L, Sunset Laboratory Inc., Tigard, OR, United States) for BC isolation, which has been the community standard for such analysis (Zhang et al., 2012; Andersson et al., 2015; Liu et al., 2017; Huang et al., 2021), as well as include approaches using in-house developed systems (e.g., Bernardoni et al., 2013; Dusek et al., 2014). We highlight the need for reporting specific ancillary information with Δ14C results for improving transparency in the conditions in which BC was isolated. Furthermore, we argue for the need for reporting recoveries for samples prepped for BC isotope analysis. Lastly, we make recommendations for improving one of the techniques.
Measurement, Analysis and Reporting of Radiocarbon
Naturally occurring radiocarbon (referred to as 14C or carbon-14) is produced when high energy neutrons from spallation reactions bombard nitrogen atoms in the upper atmosphere (Figure 1). The resulting 14C then combines with atmospheric oxygen to form carbon monoxide, over 95% of which then rapidly oxidize to form carbon dioxide (14CO2) (Libby, 1946). This 14CO2 gets incorporated into the biosphere and ocean carbon reservoirs–in plants by photosynthesis, in animals which consume plants and so on (Turnbull et al., 2016). Upon the death and burial of plants and animals, the wood and bones lose their 14C content as it changes back to 14N through beta radioactive decay — 14C decays with a half-life of 5,730 ± 40 years (Arnold and Libby, 1949; Levin and Hesshaimer, 2000). A sample in which 14C is no longer detectable is said to be “radiocarbon dead” (Ramsey, 2008). It is this property of 14C that is most useful for ‘dating’ BC aerosols—fossil sources are completely depleted in carbon-14 whereas biomass sources have a distinct non-nil carbon-14 signature that reflects their integrated period of biomass photosynthesis and storage (Levin and Hesshaimer, 2000; Ramsey, 2008). Therefore, the radiocarbon signature of BC in an atmospheric aerosol sample allows for unambiguously deconvoluting the relative contributions from fossil fuel vs biomass combustion (dead-C vs. modern-C) (Ziolkowski and Druffel, 2009).
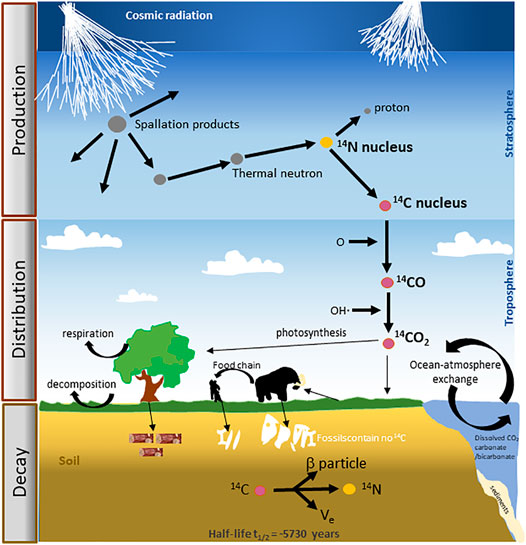
FIGURE 1. The schematic of mechanisms showing production-to-decay of radiocarbon (14C). This. can be briefly explained in four steps: 1) Cosmic rays bombard the upper atmosphere and send a. cascade of neutrons. 2) Upon striking atoms of nitrogen, the main component of Earth’s atmosphere, they transform some of 14N into Carbon-14 or 14C. 3) Carbon-14 creates radioactive. Carbon dioxide by readily combining with oxygen in the atmosphere which is then absorbed in the. biosphere by plants and enters the food chain. 4) Upon death and burial, the plants/organisms stop. absorbing Carbon-14 and its existing store slowly decays thereby acting as a ‘clock’ for dating.
The 14C levels in the modern atmosphere have been altered through artificial means: anthropogenic emissions of fossil fuels emit 14C-depleted carbon into the atmosphere, thereby diluting the proportion of 14C relative to 12C, whereas nuclear bomb testings in the mid-20th century have doubled the 14C content of CO2 in the Northern Hemisphere (Turnbull et al., 2016). At present, the levels of 14C in the atmosphere based on recent measurements show a declining trend (Graven, 2015).
Measuring 14C is challenging as one carbon-14 atom exists in living material for every 1,000,000,000,000 (or 1 in a trillion) carbon-12 atoms (Arnold and Libby, 1949; Levin and Hesshaimer, 2000). From the early 1940 s to the 1960 s, the detection of carbon-14 relied on decay-counting methods, such as the Geiger-Müller counter (Nydal 1962) or liquid scintillation counting (Anderson et al., 1951), until the 1970 s, when the accelerator mass spectrometry (AMS) was developed (Ramsey, 2008; Ziolkowski and Druffel, 2009). This enabled direct counting and reduced the sample size requirements by ∼106 times compared to its predecessors (Ramsey, 2008). The AMS data is reported as Fraction Modern (fm) which in brief is a measurement of the deviation of the 14C/12C ratio of a sample (that has 14C/12C normalized to a δ13CVPDB value of −25‰) and a standard having 95% of the radiocarbon concentration (in AD 1950) of NBS Oxalic Acid I (SRM 4990B, OX-I) normalized to δ13CVPDB = −19‰ (Olsson, 1970; Stuiver and Polach, 1977; Stuiver, 1980).
Where AS is the activity in the sample and AOX1 is the activity in the standard.
The Δ14C is then calculated by incorporating an age correction using an arbitrary standard having the best estimate of the 14C activity in the year 1950 if not affected by fossil or bomb C
Where λ is the inverse of the true mean-life of radiocarbon (0.00012097) and y is the year of sample collection.
