- 1Ecohydrology Research Group, University of Waterloo, Waterloo, ON, Canada
- 2Department of Earth and Environmental Sciences, University of Waterloo, Waterloo, ON, Canada
- 3Department of Biology, University of Waterloo, Waterloo, ON, Canada
- 4Water Institute, University of Waterloo, Waterloo, ON, Canada
Cold regions are warming faster than the rest of the planet, with the greatest warming occurring during the winter and shoulder seasons. Warmer winters are further predicted to result in more frequent soil freezing and thawing events. Freeze-thaw cycles affect biogeochemical soil processes and alter carbon and nutrient export from soils, hence impacting receiving ground and surface waters. Cold region agricultural management should therefore consider the possible effects on water quality of changing soil freeze-thaw dynamics under future climate conditions. In this study, soil column experiments were conducted to assess the leaching of fertilizer nitrogen (N) from an agricultural soil during the non-growing season. Identical time series temperature and precipitation were imposed to four parallel soil columns, two of which had received fertilizer amendments, the two others not. A 15-30-15 N-P-K fertilizer (5.8% ammonium and 9.2% urea) was used for fertilizer amendments. Leachates from the soil columns were collected and analyzed for major cations and anions. The results show that thawing following freezing caused significant export of chloride (Cl−), sulfate (SO42−) and nitrate (NO3−) from the fertilizer-amended soils. Simple plug flow reactor model calculations indicated that the high NO3− concentrations produced during the fertilized soil thawing events were due to nitrification of fertilizer N in the upper oxidized portion of the soil. The very low concentrations of NO3− and ammonium in the non-fertilized soils leachates implied that the freeze-thaw cycles had little impact on the mineralization of soil organic N. The findings, while preliminary, indicate that unwanted N enrichment of aquifers and rivers in agricultural areas caused by fall application of N fertilizers may be exacerbated by changing freeze-thaw activity.
1 Introduction
Soils are a key component of global carbon and nutrient cycles, constituting the largest terrestrial carbon reservoir and serving as an interface between the atmosphere, biosphere, hydrosphere, and lithosphere (Zhou et al., 2019). Soil hydro-biogeochemical properties and processes control water filtration, flow regulation, erosion resistance, contaminant immobilization, and crop cultivation, as well as soil greenhouse gas (GHG) emissions and primary production (Knapen et al., 2007; Keesstra et al., 2012; Smith et al., 2015). However, these soil functions are dependent on climate and land use. In agroecosystems, climate warming is expected to alter global soil biogeochemical cycling, affecting carbon and nutrient transformation and retention capacity, and intense agricultural land use has caused declines in soil organic carbon while associated fertilizer application has resulted in increased soil GHG emissions, nutrient runoff and loss (Post and Kwon, 2000; Halvorson et al., 2014). Agricultural systems are also critical source areas for nutrient pollutants (e.g., nitrogen (N) and phosphorus (P)) due to fertilizer use and have been the target of numerous management strategies (Sharpley et al., 2003). For instance, no-till conservation techniques have been increasingly employed to mitigate nutrient loss due to erosion, but nutrient export via surface runoff, subsurface leaching, and volatilization allow for continued high nutrient export (King et al., 2017; Beach et al., 2018).
In cold climate regions, as seasonal snow cover periods are shortened due to winter climate warming, soils are becoming more susceptible to freezing during the non-growing season (i.e., NGS: fall, winter, and spring) (Zhang, 2005; Hayashi et al., 2013; Zhang and Ma, 2018; Natali et al., 2019). During the shoulder NGS, diurnal temperature fluctuations will likely change the occurrence of freeze-thaw cycles (FTCs) in soils lacking an insulating snowpack (Campbell et al., 2014; Watanabe et al., 2019). FTCs impact soil physical (e.g. decrease in bulk density and soil aggregate disruption), hydrological and chemical properties (e.g., decrease soil organic matter and nitrogen availability) and microbial populations (e.g., biomass decline and compositional shifts) (Henry, 2007). These changes in soil characteristics have been observed in many laboratory and field studies and been linked to changes in carbon and nutrient dynamics in FTC-affected soils (Matzner and Borken, 2008; Campbell et al., 2010; Song et al., 2017). Winter soil carbon and nutrient dynamics in colder regions have a significant impact on annual carbon and nutrient budgets (Mikan et al., 2002; Monson et al., 2006; Matzner and Borken, 2008). A number of studies have found that microbial processes may persist at subzero temperatures, which has implications for biogeochemical activities and nutrient stocks and species available for export during thaw periods (Elberling and Brandt, 2003; Panikov et al., 2006; Groffman et al., 2009; Wipf et al., 2015). In particular, nitrogen fertilizers have been demonstrated to be susceptible to nitrification at soil temperatures below freezing (Clark et al., 2009; Chantigny et al., 2019). This is of concern as tile drainage systems are prone to nitrate (NO3−) loss during the NGS due to excessive fertilizer application, nitrification, and lack of crop NO3− uptake, while controlled drainage systems can mitigate NO3− losses but may increase N2O gas emissions via denitrification (Kliewer and Gilliam, 1995; Lalonde et al., 1996; Saadat et al., 2018). As a consequence, agricultural nitrogen pools pose a water and atmospheric contamination threat (Almasri and Kaluarachchi, 2004; Mahvi et al., 2005).
In agroecosystems, NGS studies examining nutrient loss have typically focused on how FTCs affect natural nutrient pools, but few have centered on how they impact fertilizer loss from agricultural soils. Our growing understanding of the NGS physical and biological processes require that fertilizer application practices (fertilizer source, rate, time, and place) in cold climate regions be reexamined to minimize nutrient loss during this dynamic period. For example, fall fertilizer application (NGS) is a common agricultural practice performed out of convenience and under the assumption that the applied fertilizer will remain and be available to crops come the spring (Gentry et al., 2014; Romero et al., 2017). However, these nutrients are susceptible to overwinter losses, especially in cold climate regions (Ryan et al., 2000; Chantigny et al., 2019). Current nutrient management strategies like the 4R fertilization guidelines seek to provide a holistic approach to nutrient management, accounting for economic, environmental, and social outcomes of fertilizer use (Johnston and Bruulsema, 2014). The 4R nutrient stewardship guidelines (right source at the right rate, right time, and right place) acknowledge these overwinter losses, but climate change-driven changes to the severity and incidence rates of winter soil processes such as freeze-thaw cycling remain a prominent knowledge gap. Therefore, studies on winter processes targeting fertilizer retention in agricultural soil can inform nutrient management strategies, such as the 4R fertilizer guidelines, to account for changing conditions in cold region agroecosystems.
In this study, a soil column experiment was conducted using agricultural soil to explore the effects of freeze-thaw cycling on fertilizer loss under variable winter conditions. This was achieved by exposing soil columns to a winter and shoulder season air temperature and precipitation models, based on southern Ontario climate data, to assess the leaching of nutrients from fertilized agricultural soil during the NGS. A pair of unfertilized control columns were also packed with the same soil and exposed to the same temperature conditions to determine the background nutrient leaching for comparison. A microbial study using this column system was conducted concurrently as detailed in Jensen et al. (In review). The goal of our experiment was to better delineate the effect FTCs have on nutrient exports in agricultural soils by comparing nutrient leaching under the fertilized and unfertilized conditions. While our experiment did not include a non-FTC condition, several leachate samples were collected during the early portion of the experiment prior to the onset of freezing and thawing cycles that were representative of leaching under non-FTC conditions. Our hypothesis was that the simulated FTCs would greatly enhance and alter nutrient leaching from fertilized agricultural soils and slightly enhance and alter nutrient leaching from unfertilized agricultural soils.
