- 1Department of Zoology, College of Basic Science and Humanities, Odisha University of Agriculture and Technology, Bhubaneswar, India
- 2Department of Agriculture and Allied Sciences, C. V. Raman Global University, Bhubaneswar, India
- 3Environmental Sustainability Division, CSIR-Institute of Minerals and Materials Technology, Bhubaneswar, India
Alterations of soil characteristics that result in reduction in ecosystem services invariably cause soil quality degradation. Such changes could be caused due to a variety of stressors, which might be physical, chemical, or biological and come from both anthropogenic and natural causes. Out of the wide variety of soil pollutants, agrochemicals contaminate soil biota the most. Numerous research’ findings have confirmed that soil has been the most preferred site for the disposal of xenobiotics and therefore is likely to be the source of contamination for other natural resources like ground and surface waters. The ecological risk associated with contaminated soils depends on many physicochemical and biological processes that govern the transport and transformation of pesticides. Nevertheless, the persistence of pesticides in soil is a serious threat to both below and above ground organisms which play key roles in sustaining soil functions. One of the viable methods to decontaminate soil is by utilizing living soil biota selectively. This process called. Bioremediation has traditionally been employed to remove chemical residues from soil or to lessen their toxicity levels. Although microbes have been extensively used for bioremediation, chemical breakdown and remediation are significantly aided by certain dominant soil fauna, such as earthworms. Since they modify soil quality, earthworms are regarded as soil engineers. Earthworms can participate in the degradation of pesticide residues, either directly through the release of detoxifying enzymes in their gut or indirectly through their positive influence on microbial populations which could degrade pesticides. The earthworm supported pesticide degradation is largely confined to the gut microenvironment and the soil processed by the worms. The impact of earthworm species on pesticide degradation is widely variable which could be related to their feeding habits and microhabitats. Molecular docking studies have provided evidence in support of binding of organic molecules with agrochemicals. High level of organic matter in soil is expected to increase the binding of hydrophobic pesticides to organic ligands with consequent reduction in their bioavailability to microorganisms and increasing their persistence. Activities of earthworms is likely to induce growth of aerobic microbes capable of pesticide degradation. Among the various earthworm species, Lumbricus terrestris, Perionyx excavatus and Metaphire posthuma have shown promising results as remediating agents of pesticides contaminating farm soils. The present article focuses on the remediation process of hazardous pesticide polluted soil using biological agent like earthworm. This approach may be both efficient and environmentally beneficial.
Introduction
It has been well established through long term research that there is significant reduction in ecosystem services due to soil quality degradation (Palm et al., 2007). Pesticide application for protection of agricultural crops is a very common practice. However, due to the long half life of majority of these chemicals, there is always a risk of residual accumulation which could impact the soil biota and consequently the fertility of agricultural soils (Aktar et al., 2009; Leong et al., 2020). The environmental risk associated with contaminated soils depends on many physicochemical and biological processes that modulate the transport and transformation of pesticides (Gavrilescu, 2005; Arias-Estévez et al., 2008; Mirsal, 2008; Köhne et al., 2009; Odukkathil and Vasudevan, 2013). The pesticide residues accumulating in soil pose serious threat to both below and above ground organisms, vital for various soil functions (Sanchez-Hernandez, 2019; Mandal et al., 2020).
In recent years, bioremediation has been used for removal of pesticide residues from soil with an objective to down scale the deleterious impact on the soil organisms. This process involves the degradation or immobilization of the contaminants (Masciandaro et al., 2013). In comparison to conventional chemical and mechanical methods, bioremediation is an eco-friendly, effective and economically viable technology (Cummings, 2010). In majority of bioremediation methods, bacteria and fungi are used as remediating agents. However, this process is beset with a number of challenges such as metabolic adaptation of the microbes to specific contaminants such as organophosphate pesticides (Springael and Top, 2004; Singh and Walker, 2006). Degradation of chlorpyriphos, an organophosphate by hydrolysis and microbial activity generates compounds like chlorpyrifosoxon and 3,5,6-trichloro-2-pyridinol (Racke, 1993). These compounds are potentially toxic to microbes in soil (Singh and Walker, 2006; Fang et al., 2009; John and Shaike, 2015). Similarly, certain physicochemical properties of soil such as pH may interfere in the metabolic adaptation of microbes to degrade pesticides (Singh et al., 2003).