The measurement and analysis procedures for radiocarbon undertaken in an AMS follows several steps. Briefly, gaseous CO2 samples are introduced into a vacuum line where further purification takes place using multiple cryotraps. The sample is then transferred to a heated reaction chamber, where it is transformed into graphite through the Bosch reaction. The graphite is then pressed into targets on a holder and loaded into the AMS unit alongside standards and blanks for the 14C measurement. To characterize the graphitization, handling, and AMS analysis, relevant standards (oxalic acid II as modern carbon and a second standard for fossil carbon), with similar size ranges to the samples, are processed into graphite. A complete description of the process and the details of AMS functioning is beyond the scope of this review and can be found elsewhere (e.g., Fifield, 1999; Hellborg and Skog, 2008; NOSAMS (whoi.edu)). With the development of a compact AMS, the MICADAS (MIni CArbon DAting System) (Synal et al., 2007), it has become possible to introduce the sample as CO2 (g). In this set-up, the CO2 is introduced through a capillary fitted to a versatile gas interface system (Fahrni et al., 2013; Wacker et al., 2013) into specially designed gas cathodes. The interface produces a gaseous mixture of CO2 with He under a constant pressure. This gas mixture is fed into the ion source wherein the radiocarbon isotope analysis is conducted.
Thermal-Optical Analysis and Isolation of Black Carbon
The most common approach for the quantification of OC and EC fractions in an aerosol sample is the thermo-optical analysis (Karanasiou et al., 2015). In this method, from a quartz filter sample that has collected enough aerosol material, OC is first evaporated under helium (inert atmosphere), following which EC is combusted in a helium + oxygen phase through a series of temperature ramps, respectively. Presently, several protocols (e.g., NIOSH870, EUSAAR, IMPROVE) with a variety of temperature and time settings exist (Panteliadis et al., 2015). An intercomparison exercise involving 17 European laboratories has demonstrated that the repeatability and reproducibility (expressed as relative standard deviations) for TC were 11 and 15% for EUSAAR 2 and 9.2 and 12% for NIOSH870 protocols. For EC, these were 15 and 20% for EUSAAR2 and 20 and 26% for NIOSH870, respectively (Panteliadis et al., 2015). Furthermore, total optical transmittance calculated EC for NIOSH870 was found to be 20% lower than for EUSAAR2. A relationship was also derived for ECNIOSH870 = 0.80*ECEUSAAR2 (Panteliadis et al., 2015). However, an international agreement for a standard reference method is yet to be agreed upon by the scientific community.
Even though OC and EC are considered as two different entities, they represent a chemical continuum (Elmquist et al., 2006; Saleh et al., 2018). To correct for possible artefacts during the course of OC-EC quantification, a laser signal continuously monitors the transmission or/and reflectance of light of the filter sample (Moosmüller et al., 2009; Lack et al., 2014). The main artefact is the pyrolysis of OC during the evaporation step, which produces char that is chemically similar to EC and can be only removed by combustion (Yu et al., 2002; Elmquist et al., 2006). The laser attenuation-optimized split point during the analysis is accepted as the operational boundary between OC and EC in this method (Moosmüller et al., 2009; Lack et al., 2014). However, in a true sense, there is no clear thermo-chemical boundary separating the “refractory OC” from the ‘less refractory EC’ in the continuum (Saleh et al., 2018; Saleh, 2020). As such, pre-treatment of the filters has been widely advocated and employed to reduce the mis-quantification of pyrolyzed OC as EC. If not corrected, this artefact causes an underestimation of the OC content and an overestimation of the EC (Subramanian et al., 2006). 208
Here, we discuss six different analytical techniques (ATs; Table 1) that have been used for isolating EC (BC) for radiocarbon analysis: AT 1 (Gustafsson et al., 2009; Andersson et al., 2015), AT 2 (Zhang et al., 2012), AT 3 (Dusek et al., 2014; Zenker et al., 2017), AT 4 (Bernardoni et al., 2013), AT 5 (Liu et al., 2017), AT 6 (Huang et al., 2021).
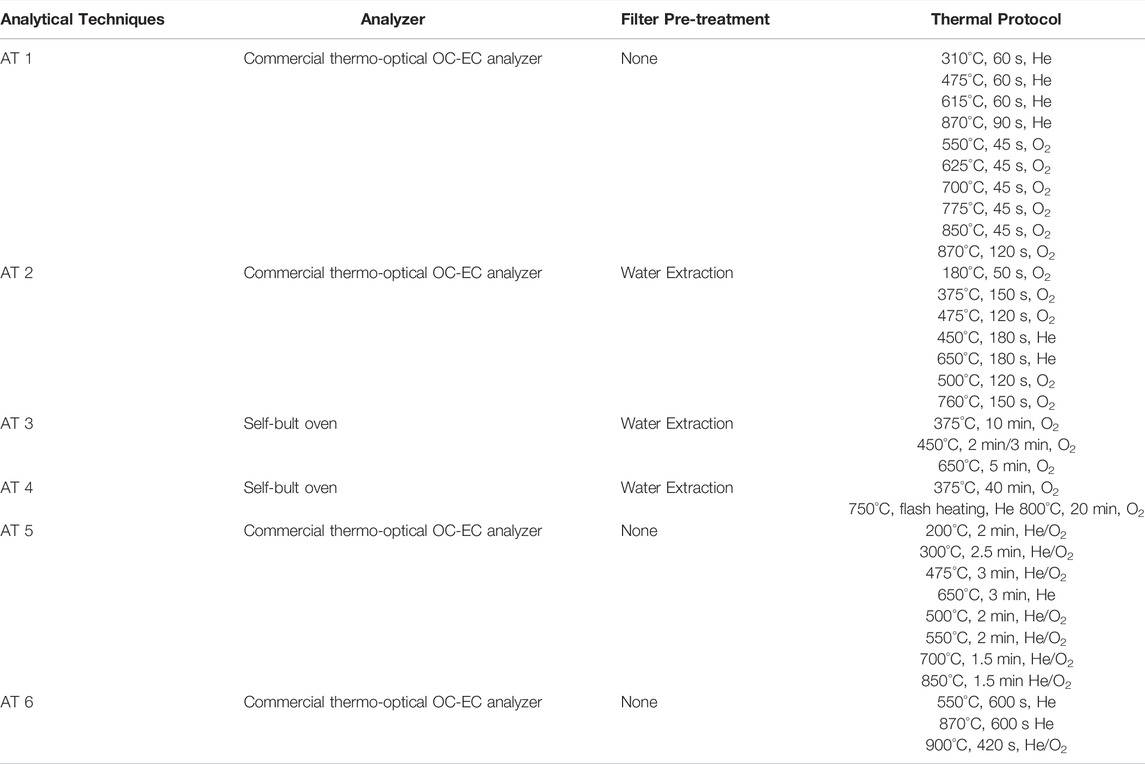
TABLE 1. A list of the reviewed and currently used analytical techniques (ATs) for the isolation of BC from a filter matrix for radiocarbon analysis. AT 1 (Gustafsson et al., 2009; Andersson et al., 2015), AT 2 (Zhang et al., 2012), AT 3 (Dusek et al., 2014; Zenker et al., 2017), AT 4 (Bernardoni et al., 2013), AT 5 (Liu et al., 2017), AT 6 (Huang et al., 2021).