2 Materials and Methods
2.1 Soil Collection and Properties
The soil sample used for the experiment was collected from an agricultural field at the rare Charitable Reserve in Cambridge, Canada (43°22′39.80″N; 80°22′07.28″W) in mid-October. This field had been cultivated in previous years but had not been cultivated for 2 years prior to sample collection. The soil was collected from the surface horizons (0–18 cm). The particle size distributions of the soil sample were determined using the pipette method (Gee and Bauder, 1986) and the analysis showed 32%, 52% and 16% of sand, silt and clay contents, respectively, with textures ranging from silt loam to loam and a pH of 7.2 (Table 1). The soil sample was analyzed with a CHNS Carbo Erba analyzer (method detection limit, MDL: 0.1 mg g−1) to determine soil chemistry. Total porosity and bulk density of soil sample were determined gravimetrically from the saturated mass, the oven-dried (at 105°C for 24 h) mass and the original volume of the sample, following the method of Boelter (1972) and the bulk density and porosity were calculated to be 1.22 g cm−3 and 0.54, respectively.
2.2 Experimental Freeze-Thaw Soil Column System
The entire experimental freeze-thaw soil column system consists of four columns (a schematic diagram of the column set-up is shown in Figure 1). The collected soil sample was sieved between 1 and 2 mm and homogenized for reproducibility. The soil was then packed into four hard acrylic columns (inner diameter: 7.5 cm, length: 60 cm; Soil Measurement Systems, LLC, United States) with a 50 cm soil depth. Homogenizing and packing the soil in this manner disrupted the existing soil structure, likely removing the preferential flow pathways that may have existed in the undisturbed sample. Each column was packed with a similar mass of soil (∼2.5 kg) and the columns were placed in an environmental chamber incubator (Percival I-41NL XC9) whose air temperature was controlled. The soil columns were initially saturated from the bottom with Milli-Q water and stored at 10°C for 5 days prior to experiment initiation. The soil column system was designed to simulate freeze-thaw cycles with realistic soil subsurface temperature gradients. A 150-W band-heater (120V, 2 W/inch2, custom-made by Gordo Sales Inc.) was placed surrounding the lower 40 cm of each column that maintained the temperature at 8°C in the lower portion of the soil, representative of southern Ontario’s subsurface soil and groundwater temperatures (Funk et al., 1980; Conant, 2004; Zhang et al., 2005), to simulate a realistic vertical soil subsurface temperature gradient. This allowed for the top 10 cm of soil above the band-heater to be exposed to fluctuating chamber air temperatures. As the air temperature drops below 0°C, freezing occurs downwards from the surface of the soil. As the air temperature returns above 0°C, the upper soil layer thaws. By pre-programming the air temperature cycle in the chamber, freeze-thaw cycles were induced in the soil columns (Section 2.5). The top and bottom of the columns were closed with the acrylic end-caps sealed tightly by the O-rings inside of the end-caps. A filter membrane (Soil Measurement Systems, LLC, United States, bubbling pressure: 600 mbar) closed off the bottom of each column with a nylon mesh (Soil Measurement Systems, LLC, United States, bubbling pressure: 32 mbar) the top of filter membrane. For each column, three steel rods connected the acrylic top and bottom end-caps and were secured with bolts. The headspace of the soil columns was flushed with water-saturated air to minimize evaporative losses through the upper part of the columns. Time-series temperature data was recorded every 15 min during the experiment by six temperature sensors (PT-100, DaqLink Fourier Systems Ltd., #DBSA720) installed in one of the soil columns at depths of +2.5, −3.5, −7, −15.5, and −33 cm relative to the soil surface, with one sensor in the chamber to monitor air temperature.
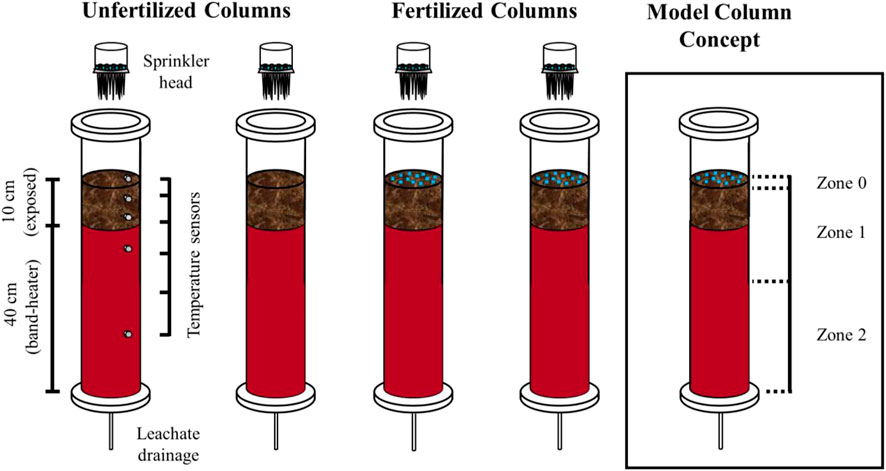
FIGURE 1. Schematic showing the two control columns (unfertilized) and two experimental columns (fertilized). Temperature sensors were installed at +2.5, −3.5, −7, −15.5, and −33 cm relative to the soil surface in one of the control columns. The column on the right is showing the concept used for the simple plug flow reactor model with three zones (Section 2.6).
2.3 Environmental Chamber Temperature and Precipitation Regimes
The soil columns were exposed to a 55-day NGS air temperature and precipitation weather sequence (Figure 2), representative of winter and shoulder seasonal climate in southern Ontario (CAPMoN, 2018). Daily air temperatures (incubator temperature) fluctuated by about 8–10°C with daily low temperatures occurring between 0:00 and 12:00 and daily high temperatures occurring between 12:00 and 24:00. The incubator took approximately 2 h to warm or cool following a temperature transition. During the coldest period of the NGS weather sequence, a 1-day and 3-day thaw (occurring around Day 30 and 32 of the experiment, respectively) were included to examine the effects of a simulated mid-winter thaw (see Figure 2). A precipitation model was simulated by adding 55–90 ml of artificial rainwater (2–3 times per week, see Figure 2) to the top of each soil column via a sprinkler. Rainwater additions were calculated in mm according to the dimensions of the soil column using cumulative monthly climate data and evenly distributed throughout a condensed NGS weather sequence.
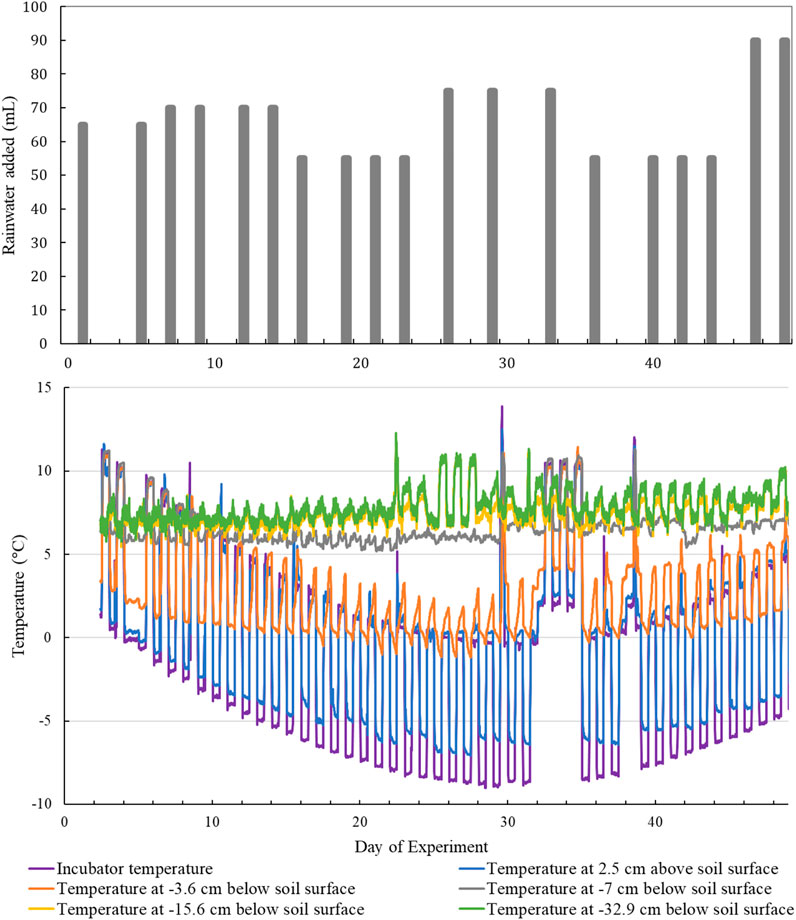
FIGURE 2. The top graph shows total applied volume of artificial rainwater over the course of the experiment. The bottom graph shows air temperature and soil temperature at various depths over the course of the experiment. Soil temperatures were measured at different depths below the soil surface in one of the columns and treated as representative for all columns. The 1-day thaw occurs around Day 30 and the 3-day thaw occurs around Day 32.