In some cases, diverse groups of organics are added to contaminated soils as nutrient source and bio stimulants. However, the addition of organics to contaminated soils may lead to adsorption of pesticides on to dissolved organic matter, thereby increasing the mobility of the pesticides (Bolan and Baskaran, 1996; Song et al., 2008; Bolan et al., 2011). Interactions between microbial populations such as competition and predation, might adversely influence the efficiency of bioremediation (Zhang et al., 2020). The use of pure preparations of detoxifying enzymes derived from microorganisms is a novel technique for preventing the negative impacts of ecological interactions on bioremediation (Sutherland et al., 2004; Nair and Jayachandran, 2017; Sharma et al., 2018). However, this strategy has some drawbacks, including the high cost of cell-free enzyme manufacturing, enzyme breakdown, and pesticide accessibility to the active region of enzymes (Sutherland et al., 2004; Scott et al., 2011).
Many authors have systematically addressed bioremediation of contaminated soils from various perspectives, ranging from technological and innovative perspectives to more ecological visions (Megharaj et al., 2011; Tyagi et al., 2011; Bharagava, 2017). However, there has not been a comprehensive analysis of the use of earthworms in vermiremediation. Hence, the purpose of this review is to explores and offers thorough insights into the applicability of earthworms as possible candidates for bioremediation of pesticide-contaminated soils.
Pesticide consumption on a global scale
The statistical data provided by the Organisation for Economic Co-operation and Development (OECD, 2013) indicated high pesticide consumption in many countries during the past decade. Certain countries in the European Union (EU) such as Spain, France, Italy, Germany, and Poland have the highest pesticide consumption rates. Few of these countries have significantly reduced their pesticide use since 2011, others have remained unchanged (Sanchez-Hernandez, 2019). Organophosphate (OP) insecticides are widely used in agriculture (Aktar et al., 2009; Sanchez-Hernandez, 2019). In the years 2010–2014, pesticide use decreased in African countries like Congo and Mauritius, while it increased significantly in Sudan, Malawi, Togo, and Rwanda (Sharma et al., 2019). According to the Pesticide Risk Reduction Programme, 160 of the 302 pesticides registered in Ethiopia included active components designated as WHO class II (moderately hazardous) compounds (Sanchez-Hernandez, 2019). Lack of awareness about pesticide use has led to the use of pesticides that come within the WHO risk classification system in Africa. Pesticide production in India began in 1952 with the production of benzene hexachloride, which was followed by DDT (Sharma et al., 2019). Pesticide synthesis since then has grown dramatically. India produced around 5,000 metric tonnes of pesticides in 1958, which increased to 85,000 metric tonnes in the mid-1990s with the registration of 145 chemicals, with insecticides being the most common pesticides produced. India is one of Asia’s main pesticide producers, generating 90,000 tonnes annually and ranking 12th in the world for pesticide output. In 2018, Brazil used 549,280 tonnes of pesticides. The three crops (soybean, corn, and sugar cane) that require the most pesticides to grow are widely cultivated throughout India (Hilbeck et al., 2015; Kumar et al., 2016; Atwood and Paisley-Jones, 2017; Disner et al., 2021). Consumption of OPs is also high in India and China. Insecticides were first used in Pakistan in 1954, when 250 metric tonnes of pesticides were imported. According to the Department of Agriculture’s Plant Protection Division, Nepal uses about 55.8 metric tonnes of pesticides per year. Pesticides are mostly used in the agriculture industry in Sri Lanka. After World War II, Sri Lanka was the first country to utilise DDT as a pesticide. In the United States, 500 million kg of pesticides are used annually at a cost of $10 billion. According to a report by Atwood and Paisley-Jones (2017), the United States spends about 16–18% of total global pesticide spending (Nyirenda et al., 2011; Abong’o et al., 2014; Schreinemachers et al., 2015; Herrero-Hernández et al., 2017; Menchen et al., 2017; Sharma et al., 2019). Gomes et al. (2020) examined the pesticide content of 172 samples of bananas, 59.3% of which were collected from Europe, 32.5% from Asia, and 8.1% from South America. According to the Brazilian, European, and Codex regulations, a total of 79.1%, 32.4%, and 42.6% of the samples were unacceptable and included pesticide residues. Brazil ranks second in the world for soybean production and is a significant producer and exporter of a number of other commodity crops. Brazil has risen to become one of the top four countries in the world for pesticide consumption as the usage of pesticides has significantly expanded along with significant increases in grain output (together with the US, EU, and China) (Paumgartten, 2020). Herbicides (56.1% of total sales pesticides) were the most popular in 1996, followed by insecticides (26%), and fungicides (15.4 percent) (Sanchez-Hernandez, 2019).