AT 1: This analytical technique relies on the widely used National Institute for Occupational Safety and Health (NIOSH 5040; Birch and Cary, 1996) protocol (see Table 1). Firstly, the OC- EC instrument is modified such that the CO2 generated by the oxidation of OC or/and EC in the main oven with MnO2 as the catalyst can be isolated without being sent to the methanator oven (used for quantification by flame ionization detector FID) at any stage. An in-house developed cryotrapping system is then used to isolate the CO2 for radiocarbon analysis. The system operates with a timer and switching valve such that the EC-derived CO2 is diverted to the cryotrapping system instead of the methanator at the desired time during the run. This system contains a magnesium perchlorate (Mg(ClO4)2) trap and a silver (Ag) wool trap for removing water vapor and halogens, respectively. The purified CO2 is then collected into a liquid nitrogen trap and isolated in glass ampules once the required amount (∼50 μg C) of EC is harvested. The CO2 collected in this manner in the glass ampules is then analyzed for radiocarbon at an AMS facility (e.g., NOSAMS).
An inherent assumption in this AT is that the charring carbon is corrected automatically using the laser signal and that the carbon evolving after the split line is identified as BC. As part of this set up the time taken for gases to travel from the laser to the FID is monitored along with the split times and the transit times. Finally, the valve switch time (VT) is calculated [VT = split time—(transit time laser to FID) + (transit time laser to switching valve)]. The VT is key for this analytical technique as this largely impacts the trapping of the generated CO2 as the timer for operation of the switching to the cryotrap system is set accordingly. No pretreatment of the filter is conducted in this technique.
AT 2: This analytical technique relies on the Swiss4S protocol (see details in Table 1), which essentially is a 4-step process and builds on previous research with the two-step Heating system for the EC/OC Determination Of Radiocarbon in the Environment (THEODORE; Szidat et al., 2004). The first three steps are dedicated towards the removal of OC (specially the water-insoluble OC). The final step is for the combustion of the remaining EC. Pretreatment of the filter samples in this technique requires extraction with ultrapure water and drying for 6 h. The dried filter sample is then introduced in the thermo-optical OC-EC analyzer and the Swiss 4S protocol is run. The CO2 evolved from the EC phase is diluted with He to 5% CO2 in a gas inlet system. The CO2/He mixture is fed directly into a MIni CArbon DAting System (MICADAS) AMS at a constant rate for radiocarbon analysis (Salazar et al., 2015).
AT 3: This analytical technique uses a two-step protocol (referred to as 2stepCIO) that separates the carbonaceous fractions of the aerosol samples. This is executed on an in-house developed aerosol combustion system which consists of an extraction and a purification line, separated by a needle valve. The two lines are kept at different pressures: the extraction line at around 1,050 hPa and the purification line below 20 hPa. The filter sample is loaded in the main part of the extraction line which houses three ovens. Sample is combusted in the first and second oven under a pure O2 flow, while the third oven ensures the full oxidation of remaining incomplete combustion products. BC is isolated when the water-extracted filter sample is inserted into the reaction tube for 10 min at 375°C in the first oven for OC combustion, followed by 2 min at 450°C in the second oven for removing the most refractory OC and a part of the EC (which therefore always leads to a recovery rate of <100%). Finally, the sample is combusted for 5 min at 650°C for EC combustion. The CO2 formed during this final step is isolated in a cryogenic trap built in the purification line and transferred to a flask containing phosphorus pentoxide (P4O10) to remove traces of water vapor. Lastly, the CO2 is stored in flame-sealed glass ampules before conducting the AMS analysis.
AT 4: This analytical technique uses a dedicated sample preparation-graphitization line for aerosol samples. Water-extracted filter samples are combusted in a self-constructed oven. As a first step, OC is removed by the pre-combustion of the sample in purified O2 at 375°C for 40−min, followed by a subsequent flash heating at 750°C in purified He. Following this, EC combustion is accomplished by heating the oven to 800°C for 20 min under a constant O2 flow with a CuO catalyst to ensure complete combustion. The generated CO2 is passed through chemical and thermal traps to remove halogens, sulphate and H2O, N2O, respectively. This purified CO2 is then transferred to the AMS for radiocarbon analysis.