2.4 Fertilizer Amendments and Artificial Rainwater
The 15-30-15 NPK Miracle-Gro was used as fertilizer amendments for the experiment. This fertilizer was selected for its solubility to leach through the experimental columns. Approximately 1 g of the fertilizer was ground up for each column and evenly distributed on top of the duplicate fertilized experimental soil columns (4,835 lbs hectare−1; about 25-fold the field applied rate) while the duplicate control columns were left unfertilized (Figure 1). The unfertilized and fertilized treatments were only run in duplicate due to limited access to environmental chamber space and soil column setups. According to the NPK fertilizer label, an estimated 150 mg of elemental nitrogen was then applied to each fertilized column. Artificial rainwater was prepared with a chemical compositions containing Cl− (0.25 mg L−1), SO42− (2.2 mg L−1), NO3− (2.6 mg L−1), Na+ (0.10 mg L−1), Ca2+ (0.48 mg L−1), Mg2+ (0.08 mg L−1), and K+ (0.05 mg L−1), representative of southern Ontario’s rainwater compositions (“Major Ions” 2018). A solution consisting of the mass of fertilizer applied to each column and a volume of Milli-Q water equivalent to the volume of total applied rainwater to each column (1.435 L) was prepared and the concentrations of selected anions were analyzed using ion chromatography (Section 2.6) and with concentrations of 10.7, 0.2, and 35 mg L−1 for Cl−, SO42−, and PO43−, respectively, while concentrations of NO2− and NO3− were below the detection limits. Note that nitrogen in this fertilizer is primarily derived from ammonium chloride (NH4Cl) and urea (CH₄N₂O).
2.5 Leachate Sampling and Analytical Methods
Leachate pore water samples were collected from the bottoms of the four columns when there was sufficient volume for chemical analysis, usually 1 day following rainwater applications. Leachate samples were collected into 100 ml plastic vials and 1 ml of the samples were immediately used for pH and electrical conductivity (EC) analysis using handheld meters (LAQUAtwin meters, model Horiba B-213). One ml of leachate samples was filtered through a 0.2 μm membrane filter (Thermo Scientific Polysulfone filter) and was analyzed for major anions including Cl−, NO3−, PO43−, and SO42− using ion chromatography (IC, Dionex ICS-5000 with a capillary IonPac® AS18 column; ± 3.0% error and ±1.6% precision).
2.6 Model Description
For the modeling of the nitrogen transformation in the fertilized soil columns, a simple plug flow reactor model was used with chemical reactions in a continuous, connected, and flowing system of cylindrical geometries (shown as 3 separate zones in Figure 1) (Ehrl et al., 2018; Gharasoo et al., 2019). In this model simulation, urea is considered to be placed on the top few centimeters of the column (Zone 0) where it is hydrolysed to an unstable carbamic acid that quickly breaks down to carbon dioxide (CO2) and ammonia gas (NH3) and the latter reacts with water to form NH4+. This chain of reactions occurs only at Zone 0 and is described by the following reaction:
where
where
where
where
where
Parameter values were determined from the soil column measurements or obtained from the literature as listed in Table 2. The model run lasted 60 days and the fitting procedure was performed by ReKinSim (Gharasoo et al., 2017).
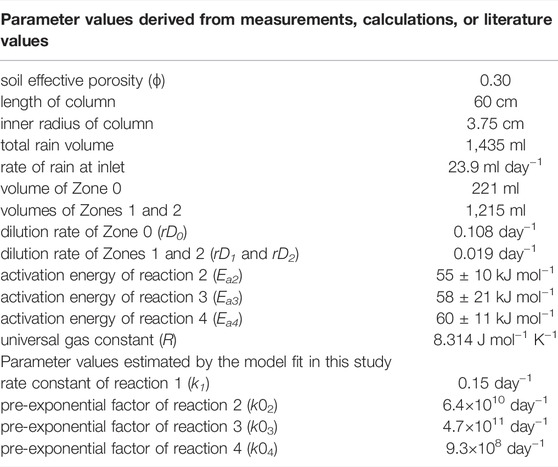
TABLE 2. List of parameters values used for model simulation and estimated parameters from the optimization of Eq. 5 to the measured data. Reaction energies for the reactions 2, 3, and 4 were obtained from McKenney et al., 1984; Ambus, 1993; Saad and Conrad, 1993; and Maag et al., 1997.
For the modeling of Cl− and SO42 dissolution, the dissolution rates were assumed to follow a zero-order term where the actual rates at each time step are estimated by the model. Since Cl− and SO42- are treated as non-reactive in this model, the zones from the N modeling were removed and a column dilution rate of
where kCl and kSO4 are the dissolution rates of Cl− and SO42−, and fRCl and fRSO4 are the retardation factors estimated by the model.
3 Results
3.1 Temperature and Moisture Regimes in the Soil Columns
The environmental chamber air temperature regularly fluctuated above and below zero during the beginning and end of the experiment (Figure 2). Air temperatures remained largely below 0 °C during the middle of the experiment, except during the simulated midwinter thaws. The near surface temperature sensor (−3.6 cm below soil surface) recorded temperatures regularly fluctuating above and below 0°C between Days 16–29, but otherwise all recorded temperatures were above 0°C. Freeze-thaw cycles induced in the near surface soil were the result of realistic diurnal fluctuations in the subsurface temperature (∼3°C) (Decker et al., 2003). The near surface soil temperatures during the simulated midwinter thaws, following the coldest portion of the experiment, were comparable to temperatures recorded at the beginning and end of the experiment. Soil temperatures at depth below −7 cm remained relatively stable throughout the experiment as deeper sections of the soil columns were buffered by the band-heater warming. Column moisture contents did not vary much by depth over the course of the experiment. The average water filled pore space was 50.1% with a SD of ±7.2.
3.2 Soil Column Leachates
The pH values varied from 6.5 to 8.5 in unfertilized column leachates and from 7 to 8.5 in fertilized column leachates. The EC varied from 0.1 to 170 and 200 μS/cm in unfertilized and fertilized columns, respectively. PO43− was not detected in either the unfertilized or fertilized column leachates. Cl− concentrations in the unfertilized column leachates remained low (<5 mg L−1) throughout the duration of the experiment (Figure 3). For the fertilized columns, leachate Cl− concentrations were initially low and started to increase from around Day 15. Residence time was calculated to be ∼13.9 days (ɸ = 0.30; average WFPS
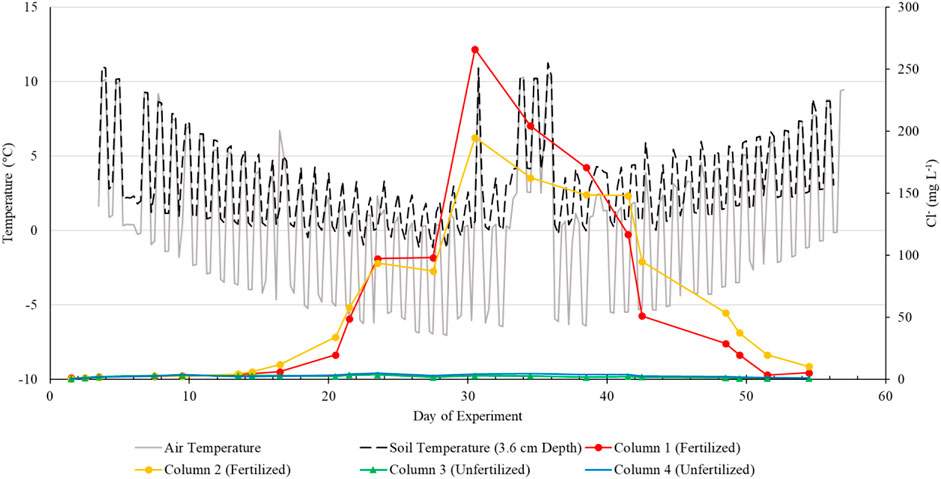
FIGURE 3. Temperature and leachate Cl− concentrations versus time for fertilized and unfertilized columns.