AT 5: This analytical technique relies on the European Supersites for Atmospheric Aerosol Research (EUSAAR_2; Cavalli et al., 2010) protocol, and its temperature program is set to 200°C for 120 s, 300°C for 150 s, 450°C for 180 s, and 650°C for 180 s in He, and then 500°C for 120 s, 550°C for 120 s, 700°C for 70 s, and 850°C for 80 s in a He/O2 mixture, respectively. Upon a thorough investigation of mass recoveries and the removal of water-soluble and water-insoluble OC, the EUSAAR 2 protocol was optimized to remove all of OC with minimal effect on EC, without any filter pretreatment, using an intermediate step at 475°C. In the thermo-optical OC-EC analyzer, the filter sample is heated for 120 s at 200 °C, 150 s at 300°C, and 180 s at 475°C in an oxidative atmosphere (10% oxygen, 90% helium) and 180 s at 650°C in helium to remove all of the OC, then the remaining EC is extracted with the EUSAAR protocol. The EC-derived CO2 is then guided through a high vacuum setup wherein gaseous H2O and other contaminants are removed using a dry ice/ethanol trap. The CO2 is finally trapped by liquid nitrogen and transferred to a glass/quartz ampule for AMS analysis.
AT 6: This analytical technique relies on the EnCan-Total- 900 (ECT9) protocol (Huang et al., 2006) which was originally developed for quantifying OC and EC, along with the analysis of their stable carbon isotope compositions (δ13C). The ECT9 protocol has been employed since 2006 for monitoring carbonaceous aerosols in the Arctic at the Alert station, as part of the Canadian Aerosol Baseline Measurements Network operated by Environment and Climate Change Canada. In principle, the ECT9 protocol is different from the standard thermo-optical protocols (e.g., NIOSH, EUSAAR) as it aims to remove the pyrolyzed OC (PyOC, char) along with carbonate carbon (CC) using an intermediate temperature step in pure He via high-temperature evaporation at 870°C. This protocol does not use either reflectance or transmittance signals and uses relatively longer retention times to isolate the OC, PyOC + CC, and EC components. Specifically, the sample analysis in ECT9 protocol proceeds in three steps: firstly, OC is removed at 550°C for 600 s in pure He, followed by the removal of PyOC 293 + CC at 870°C for 600 s in pure He, and finally EC is combusted at 900°C for 420 s in a mixture of 2% O2 with 98% He. The oxidation to CO2 occurs in a furnace containing MnO2 maintained at 870°C. This CO2 is then cryo-trapped with liquid N2 in a U-shaped glass trap which is introduced in a vacuum line for further purification by sequential distillation using three cryo-traps. Purified CO2 is flame-sealed in glass ampules for AMS analysis.
Perspective on the State-of-The-Art and Recommendations
We note that radiocarbon, 14C, analysis is a powerful tool with a unique potential to quantitatively distinguish fossil fuel sources (fm = 0) from biomass-burning and biogenic emissions (fm > 1 in the present-day living biosphere and atmospheric CO2). Furthermore, we conjecture that thermal treatment remains the most common method used to separate OC from BC (EC) for 14C measurements. However, in our review we find that challenges remain regarding the chemical delineation of the OC and EC fractions as neither of them are a discrete chemical species, but a chemical continuum. Here, we identified six different BC isolation methods that are currently used for radiocarbon analysis of BC worldwide. Detailed protocols show that they operate over a large range of temperature settings as well as filter pre-treatments (Table 1). In terms of commonality, several methodologies have attempted to minimize the inevitable charred OC fraction created during the thermal treatment in order to isolate ‘pure’ BC. However, they inherently differ in the manner in which the charring issue is addressed. This probably is one of the key factors leading to the disagreement in 14C-BC results in inter-comparison studies. While the most common routine for minimizing this effect on the isotope analysis of BC has been the pre-treatment of filters (water extraction) followed by temperature optimization (e.g., AT 2), some methods (e.g., AT 5 and 6) tackle this issue through the introduction of intermediate heating steps in temperature protocols alone. AT 1 on the other hand is the only method which neither includes a pre-treatment step nor uses an intermediate temperature-based optimization for correcting/minimizing the charring effect. We echo the opinion from previous studies (e.g., Zenker et al., 2017) that it is likely that radiocarbon-isotope analysis of BC by this method may lead to an overestimation of the “modern” fraction of BC. A thorough chemical/isotope investigation of the charring-induced discrepancy on the Δ14C-BC composition is warranted for AT 1.
Several corrections are generally applied when reporting the final fm values, which also differ among the various methods (Zhang et al., 2012; Zenker et al., 2017). While background and blank contamination, OC charring corrections are common in most ATs, the “yield correction” is used only by a few. This is much needed for an accurate uncertainty estimation. In general, it is very likely that a 100% recovery of BC is not attainable for all samples when including pre-treatments and charring corrections. As such, the ideal approach should be to incorporate the yield correction—the final fm values are reported corresponding to a 100% recovery based on an extrapolation of the empirically determined relationship between the BC yield (upon isolation) and the measured fm (BC). As an example, a recent study mentioned that BC recovery with the AT 1 for filters from a sampling campaign in S Asia were as low as 65% (Dasari, 2021). Incorporating yield correction on such recoveries could lead to a difference of upto 100% in the reported fm (BC) based on reported empirical correlations (Zhang et al., 2012). Also, there could be the issue of laser pegging on heavily loaded filters that are analysed for 14C-BC (Khan et al., 2012). This largely depends on the filter collection location (Khan et al., 2012). We have not found any ancillary information on the laser attenuation for the reported 14C-BC values from polluted sites (e.g., Andersson et al., 2015; Fang et al., 2018; Bikkina et al., 2019). This information along with BC recoveries ought to be reported in the publications dealing with radiocarbon-based BC studies for improving transparency in the results. We recommend that filter pre-treatment with water extraction step needs to be incorporated in AT 1 to better tackle the possible charring issue.
We also find a geographical bias in the application of the different techniques, for e.g., in the case of East Asia various ATs have been used to conduct the source apportionment of BC (Liu et al., 2017; Fang et al., 2018; Ni et al., 2018) and in general agree that this is a fossil fuel dominated BC regime, however, in the case of South Asia only AT1 has been applied to different sites over a decade (Bosch et al., 2014; Bikkina et al., 2019; Dasari et al., 2020). There is an acute need to cross-validate these estimates as South Asian BC has a high contribution from biomass burning and AT1 is a technique wherein the fm is likely overestimated.