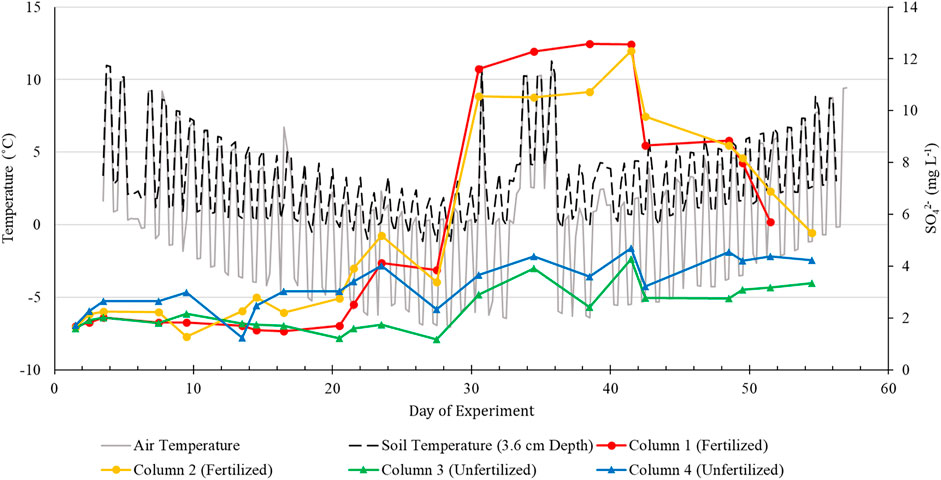
FIGURE 4. Temperature and leachate SO42- concentrations versus time for fertilized and unfertilized columns.
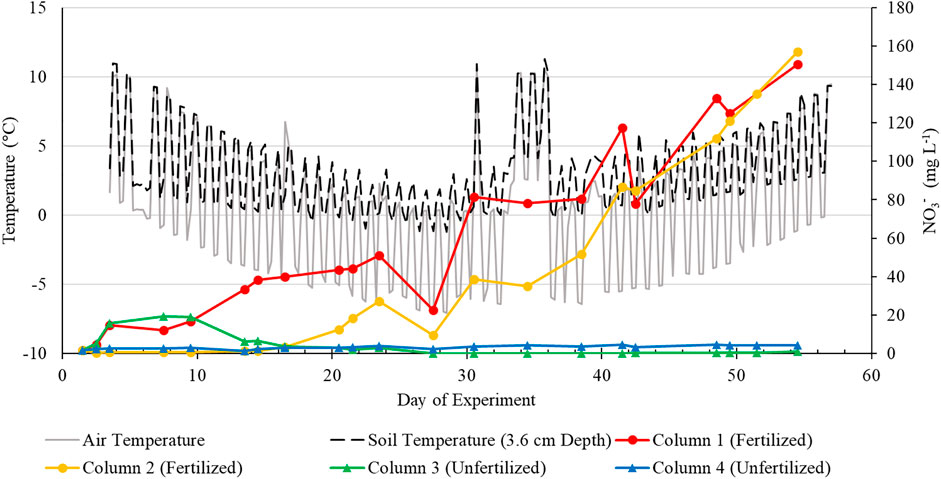
FIGURE 5. Temperature and leachate NO3− concentrations versus time for fertilized and unfertilized columns.

TABLE 3. Mass balance of total nitrogen in two fertilized columns. Extrapolated nitrogen loss is estimated assuming experiment timeline only captured half of NO3− losses.
3.3 Modeling Results
3.3.1 Modeling Chloride and Sulfate Transport
Cl− and SO42− containing compounds in the fertilizer were assumed to quickly dissolve and the resulting concentration profiles are maintained following dissolution. The fate of Cl− seems to be controlled by transport and not affected much by dissolution mechanics or biological processing. As SO42− is treated as non-reactive in our model, it behaves in a similar manner to Cl−. As such, Cl− and SO42− transport through the column is a lot quicker than the nitrogen compounds (i.e., having a shorter resident time). Therefore, the Cl− and SO42− dilution rates were calibrated with a retardation and acceleration factor that is estimated by the model along with the dissolution rates. Experimental results suggest that the dissolution rates are very high at the beginning and then fall dramatically.
The model fit of the Cl− and SO42− closely match the averaged experimental leachate concentrations in the fertilized column (Figure 6). These model results support that the measured Cl− and SO42− leachate concentrations represent the breakthrough of a concentrated front. The results from the fit of model to the experimental breakthrough curves of Cl− and SO42− indicate that dissolution rates are exceptionally high at the beginning of the experiment and the applied fertilizer is quickly consumed. To verify the quick dissolution of Cl− and SO42− from the applied fertilizer, a dissolution batch test was conducted. Results from this test suggested that the fertilizer components containing Cl− and SO42− quickly dissolve, confirming the model results that indicate fast dissolution rates for fertilizer components containing Cl− and SO42−. The retardation factors fRCl and fRSO4 are estimated to be −0.9167 by the model. The negative value indicates that the transport of Cl− and SO42− is much faster than nitrogen species at a reference retardation factor of zero.
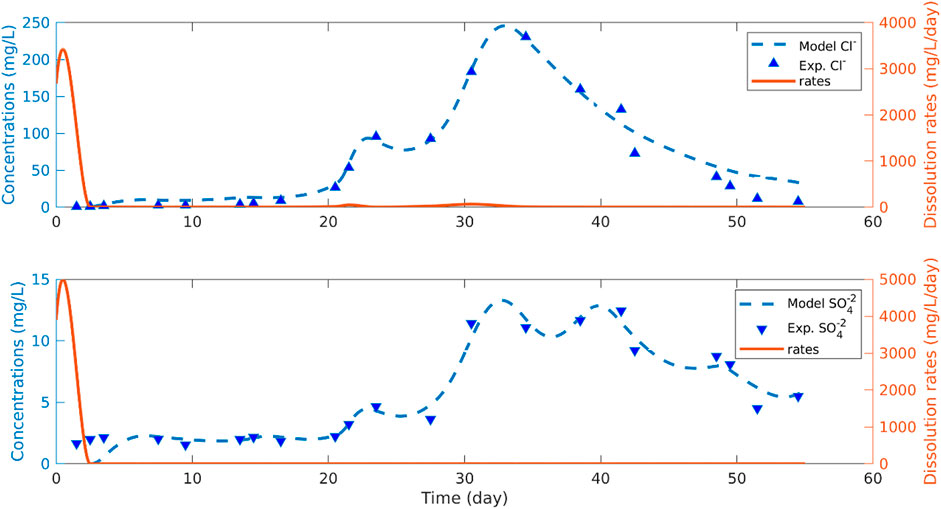
FIGURE 6. Model fit of Cl− and SO42- to the measured leachate concentrations in the fertilized soil columns. The experimental Cl− and SO42- data are averaged from the fertilized columns.