A few other methods have been also developed for 14C-BC analysis such as the CTO-375 method (Zencak et al., 2007) which is thought to harvest only the most refractory part of EC (soot-EC) and is thus associated with much lower EC recoveries than the thermo-optical analysis, and hydropyrolysis method (Ascough et al., 2009; Zhang et al., 2019) which is believed to avoid the charring effect by using high-pressure hydrogen during the pre-treatment phase. However, the latter remains in infancy in terms of application. A comprehensive inter-comparison exercise is much needed to fully deconvolute the influence of the different approaches on the reported results. Prior to that, suitable (EC) reference materials for 14C analysis on aerosols need to be defined. This is because, presently, only ambient filter samples are used in 14C inter-comparisons where the “true” fm is not known (Liu et al., 2017; Zenker et al., 2017; Huang et al., 2021). Therefore, despite similar or different results, the accuracy of all methods still remains impossible to decide.
Author Contributions
Conceptualization, SD; Investigation, SD and DW; Writing—original draft preparation, SD; Writing—review and editing, SD and DW; Visualization, SD All authors have approved the submitted version of the manuscript.
Conflict of Interest
The authors declare that the research was conducted in the absence of any commercial or financial relationships that could be construed as a potential conflict of interest.
Publisher’s Note
All claims expressed in this article are solely those of the authors and do not necessarily represent those of their affiliated organizations, or those of the publisher, the editors and the reviewers. Any product that may be evaluated in this article, or claim that may be made by its manufacturer, is not guaranteed or endorsed by the publisher.
References
Anderson, E. C., Arnold, J. R., and Libby, W. F. (1951). Measurement of Low Level Radiocarbon. Rev. Sci. Instrum. 22, 225–230. doi:10.1063/1.1745896
Andersson, A., Deng, J., Du, D., Zheng, M., Yan, C., Sköld, M., et al. (2015). Regionally-Varying Combustion Sources of the January 2013 Severe Haze Events over Eastern China. Environ. Sci. Technol. 49, 2038–2043. doi:10.1021/es503855e
Arnold, J. R., and Libby, W. F. (1949). Age Determinations by Radiocarbon Content: Checks with Samples of Known Age. Science 110, 678–680. doi:10.1126/science.110.2869.678
Ascough, P., Bird, M., Brock, F., Higham, T. F. G., Meredith, W., Snape, C. E., et al. (2009). Hydropyrolysis as a New Tool for Radiocarbon Pre-Treatment and the Quantification of Black Carbon. Quat. Geochronol. 4, 140–147. doi:10.1016/j.quageo.2008.11.001
Bernardoni, V., Calzolai, G., Chiari, M., Fedi, M., Lucarelli, F., Nava, S., et al. (2013). Radiocarbon Analysis on Organic and Elemental Carbon in Aerosol Samples and Source Apportionment at an Urban Site in Northern Italy. J. Aerosol Sci. 56, 88–99. doi:10.1016/j.jaerosci.2012.06.001
Bikkina, S., Andersson, A., Kirillova, E. N., Holmstrand, H., Tiwari, S., Srivastava, A. K., et al. (2019). Air Quality in Megacity Delhi Affected by Countryside Biomass Burning. Nat. Sustain. 2, 200–205. doi:10.1038/s41893-019-0219-0
Birch, M. E., and Cary, R. A. (1996). Elemental Carbon-Based Method for Monitoring Occupational Exposures to Particulate Diesel Exhaust. Aeros. Sci. Techn. 25, 221–241. doi:10.1080/02786829608965393
Bond, T. C., Doherty, S. J., Fahey, D. W., Forster, P. M., Berntsen, T., DeAngelo, B. J., et al. (2013). Bounding the Role of Black Carbon in the Climate System: A Scientific Assessment. J. Geophys. Res. 118, 5380–5552. doi:10.1002/jgrd.50171
Bosch, C., Andersson, A., Kirillova, E. N., Budhavant, K., Tiwari, S., Praveen, P. S., et al. (2014). Source-Diagnostic Dual-Isotope Composition and Optical Properties of Water-Soluble Organic Carbon and Elemental Carbon in the South Asian Outflow Intercepted over the Indian Ocean. J. Geophys. Res. Atmos. 119, 74. doi:10.1002/2014JD022127
Briggs, N. L., and Long, C. M. (2016). Critical Review of Black Carbon and Elemental Carbon Source Apportionment in Europe and the United States. Atmos. Environ. 144, 409–427. doi:10.1016/j.atmosenv.2016.09.002
Bronk Ramsey, C. B. (2008). Radiocarbon Dating: Revolutions in Understanding. Archaeometry 50, 249–275. doi:10.1111/j.1475-4754.2008.00394.x
Cavalli, F., Viana, M., Yttri, K. E., Genberg, J., and Putaud, J. P. (2010). Toward a Standardised Thermal-Optical Protocol for Measuring Atmospheric Organic and Elemental Carbon: The EUSAAR Protocol. Atmos. Meas. Tech. 3, 79–89. doi:10.5194/amt-3-79-2010
Dasari, S., Andersson, A., Stohl, A., Evangeliou, N., Bikkina, S., Holmstrand, H., et al. (2020). Source Quantification of South Asian Black Carbon Aerosols with Isotopes and Modeling. Environ. Sci. Technol. 54, 11771–11779. doi:10.1021/acs.est.0c02193