3.3.2 Modeling Nitrogen Transformations
The calculated rate constants of k1, k2, k3, and k4 for the reactions 1 to 4 were as
The model results for NO3− concentrations in Zone 2 show a strong fit to the average measured NO3− in the fertilized soil column leachates (R2 = 0.88) (Figure 7). The modeled NH4+ concentrations in Zone 0 showed an increase up to 1,000 mg L−1 around Day 10 and then declined, as the urea is hydrolyzed in the first 20 days after applying the fertilizer. NH4+ and NO2− concentrations in Zone 1 mirrored the NH4+ concentration curve in Zone 0 but attained much lower peak concentrations (∼10 mg L−1). NO3− concentrations were highest in Zone 1 reaching a maximum of ∼250 mg L−1, as the model assumes nitrification is dominant in this zone but declined around Day 35 as NH4+ input into Zone 1 begins to diminish. In Zone 2, NO3− concentrations were tempered by reducing conditions that favor denitrification, peaking at ∼130 mg L−1 by the end of the model simulation. The N2 concentrations in Zone 2 increased for the entirety of the model and leveled off to a maximum concentration of 10 mg L−1 at the end of model simulation (see Figure 7).
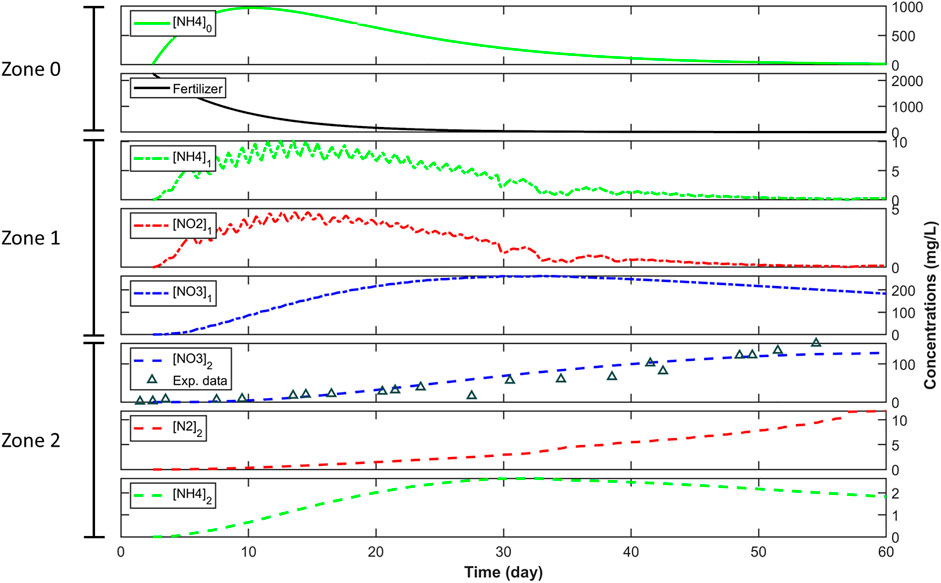
FIGURE 7. Model fit of reactions to the averaged NO3− concentrations measured in the fertilized soil columns (R2 = 0.88) and simulation of other nitrogen transformation processes in three zones 0, 1, and 2 used for the simple plug flow reactor model. A list of parameters and their estimated values are presented in Table 2.
4 Discussion
4.1 Impact of Winter Processes on Chloride and Sulfate Leachates
Of the analytes examined in the leachate samples, the patterns of Cl−, SO42−, and NO3−concentrations differed greatly between the fertilized and unfertilized columns. As Cl− is a relatively non-reactive ion that does not easily adsorb or react to the soil matrix, it serves as a tracer for the fertilizer front progressing through the fertilized soil columns (White and Broadley, 2001). If all applied fertilizer were incorporated into the applied rainwater solution, the effective average Cl− concentration would be ∼90 mg L−1. However, most of the applied fertilizer was likely dissolved in the first several rainwater applications, forming a concentrated Cl− front, and the observed peak at Day ∼30 represents the breakthrough of this front and is an indicator of the dissolved fertilizer passing through the fertilized soil columns.
Similarly, if all applied fertilizer were incorporated into the applied rainwater solution, the effective average SO42− concentration would be ∼4.3 mg L−1. Thus, the observed SO42− peaks in the fertilized column leachates (Figure 4) likely represents the breakthrough of a solution front concentrated in SO42− relative to the background SO42− concentration in the artificial rainwater that can be attributed to the applied fertilizer. The decrease in SO42− concentrations during the coldest portion of the weather sequence (Days 24–28) may be due to the formation of an ice barrier at the soil surface, creating anaerobic conditions in the soil subsurface and facilitating SO42- reduction. However, Sawicka et al. (2010) noted that the freezing process is detrimental to sulfate-reducing bacteria and their activity remains limited until they are reactivated during the thaw. This would imply that the lower SO42- concentrations observed during the freezing period of the experiment are likely a result of transport dynamics and not microbial processes.
4.2 Impact of Winter Processes on Nitrate Leachates
Low measured NO3− concentration in the artificial rainwater and applied fertilizer suggest that the increase of NO3− in the fertilized column leachates over the entirety of the experiment (Figure 5) is due to nitrification processes. Matzner and Borken (2008) reported that soil freezing can induce NO3− leaching in their field nutrient leaching freeze-thaw experiments. However, Henry. (2007) stated that while soil mesocosm experiments have generally shown increased potential for nutrient leaching following freeze-thaw events, the temperature fluctuation ranges utilized in these experiments are typically much larger (6–10°C) than those experienced in in situ soils (1–2°C). The soil columns in this study were subjected to ∼10°C air temperature fluctuations, which only induced moderate freeze-thaw soil temperature fluctuations (∼3°C). As unfertilized NO3− leachate concentrations did not increase following the onset of moderate FTCs during the mid-period of the experiment, our results appear to agree with previous studies that found FTCs had a negligible effect on N mineralization, assuming inorganic nitrogen generated through mineralization would subsequently be nitrified to NO3− (Larsen et al., 2002; Hentschel et al., 2008). However, low NO3− leachate concentrations in the unfertilized columns could be a result of the relatively low total N content in the soil from the sampling site (1.6 g kg−1) and the moderate intensity of the freezing temperatures applied in this experiment, as several previous studies have found that an increase in N mineralization can occur at colder freezing temperatures (Zhou et al., 2011; Jiang et al., 2018).
In a multi-site field freeze-thaw N loss study, Chantigny et al. (2019) found that artificial N fertilizers are susceptible to overwinter NO3− losses via nitrification, especially compared to organic N fertilizers, as organic fertilizers naturally included a carbon source to facilitate N immobilization. Similarly, in a soil jar batch experiment, Clark et al. (2009) determined that low temperature (−2 to 2 °C) nitrification can lead to NO3− accumulation or loss that is not mitigated by N immobilization. While substantial NO3− leaching was observed from the fertilized columns following a 1-day thaw (Day ∼30), leachate NO3− concentrations continued to increase for the remainder of the experiment despite warmer temperatures, suggesting that NO3− immobilization mechanisms did not resume or were not potent enough to stymie NO3− leaching. Extrapolating these NO3−-N losses assuming the experiment only captured approximately half of fertilizer-induced NO3− losses, approximately 2,891.4 and 2,216.2 µmol of N would have leached from Columns 1 and 2, respectively, had the experiment been allowed to continue. Considering the N input from the fertilizer amendments, approximately 27% and 20.7% of fertilizer N would have been converted to NO3−.
4.3 Modeling Nitrogen Transformations and Losses
The increase of modeled NH4+ in Zone 0 (Figure 7) is the result of rapid urea hydrolysis from the applied fertilizer. The transient NH4+ and NO2− concentrations in Zone 1 were generated and subsequently consumed by the active nitrification occurring in this zone. The oscillating nature of the NH4+ and NO2− concentrations shows the sensitivity of these nitrification substrates to the temperature changes during the freezing and thawing cycles. The NO3− concentrations in Zone 1 were high in the simulation because of high nitrification in this oxidized portion of the soil column. The increase and decrease of nitrification products are largely dictated by the supply of NH4+ entering this zone. The increasing N2 concentration in Zone 2 can be attributed to N2 serving as the denitrification end-product in this anoxic portion of the soil column.
Both average measured and modeled leachate NO3− concentrations trend upwards following an initial delay required for the rainwater to pass through the columns. While the model does not capture the stark freeze to thaw transitions of the 1-day and 3-day thaws occurring around Day ∼30 of the experiment, this appears to only affect the shapes of the trends. The modeled leachate NO3− concentration increase is more gradual than the measured leachate concentration. As Zone 2 was assumed to be entirely anoxic and dominated by denitrification, this sustained increase of leachate NO3− is likely bolstered by the continued contribution of recently produced NO3− in Zone 1. Although the measured leachate NO3− continued to increase until the end of the 55 days, the modeled NO3− concentration increase begins to level off by the end of the 60-day fit, suggesting that measured leachate NO3− concentrations would have begun to decline due to denitrification if the experiment had been allowed to continue.
The observed trends in measured and modeled NO3− leachate concentrations have implications for future FTC nutrient leaching studies. Fast dissolving NPK fertilizers like the fertilizer used in this study appear susceptible to NO3− loss via nitrification following FTCs. Nitrification-inhibited or controlled-release fertilizers are commonly put forward as means of reducing overwinter NO3− losses from fall-applied fertilizers (Di and Cameron, 2004; Randall and Vetsch, 2005; Thapa et al., 2016), however the impacts that evolving cold climate conditions have on these commonly used preventative measures remain understudied (Grant et al., 2020). Nitrification inhibitor efficiency has been demonstrated to be temperature and soil type dependent (Di and Cameron, 2004; McGeough et al., 2016; Guardia et al., 2018). Thus, examining how the changes in soil temperature and structure by FTC affect the nitrification inhibitor efficacy will be important for managing agricultural pollutants in colder climates.
5 Summary and Conclusion
This study examined the effect of FTCs on nutrients leaching from unfertilized and fertilized soil columns packed with agricultural soil. There was a significant difference in Cl−, SO42−, and NO3− leachate concentrations between the fertilized and unfertilized soil columns. In the fertilized column leachates, the elevated Cl− and SO42− concentrations can be attributed to the quick dissolution of Cl−-and SO42−-containing fertilizer components and transport while the increase in NO3− concentrations are a result of the active nitrification occurring in the oxidized upper portion of the soil columns. The model simulation results from a simple plug flow reactor model were consistent with the measured NO3− concentrations and indicated that intense nitrification of the urea occurred in the shallow, oxidized portion of the fertilized columns. Denitrification rates in the deeper anoxic portion of the column and immobilization mechanisms were not able to dampen leachate NO3− concentrations, but the end of the 60-day fit results suggested that the nitrification reactants are near exhausted by this time and leachate NO3− concentrations would decrease if the experiment had been allowed to continue. These findings indicate that fall-applied NPK fertilizers are prone to loss under soil FTCs during the NGS and future studies should investigate the effects of freeze-thaw cycling on methods commonly used to mitigate NO3− losses, such as nitrification inhibitors, as well as to compare losses under non-FTC conditions at similar stages of fertilizer transport through the soil. Future soil column studies should also seek to use intact soil cores to improve representativeness and actively measure gaseous nitrogen species via headspace measurements to better capture and model soil denitrification.
Data Availability Statement
The datasets presented in this study can be found in online repositories. The names of the repository/repositories and accession number(s) can be found below: https://www.frdr-dfdr.ca/repo/dataset/1519fbd9-6155-43c6-88cb-baa7bb5d07b4.
Author Contributions
KK: Designed and conducted the experiment, performed analysis, wrote original draft; GJ: Designed and conducted the experiment, reviewed, and edited; MG: Designed and performed the modeling approach; LH: Designed the experiment, reviewed and edited; DR: Reviewed and edited; PV: Designed the experiment, reviewed and edited; FR: Designed the experiment, reviewed and edited original draft.
Funding
This work was supported by the Canada Excellence Research Chair (CERC) program in Ecohydrology, the Global Water Futures (GWF) Winter Soil Processes in Transition project funded under the Canada First Excellence Research Fund, and Natural Sciences and Engineering Research Council (NSERC) Discovery Grant: RGPIN-2015-03801.
Conflict of Interest
The authors declare that the research was conducted in the absence of any commercial or financial relationships that could be construed as a potential conflict of interest.
Publisher’s Note
All claims expressed in this article are solely those of the authors and do not necessarily represent those of their affiliated organizations, or those of the publisher, the editors and the reviewers. Any product that may be evaluated in this article, or claim that may be made by its manufacturer, is not guaranteed or endorsed by the publisher.
Acknowledgments
We thank the rare Charitable Research Reserve (Cambridge, Ontario, Canada) for access to the field site where soil sample was collected. We also thank Marianne Vandergriendt and Shuhuan Li for assistance with laboratory experiments and analyses. We are indebted to Dr. Christina Smeaton for helpful discussions during the experimental design and data interpretations.
References
Almasri, M. N., and Kaluarachchi, J. J. (2004). Assessment and Management of Long-Term Nitrate Pollution of Ground Water in Agriculture-Dominated Watersheds. J. Hydrology 295 (1), 225–245. doi:10.1016/j.jhydrol.2004.03.013
Ambus, P. (1993). Control of Denitrification Enzyme Activity in a Streamside Soil. FEMS Microbiol. Ecol. 11 (3-4), 225–234. doi:10.1111/j.1574-6968.1993.tb05814.x
Beach, H. M., Laing, K. W., van de Walle, M., and Martin, R. C. (2018). The Current State and Future Directions of Organic No-Till Farming with Cover Crops in Canada, with Case Study Support. Sustain. Switz. 10 (2), 1–15. doi:10.3390/su10020373
Campbell, J. L., Ollinger, S. V., Flerchinger, G. N., Wicklein, H., Hayhoe, K., and Bailey, A. S. (2010). Past and Projected Future Changes in Snowpack and Soil Frost at the Hubbard Brook Experimental Forest, New Hampshire, USA. Hydrol. Process. 24 (17), 2465–2480. doi:10.1002/hyp.7666
Campbell, J. L., Socci, A. M., and Templer, P. H. (2014). Increased Nitrogen Leaching Following Soil Freezing Is Due to Decreased Root Uptake in a Northern Hardwood Forest. Glob. Change Biol. 20 (8), 2663–2673. doi:10.1111/gcb.12532
CAPMoN (2018). Major Ions. Retrieved from Environment and Climate Change Canada website: http://donnees.ec.gc.ca/data/air/monitor/monitoring-of-atmospheric-precipitation-chemistry/major-ions/
Chantigny, M. H., Bittman, S., Larney, F. J., Lapen, D., Hunt, D. E., Goyer, C., et al. (2019). A Multi-Region Study Reveals High Overwinter Loss of Fall-Applied Reactive Nitrogen in Cold and Frozen Soils. Can. J. Soil. Sci. 99 (2), 126–135. doi:10.1139/cjss-2018-0151
Clark, K., Chantigny, M. H., Angers, D. A., Rochette, P., and Parent, L.-É. (2009). Nitrogen Transformations in Cold and Frozen Agricultural Soils Following Organic Amendments. Soil Biol. Biochem. 41 (2), 348–356. doi:10.1016/j.soilbio.2008.11.009
Conant, B. (2004). Delineating and Quantifying Ground Water Discharge Zones Using Streambed Temperatures. Ground Water 42 (2), 243–257. doi:10.1111/j.1745-6584.2004.tb02671.x
Decker, K. L. M., Wang, D., Waite, C., and Scherbatskoy, T. (2003). Snow Removal and Ambient Air Temperature Effects on Forest Soil Temperatures in Northern Vermont. Soil Sci. Soc. Am. J. 67 (4), 1234–1242. doi:10.2136/sssaj2003.1234
Di, H. J., and Cameron, K. C. (2004). Effects of Temperature and Application Rate of a Nitrification Inhibitor, Dicyandiamide (DCD), on Nitrification Rate and Microbial Biomass in a Grazed Pasture Soil. Soil Res. 42 (8), 927–932. doi:10.1071/sr04050
Ehrl, B. N., Gharasoo, M., and Elsner, M. (2018). Isotope Fractionation Pinpoints Membrane Permeability as a Barrier to Atrazine Biodegradation in Gram-Negative Polaromonas Sp. Nea-C. Environ. Sci. Technol. 52 (7), 4137–4144. doi:10.1021/acs.est.7b06599
Elberling, B., and Brandt, K. K. (2003). Uncoupling of Microbial CO2 Production and Release in Frozen Soil and its Implications for Field Studies of Arctic C Cycling. Soil Biol. Biochem. 35 (2), 263–272. doi:10.1016/s0038-0717(02)00258-4
Funk, G., McClenaghan, W. A., and Holland, C. (1980). Water Wells and Ground Water Supplies in Ontario. Toronto: Ontario Government Bookstore and Publications Centre.