Dasari, S. (2021). Isotope-Based Constraints on Sources and Processing of Black Carbon, Carbon Monoxide, and Brown Carbon in South Asia. Doctoral dissertation. Department of Environmental Science, Stockholm University. ISBN: 978-91-7911-443-5
Dusek, U., Monaco, M., Prokopiou, M., Gongriep, F., Hitzenberger, R., Meijer, H. A. J., et al. (2014). Evaluation of a Two-Step Thermal Method for Separating Organic and Elemental Carbon for Radiocarbon Analysis. Atmos. Meas. Tech. 7, 1943–1955. doi:10.5194/amt-7-1943-2014
Dusek, U., ten Brink, H. M., Meijer, H. A. J., Kos, G., Mrozek, D., Röckmann, T., et al. (2013). The Contribution of Fossil Sources to the Organic Aerosol in The Netherlands. Atmos. Environ. 74, 169–176. doi:10.1016/j.atmosenv.2013.03.015
Elmquist, M., Cornelissen, G., Kukulska, Z., and Gustafsson, Ö. (2006). Distinct Oxidative Stabilities of Char versus Soot Black Carbon: Implications for Quantification and Environmental Recalcitrance. Glob. Biogeochem. Cycles. 20, GB2009. doi:10.1029/2005GB002629
Fahrni, S. M., Wacker, L., Synal, H. A., and Szidat, S. (2013). Improving a Gas Ion Source for 14C AMS. Nucl. Instrum. Methods Phys. Res. Sect. B Beam Interact. Mater. Atoms 294, 320–327. doi:10.1016/j.nimb.2012.03.037
Fang, W., Du, K., Andersson, A., Xing, Z., Cho, C., Kim, S. W., et al. (2018). Dual-Isotope Constraints on Seasonally Resolved Source Fingerprinting of Black Carbon Aerosols in Sites of the Four Emission Hot Spot Regions of China. J. Geophys. Res. Atmos. 123, 11,735–11,747. doi:10.1029/2018JD028607
Fifield, L. K. (1999). Accelerator Mass Spectrometry and its Applications. Rep. Prog. Phys. 62, 1223–1274. doi:10.1088/0034-4885/62/8/202
Fushimi, A., Wagai, R., Uchida, M., Hasegawa, S., Takahashi, K., Kondo, M., et al. (2011). Radiocarbon (14C) Diurnal Variations in Fine Particles at Sites Downwind from Tokyo, Japan in Summer. Environ. Sci. Technol. 45, 6784–6792. doi:10.1021/es201400p
Graven, H. D. (2015). Impact of Fossil Fuel Emissions on Atmospheric Radiocarbon and Various Applications of Radiocarbon over This Century. Proc. Natl. Acad. Sci. U. S. A. 112, 9542–9545. doi:10.1073/pnas.1504467112
Gustafsson, Ö., Kruså, M., Zencak, Z., Sheesley, R. J., Granat, L., Engström, E., et al. (2009). Brown Clouds over South Asia: Biomass or Fossil Fuel Combustion? Science 323, 495–498. doi:10.1126/science.1164857
Heal, M. R., Naysmith, P., Cook, G. T., Xu, S., Duran, T. R., and Harrison, R. M. (2011). Application of 14C Analyses to Source Apportionment of Carbonaceous PM2.5 in the UK. Atmos. Environ. 45, 2341–2348. doi:10.1016/j.atmosenv.2011.02.029
Hellborg, R., and Skog, G. (2008). Accelerator Mass Spectrometry. Mass Spectrom. Rev. 27, 398–427. doi:10.1002/mas.20172
Huang, L., Brook, J. R., Zhang, W., Li, S. M., Graham, L., Ernst, D., et al. (2006). Stable Isotope Measurements of Carbon Fractions (OC/EC) in Airborne Particulate: A New Dimension for Source Characterization and Apportionment. Atmos. Environ. 40, 2690–2705. doi:10.1016/j.atmosenv.2005.11.062
Huang, L., Zhang, W., Santos, G. M., Rodríguez, B. T., Holden, S. R., Vetro, V., et al. (2021). Application of the ECT9 Protocol for Radiocarbon-Based Source Apportionment of Carbonaceous Aerosols. Atmos. Meas. Tech. 14, 3481–3500. doi:10.5194/amt-14-3481-2021
Karanasiou, A., Diapouli, E., Cavalli, F., Eleftheriadis, K., Viana, M., Alastuey, A., et al. (2011). On the Quantification of Atmospheric Carbonate Carbon by Thermal/optical Analysis Protocols. Atmos. Meas. Tech. 4, 2409–2419. doi:10.5194/amt-4-2409-2011
Karanasiou, A., Minguillón, M. C., Viana, M., Alastuey, A., Putaud, J. P., Maenhaut, W., et al. (2015). Thermal-Optical Analysis for the Measurement of Elemental Carbon (EC) and Organic Carbon (OC) in Ambient Air a Literature Review. Atmos. Meas. Tech. Disc. 8, 9649–9712. doi:10.5194/amtd-8-9649-2015
Khan, B., Hays, M. D., Geron, C., and Jetter, J. (2012). Differences in the OC/EC Ratios that Characterize Ambient and Source Aerosols Due to Thermal-Optical Analysis. Aeros. Sci. Tech. 46, 127–137. doi:10.1080/02786826.2011.609194
Lack, D. A., Moosmüller, H., McMeeking, G. R., Chakrabarty, R. K., and Baumgardner, D. (2014). Characterizing Elemental, Equivalent Black, and Refractory Black Carbon Aerosol Particles: A Review of Techniques, Their Limitations and Uncertainties. Analy. Bioanaly. Chem. 406, 99–122. doi:10.1007/s00216-013-7402-3
Lelieveld, J., Evans, J. S., Fnais, M., Giannadaki, D., and Pozzer, A. (2015). The Contribution of Outdoor Air Pollution Sources to Premature Mortality on a Global Scale. Nature 525, 367–371. doi:10.1038/nature15371
Levin, I., and Hesshaimer, V. (2000). Radiocarbon - A Unique Tracer of Global Carbon Cycle Dynamics. Radiocarbon 42, 69–80. Cambridge Univ. Press. doi:10.1017/S0033822200053066
Li, C., Bosch, C., Kang, S., Andersson, A., Chen, P., Zhang, Q., et al. (2016). Sources of Black Carbon to the Himalayan-Tibetan Plateau Glaciers. Nat. Commun. 7, 12574. doi:10.1038/ncomms12574
Liang, C. S., Duan, F. K., He, K. B., and Ma, Y. L. (2016). Review on Recent Progress in Observations, Source Identifications and Countermeasures of PM2.5. Environ. Int. 86, 150–170. doi:10.1016/j.envint.2015.10.016
Libby, W. F. (1946). Atmospheric Helium Three and Radiocarbon from Cosmic Radiation. Phys. Rev. 69, 671–672. doi:10.1103/PhysRev.69.671.2
Liu, J., Li, J., Ding, P., Zhang, Y., Liu, D., Shen, C., et al. (2017). Optimizing Isolation Protocol of Organic Carbon and Elemental Carbon for 14C Analysis Using Fine Particulate Samples. Atmos. Environ. 154, 9–19. doi:10.1016/j.atmosenv.2017.01.027
Moosmüller, H., Chakrabarty, R. K., and Arnott, W. P. (2009). Aerosol Light Absorption and its Measurement: A Review. J. Quant. Spectro. Rad. Trans. 110, 844–878. doi:10.1016/j.jqsrt.2009.02.035
Myhre, G., Shindell, D., Bréon, F. M., Collins, W., Fuglestvedt, J., Huang, J., et al. (2013). “Anthropogenic and Natural Radiative Forcing,” in Climate Change 2013: The Physical Science Basis: Working Group I Contribution to the Fifth Assessment Report of the Intergovernmental Panel on Climate Change. Editors T. F. Stocker, D. Qin, G.-K. Plattner, M. Tignor, S. K. Allen, J. Boschunget al. (Cambridge, United Kingdom and New York, NY, USA: Cambridge University Press), 8, 659–740. doi:10.1017/CBO9781107415324.018
Ni, H., Huang, R. J., Cao, J., Liu, W., Zhang, T., Wang, M., et al. (2018). Source Apportionment of Carbonaceous Aerosols in Xi'an, China: Insights from a Full Year of Measurements of Radiocarbon and the Stable Isotope <sup>13</sup>C. Atmos. Chem. Phys. 18, 16363–16383. doi:10.5194/acp-18-16363-2018
Nydal, R. (1962). Proportional Counting Technique for Radiocarbon Measurements. Rev. Sci. Instrum. 33, 1313–1320. doi:10.1063/1.1717767
Olsson, I. U. (1970). “The Use of Oxalic Acid as a Standard,” in Proceedings of the 12th Nobel Symposium, Institute of Physics at Uppsala University, Sweden, December 12, 1969. Editor I. U. Olsson.
Panteliadis, P., Hafkenscheid, T., Cary, B., Diapouli, E., Fischer, A., Favez, O., et al. (2015). ECOC Comparison Exercise with Identical Thermal Protocols after Temperature Offset Correction - Instrument Diagnostics by In-Depth Evaluation of Operational Parameters. Atmos. Meas. Tech. 8, 779–792. doi:10.5194/amt-8-779-2015
Petzold, A., Ogren, J. A., Fiebig, M., Laj, P., Li, S. M., Baltensperger, U., et al. (2013). Recommendations for Reporting “Black Carbon” Measurements. Atmos. Chem. Phys. 13, 8365–8379. doi:10.5194/acp-13-8365-2013
Pöschl, U. (2005). Atmospheric Aerosols: Composition, Transformation, Climate and Health Effects. Angew. Chem. Int. Ed. 44, 7520–7540. doi:10.1002/anie.200501122
Ramanathan, V., and Carmichael, G. (2008). Global and Regional Climate Changes Due to Black Carbon. Nat. Geosci. 1, 221–227. doi:10.1038/ngeo156
Rana, A., Jia, S., and Sarkar, S. (2019). Black Carbon Aerosol in India: A Comprehensive Review of Current Status and Future Prospects. Atmos. Res. 218, 207–230. doi:10.1016/j.atmosres.2018.12.002
Salazar, G., Zhang, Y. L., Agrios, K., and Szidat, S. (2015). Development of a Method for Fast and Automatic Radiocarbon Measurement of Aerosol Samples by Online Coupling of an Elemental Analyzer with a MICADAS AMS. Nucl. Instrum. Methods Phys. Res. Sect. B Beam Interact. Mat. Atoms. 361, 163–167. doi:10.1016/j.nimb.2015.03.051
Saleh, R., Cheng, Z., and Atwi, K. (2018). The Brown-Black Continuum of Light-Absorbing Combustion Aerosols. Environ. Sci. Technol. Lett. 5, 508–513. doi:10.1021/acs.estlett.8b00305
Saleh, R. (2020). From Measurements to Models: toward Accurate Representation of Brown Carbon in Climate Calculations. Curr. Pollut. Rep. 6, 90–104. doi:10.1007/s40726-020-00139-3
Sillanpää, M., Frey, A., Hillamo, R., Pennanen, A. S., and Salonen, R. O. (2005). Organic, Elemental and Inorganic Carbon in Particulate Matter of Six Urban Environments in Europe. Atmos. Chem. Phys. 5, 2869–2879. doi:10.5194/acp-5-2869-2005
Stuiver, M., and Polach, H. A. (1977). Discussion Reporting of 14C Data. Radiocarbon 19, 355–363. doi:10.1017/S0033822200003672
Stuiver, M. (1980). Workshop on 14C Data Reporting. Radiocarbon 22, 964–966. doi:10.1017/S0033822200010389
Subramanian †, R., Khlystov, A. Y., and Robinson, A. L. (2006). Effect of Peak Inert-Mode Temperature on Elemental Carbon Measured Using Thermal-Optical Analysis. Aerosol Sci. Technol. 40, 763–780. doi:10.1080/02786820600714403