Gee, G., and Bauder, J. (1986). Methods of Soil Analysis: Part 1. Physical and Mineralogical Methods. Madison, USA: Soil Science Society of America.
Gentry, L. E., David, M. B., and McIsaac, G. F. (2014). Variation in Riverine Nitrate Flux and Fall Nitrogen Fertilizer Application in East-Central Illinois. J. Environ. Qual. 43 (4), 1467–1474. doi:10.2134/jeq2013.12.0499
Gharasoo, M., Ehrl, B. N., Cirpka, O. A., and Elsner, M. (2019). Modeling of Contaminant Biodegradation and Compound-specific Isotope Fractionation in Chemostats at Low Dilution Rates. Environ. Sci. Technol. 53 (3), 1186–1196. doi:10.1021/acs.est.8b02498
Gharasoo, M., Thullner, M., and Elsner, M. (2017). Introduction of a New Platform for Parameter Estimation of Kinetically Complex Environmental Systems. Environ. Model. Softw. 98, 12–20. doi:10.1016/j.envsoft.2017.09.005
Grant, R. F., Lin, S., and Hernandez-Ramirez, G. (2020). Modelling Nitrification Inhibitor Effects on N<sub>2</sub>O Emissions after Fall- and Spring-Applied Slurry by Reducing Nitrifier NH<sub>4</sub><sup>+</sup> Oxidation Rate. Biogeosciences 17 (7), 2021–2039. doi:10.5194/bg-17-2021-2020
Groffman, P., Butterbach-Bahl, K., Fulweiler, W., Gold, A., MorseStander, J. E., Tague, C., et al. (2009). Challenges to Incorporating Spatially and Temporally Explicit Phenomena (Hotspots and Hot Moments) in Denitrification Models. Biogeochemistry 93 (1), 49–77. doi:10.1007/s10533-008-9277-5
Guardia, G., Marsden, K. A., Vallejo, A., Jones, D. L., and Chadwick, D. R. (2018). Determining the Influence of Environmental and Edaphic Factors on the Fate of the Nitrification Inhibitors DCD and DMPP in Soil. Sci. Total Environ. 624, 1202–1212. doi:10.1016/j.scitotenv.2017.12.250
Halvorson, A. D., Snyder, C. S., Blaylock, A. D., and Del Grosso, S. J. (2014). Enhanced‐Efficiency Nitrogen Fertilizers: Potential Role in Nitrous Oxide Emission Mitigation. Agron. J. 106, 715–722. doi:10.2134/agronj2013.0081
Henry, H. A. L. (2007). Soil Freeze-Thaw Cycle Experiments: Trends, Methodological Weaknesses and Suggested Improvements. Soil Biol. Biochem. 39, 977–986. doi:10.1016/j.soilbio.2006.11.017
Hentschel, K., Borken, W., and Matzner, E. (2008). Repeated Freeze-Thaw Events Affect Leaching Losses of Nitrogen and Dissolved Organic Matter in a Forest Soil. Z. Pflanzenernähr. Bodenk. 171 (5), 699–706. doi:10.1002/jpln.200700154
Jensen, G., Krogstad, K., Rezanezhad, F., and Hug, L. Microbial Community Compositional Stability in Agricultural Soils during Freeze-Thaw and Fertilizer Stress. Front. Environ. Sci. (In review). doi:10.3389/fenvs.2022.908568
Jiang, N., Juan, Y., Tian, L., Chen, X., Sun, W., and Chen, L. (2018). Modification of the Composition of Dissolved Nitrogen Forms, Nitrogen Transformation Processes, and Diversity of Bacterial Communities by Freeze-Thaw Events in Temperate Soils. Pedobiologia 71, 41–49. doi:10.1016/j.pedobi.2018.08.004
Johnston, A. M., and Bruulsema, T. W. (2014). 4R Nutrient Stewardship for Improved Nutrient Use Efficiency. Procedia Eng. 83, 365–370. doi:10.1016/j.proeng.2014.09.029
Keesstra, S., Geissen, V., Mosse, K., Piiranen, S., Scudiero, E., Leistra, M., et al. (2012). Soil as a Filter for Groundwater Quality. Curr. Opin. Environ. Sustain. 4, 507–516. doi:10.1016/j.cosust.2012.10.007
King, T., Schoenau, J., and Elliott, J. (2017). Relationship between Manure Management Application Practices and Phosphorus and Nitrogen Export in Snowmelt Run-Off Water from a Black Chernozem Saskatchewan Soil. Sar 6 (2), 93–114. doi:10.5539/sar.v6n2p93
Kliewer, B. A., and Gilliam, J. W. (1995). Water Table Management Effects on Denitrification and Nitrous Oxide Evolution. Soil Sci. Soc. Am. J. 59 (6), 1694–1701. doi:10.2136/sssaj1995.03615995005900060027x
Knapen, A., Poesen, J., Govers, G., Gyssels, G., and Nachtergaele, J. (2007). Resistance of Soils to Concentrated Flow Erosion: A Review. Earth-Science Rev. 80, 75–109. doi:10.1016/j.earscirev.2006.08.001
Lalonde, V., Madramootoo, C. A., Trenholm, L., and Broughton, R. S. (1996). Effects of Controlled Drainage on Nitrate Concentrations in Subsurface Drain Discharge. Agric. Water Manag. 29 (2), 187–199. doi:10.1016/0378-3774(95)01193-5
Larsen, K. S., Jonasson, S., and Michelsen, A. (2002). Repeated Freeze-Thaw Cycles and Their Effects on Biological Processes in Two Arctic Ecosystem Types. Appl. Soil Ecol. 21 (3), 187–195. doi:10.1016/s0929-1393(02)00093-8
Maag, M., Malinovsky, M., and Nielsen, S. M. (1997). Kinetics and Temperature Dependence of Potential Denitrification in Riparian Soils. J. Environ. Qual. 26 (1), 215–223. doi:10.2134/jeq1997.00472425002600010031x
Mahvi, A. H., Nouri, J., Babaei, A. A., and Nabizadeh, R. (2005). Agricultural Activities Impact on Groundwater Nitrate Pollution. Int. J. Environ. Sci. Technol. 2 (1), 41–47. doi:10.1007/bf03325856
Marozava, S., Meyer, A. H., Pérez-de-Mora, A., Gharasoo, M., Zhuo, L., Wang, H., et al. (2019). Mass Transfer Limitation during Slow Anaerobic Biodegradation of 2-Methylnaphthalene. Environ. Sci. Technol. 53 (16), 9481–9490. doi:10.1021/acs.est.9b01152
Matzner, E., and Borken, W. (2008). Do freeze-thaw Events Enhance C and N Losses from Soils of Different Ecosystems? A Review. Eur. J. Soil Sci. 59, 2–22. doi:10.1111/j.1365-2389.2007.00992.x
McGeough, K. L., Watson, C. J., Müller, C., Laughlin, R. J., and Chadwick, D. R. (2016). Evidence that the Efficacy of the Nitrification Inhibitor Dicyandiamide (DCD) Is Affected by Soil Properties in UK Soils. Soil Biol. Biochem. 94, 222–232. doi:10.1016/j.soilbio.2015.11.017
McKenney, D. J., Johnson, G. P., and Findlay, W. I. (1984). Effect of Temperature on Consecutive Denitrification Reactions in Brookston Clay and Fox Sandy Loam. Appl. Environ. Microbiol. 47 (5), 919–926. doi:10.1128/aem.47.5.919-926.1984