Synal, H. A., Stocker, M., and Suter, M. (2007). MICADAS: A New Compact Radiocarbon AMS System. Nucl. Instrum. Methods Phys. Res. Sect. B Beam Interact. Mater. Atoms 259, 7–13. doi:10.1016/j.nimb.2007.01.138
Szidat, S., Bench, G., Bernardoni, V., Calzolai, G., Czimczik, C. I., Derendorp, L., et al. (2013). Intercomparison of 14C Analysis of Carbonaceous Aerosols: Exercise 2009. Radiocarbon 55, 1496–1509. doi:10.1017/S0033822200048426
Szidat, S., Jenk, T. M., Gäggeler, H. W., Synal, H. A., Hajdas, I., Bonani, G., et al. (2004). THEODORE, a Two-Step Heating System for the EC/OC Determination of Radiocarbon (14C) in the Environment. Nucl. Instrum. Methods Phys. Res. Sect. B Beam Interact. Mater. Atoms 223-224, 829–836. doi:10.1016/j.nimb.2004.04.153
Szidat, S., Jenk, T. M., Synal, H. A., Kalberer, M., Wacker, L., Hajdas, I., et al. (2006). Contributions of Fossil Fuel, Biomass-Burning, and Biogenic Emissions to Carbonaceous Aerosols in Zurich as Traced by14C. J. Geophys. Res. 111, D07206. doi:10.1029/2005JD006590
Turnbull, J. C., Graven, H., and Krakauer, N. Y. (2016). “Radiocarbon in the Atmosphere,” in Radiocarbon and Climate Change (Cham: Springer), 83–137. doi:10.1007/978-3-319-25643-6_4
Wacker, L., Fahrni, S. M., Hajdas, I., Molnar, M., Synal, H. A., Szidat, S., et al. (2013). A Versatile Gas Interface for Routine Radiocarbon Analysis with a Gas Ion Source. Nucl. Instrum. Methods Phys. Res. Sect. B Beam Interact. Mater. Atoms 294, 315–319. doi:10.1016/J.NIMB.2012.02.009
Winiger, P., Andersson, A., Eckhardt, S., Stohl, A., and Gustafsson, Ö. (2016). The Sources of Atmospheric Black Carbon at a European Gateway to the Arctic. Nat. Commun. 7, 12776. doi:10.1038/ncomms12776
World Health Organization (2021). Review of Evidence on Health Aspects of Air Pollution: REVIHAAP Project: Technical Report (No. WHO/EURO: 2013-2663-42419-58845). Copenhagen, Denmark: World Health Organization. Regional Office for Europe.
Yu, J. Z., Xu, J., and Yang, H. (2002). Charring Characteristics of Atmospheric Organic Particulate Matter in Thermal Analysis. Environ. Sci. Technol. 36, 754–761. doi:10.1021/es015540q
Zencak, Z., Elmquist, M., and Gustafsson, Ö. (2007). Quantification and Radiocarbon Source Apportionment of Black Carbon in Atmospheric Aerosols Using the CTO-375 Method. Atmos. Environ. 41, 7895–7906. doi:10.1016/j.atmosenv.2007.06.006
Zenker, K., Vonwiller, M., Szidat, S., Calzolai, G., Giannoni, M., Bernardoni, V., et al. (2017). Evaluation and Inter-Comparison of Oxygen-Based OC-EC Separation Methods for Radiocarbon Analysis of Ambient Aerosol Particle Samples. Atmosphere 8, 226. doi:10.3390/atmos8110226
Zhang, G., Liu, J., Li, J., Li, P., Wei, N., and Xu, B. (2021). Radiocarbon Isotope Technique as a Powerful Tool in Tracking Anthropogenic Emissions of Carbonaceous Air Pollutants and Greenhouse Gases: A Review. Fundam. Res. 1, 306–316. doi:10.1016/j.fmre.2021.03.007
Zhang, X., Li, J., Mo, Y., Shen, C., Ding, P., Wang, N., et al. (2019). Isolation and Radiocarbon Analysis of Elemental Carbon in Atmospheric Aerosols Using Hydropyrolysis. Atmos. Environ. 198, 381–386. doi:10.1016/j.atmosenv.2018.11.005
Zhang, Y. L., Perron, N., Ciobanu, V. G., Zotter, P., Minguillón, M. C., Wacker, L., et al. (2012). On the Isolation of OC and EC and the Optimal Strategy of Radiocarbon-Based Source Apportionment of Carbonaceous Aerosols. Atmos. Chem. Phys. 12, 10841–10856. doi:10.5194/acp-12-10841-2012
Zhao, Y., Nielsen, C. P., Lei, Y., McElroy, M. B., and Hao, J. (2011). Quantifying the Uncertainties of a Bottom-Up Emission Inventory of Anthropogenic Atmospheric Pollutants in China. Atmos. Chem. Phys. 11, 2295–2308. doi:10.5194/acp-11-2295-2011
Keywords: air pollution, incomplete combustion, elemental carbon, charring effect, thermo-optical analysis
Citation: Dasari S and Widory D (2022) Radiocarbon (14C) Analysis of Carbonaceous Aerosols: Revisiting the Existing Analytical Techniques for Isolation of Black Carbon. Front. Environ. Sci. 10:907467. doi: 10.3389/fenvs.2022.907467
Received: 29 March 2022; Accepted: 16 May 2022;
Published: 13 July 2022.
Edited by:
Laodong Guo, University of Wisconsin–Milwaukee, United StatesReviewed by:
Junwen Liu, Jinan University, ChinaCopyright © 2022 Dasari and Widory. This is an open-access article distributed under the terms of the Creative Commons Attribution License (CC BY). The use, distribution or reproduction in other forums is permitted, provided the original author(s) and the copyright owner(s) are credited and that the original publication in this journal is cited, in accordance with accepted academic practice. No use, distribution or reproduction is permitted which does not comply with these terms.
*Correspondence: Sanjeev Dasari, c2FuamVldi5kYXNhcmlAdW5pdi1ncmVub2JsZS1hbHBlcy5mcg==