Mikan, C. J., Schimel, J. P., and Doyle, A. P. (2002). Temperature Controls of Microbial Respiration in Arctic Tundra Soils above and below Freezing. Soil Biol. Biochem. 34 (11), 1785–1795. doi:10.1016/s0038-0717(02)00168-2
Monson, R. K., Lipson, D. L., Burns, S. P., Turnipseed, A. A., Delany, A. C., Williams, M. W., et al. (2006). Winter Forest Soil Respiration Controlled by Climate and Microbial Community Composition. Nature 439 (7077), 711–714. doi:10.1038/nature04555
Panikov, N. S., Flanagan, P. W., Oechel, W. C., Mastepanov, M. A., and Christensen, T. R. (2006). Microbial Activity in Soils Frozen to below −39°C. Soil Biol. Biochem. 38 (4), 785–794. doi:10.1016/j.soilbio.2005.07.004
Post, W. M., and Kwon, K. C. (2000). Soil Carbon Sequestration and Land-Use Change: Processes and Potential. Glob. Change Biol. 6, 317–327. doi:10.1046/j.1365-2486.2000.00308.x
Randall, G. W., and Vetsch, J. A. (2005). Nitrate Losses in Subsurface Drainage from a Corn-Soybean Rotation as Affected by Fall and Spring Application of Nitrogen and Nitrapyrin. J. Environ. Qual. 34 (2), 590–597. doi:10.2134/jeq2005.0590
Romero, C. M., Engel, R. E., Chen, C., Wallander, R., and Jones, C. A. (2017). Late-Fall, Winter, and Spring Broadcast Applications of Urea to No-Till Winter Wheat II. Fertilizer N Recovery, Yield, and Protein as Affected by NBPT. Soil Sci. Soc. Am. J. 81 (2), 331–340. doi:10.2136/sssaj2016.10.0333
Ryan, M. C., Kachanoski, R. G., and Gillham, R. W. (2000). Overwinter Soil Nitrogen Dynamics in Seasonally Frozen Soils. Can. J. Soil. Sci. 80 (4), 541–550. doi:10.4141/s99-017
Saad, O. A. L. O., and Conrad, R. (1993). Temperature Dependence of Nitrification, Denitrification, and Turnover of Nitric Oxide in Different Soils. Biol. Fertil. Soils 15 (1), 21–27. doi:10.1007/bf00336283
Saadat, S., Bowling, L., Frankenberger, J., and Kladivko, E. (2018). Nitrate and Phosphorus Transport through Subsurface Drains under Free and Controlled Drainage. Water Res. 142, 196–207. doi:10.1016/j.watres.2018.05.040
Sharpley, A., Weld, J., Beegle, D., Kleinman, P., Gburek, W., Moore, P., et al. (2003). Development of Phosphorus Indices for Nutrient Management Planning Strategies in the United States. J. Soil Water Conservation 58 (3), 137.
Smith, P., Cotrufo, M. F., Rumpel, C., Paustian, K., Kuikman, P. J., Elliott, J. A., et al. (2015). Biogeochemical Cycles and Biodiversity as Key Drivers of Ecosystem Services provided by Soils. SOIL 1, 665–685. doi:10.5194/soil-1-665-2015
Song, Y., Zou, Y., Wang, G., and Yu, X. (2017). Altered Soil Carbon and Nitrogen Cycles Due to the Freeze-Thaw Effect: A Meta-Analysis. Soil Biol. Biochem. 109, 35–49. doi:10.1016/j.soilbio.2017.01.020
Thapa, R., Chatterjee, A., Awale, R., McGranahan, D. A., and Daigh, A. (2016). Effect of Enhanced Efficiency Fertilizers on Nitrous Oxide Emissions and Crop Yields: A Meta-Analysis. Soil Sci. Soc. Am. J. 80 (5), 1121–1134. doi:10.2136/sssaj2016.06.0179
Watanabe, T., Tateno, R., Imada, S., Fukuzawa, K., Isobe, K., Urakawa, R., et al. (2019). The Effect of a Freeze-Thaw Cycle on Dissolved Nitrogen Dynamics and its Relation to Dissolved Organic Matter and Soil Microbial Biomass in the Soil of a Northern Hardwood Forest. Biogeochemistry 142 (3), 319–338. doi:10.1007/s10533-019-00537-w
White, P., and Broadley, M. R. (2001). Chloride in Soils and its Uptake and Movement within the Plant: A Review. Ann. Bot. 88, 967–988. doi:10.1006/anbo.2001.1540
Wipf, S., Sommerkorn, M., Stutter, M. I., Wubs, E. R. J., Van Der Wal, R., and Barger, N. (2015). Snow Cover, Freeze-Thaw, and the Retention of Nutrients in an Oceanic Mountain Ecosystem. Ecosphere 6, 1–16. doi:10.1890/es15-00099.1
Zhang, T. (2005). Influence of the Seasonal Snow Cover on the Ground Thermal Regime: An Overview. Rev. Geophys., 1–23. doi:10.1029/2004rg000157
Zhang, Y., Chen, W., Smith, S., Riseborough, D., and Cihlar, J. (2005). Soil Temperature in Canada during the Twentieth Century: Complex Responses to Atmospheric Climate Change. J. Geophys. Res. 110. doi:10.1029/2004jd004910
Zhang, Y., and Ma, N. (2018). Spatiotemporal Variability of Snow Cover and Snow Water Equivalent in the Last Three Decades over Eurasia. J. Hydrology 559, 238–251. doi:10.1016/j.jhydrol.2018.02.031
Zhou, W., Chen, H., Zhou, L., Lewis, B. J., Ye, Y., Tian, J., et al. (2011). Effect of Freezing-Thawing on Nitrogen Mineralization in Vegetation Soils of Four Landscape Zones of Changbai Mountain. Ann. For. Sci. 68 (5), 943–951. doi:10.1007/s13595-011-0100-4
Keywords: winter soil processes, freeze-thaw cycles, agricultural soils, fertilizer application, nutrient leaching
Citation: Krogstad K, Gharasoo M, Jensen G, Hug LA, Rudolph D, Van Cappellen P and Rezanezhad F (2022) Nitrogen Leaching From Agricultural Soils Under Imposed Freeze-Thaw Cycles: A Column Study With and Without Fertilizer Amendment. Front. Environ. Sci. 10:915329. doi: 10.3389/fenvs.2022.915329
Received: 07 April 2022; Accepted: 17 June 2022;
Published: 11 July 2022.
Edited by:
Carl Mitchell, University of Toronto Scarborough, CanadaCopyright © 2022 Krogstad, Gharasoo, Jensen, Hug, Rudolph, Van Cappellen and Rezanezhad. This is an open-access article distributed under the terms of the Creative Commons Attribution License (CC BY). The use, distribution or reproduction in other forums is permitted, provided the original author(s) and the copyright owner(s) are credited and that the original publication in this journal is cited, in accordance with accepted academic practice. No use, distribution or reproduction is permitted which does not comply with these terms.
*Correspondence: Konrad Krogstad, a2prcm9nc3RhZEB1d2F0ZXJsb28uY2E=