Pesticide contamination and soil health
Pesticides have become an environmental issue since these are difficult to store and dispose off safely. When these are applied at a larger scale, they pollute soil and water, harming the microflora and microfauna, and preventing plants from absorbing critical mineral nutrients. The contamination of water bodies is also caused by pesticide leaching. Pesticides contaminate drinking water and drain into the groundwater. A survey in the United States revealed, that the herbicides atrazine, simazine, prometon and metolachlor were the most frequently used pesticides (53% of a total of 2,542 samples), Another report from similar survey performed in Catalonia (Spain), showed that the herbicides simazine, atrazine and diuron were the most frequently detected pesticides. The organophosphorus diazinon (0.32–30.8 ng/l,min–max concentrations), dimethoate (0.24–2,277 ng/l), fenitrothion (8.15–19.5 ng/l) and malathion (2.57–86.6 ng/l) were detected in 51%, 10%, 3% and 7% of groundwater samples respectively (Köck-Schulmeyer et al., 2014; Toccalino et al., 2014).
Pesticides may harm soil’s indigenous microorganisms and disrupt the soil ecology, contaminating the food chain and endangering human health. Pesticides are now widely acknowledged to pose a severe threat to non-target creatures in the agroecosystems, such as earthworms, pollinators (bees), and natural pest adversaries.
Functional role of soil invertebrates and impact of pesticides
Soil invertebrates provide a wide range of ecosystem services that are critical for agricultural sustainability. Soil biodiversity supports self-sustaining ecosystem activities that power specialised processes including soil structure preservation, nutrient cycling, carbon transformations, and pest and disease regulation (Perrings et al., 2006; Kibblewhite et al., 2008; Chagnon et al., 2015). Soil porosity is altered by the burrowing activity of soil animals, which increases aeration, water infiltration and retention, and reduces compaction. Nematodes, springtails, earthworms, millipedes, and woodlice convert decayed matter and minerals into useful forms, cycle nutrients, and improve soil fertility. Gunstone et al. (2021) analysed biochemical biomarkers such as enzyme activity, membrane stability, gene expression, metabolism, DNA damage, and general oxidative stress, reproduction, survival, and behaviour like feeding rate, activity, burrowing, cast production, litter decomposition, avoidance, and respiration of Enchytraeids (pot worms) and found significant negative impact of insecticides and fungicides.
A vast number of transformation products (TPs) have been identified from a variety of pesticides. Few of the probable pesticide TPs have been measured in soil, indicating that more research is needed in this area. Pesticides that are hydrophobic, persistent and bioaccumulable are highly linked to soil. The organochlorine DDT, endosulfan, endrin, heptachlor, lindane, and their TPs are examples of pesticides that behave this way. Although the majority of them are now prohibited in agriculture, their residues are still prevalent. Chemicals used indiscriminately may work for a few years but may cause damage to beneficial organisms. Certain reports show clear adverse impact of these pesticides on soil microorganisms (Ramadass & Thiagarajan. 2017; Samal et al., 2019).
Bioremediation: A solution to pesticide contamination
Increased release of a wide spectrum of xenobiotic chemicals into the environment has come from the intensification of agriculture and manufacturing enterprises. Excessive hazardous waste loading has resulted in scarcity of clean water and soil disturbances thus affecting crop output. To clean up contaminated soil, bioremediation employs biological agents, primarily microorganisms such as yeast, fungi, or bacteria (Malla et al, 2018; Malla et al., 2022a; Malla et al., 2022b; Malla et al., 2022c). This approach is based on encouraging the establishment of certain microflora or microbial consortia that are native to polluted locations and capable of performing specified functions. This technique is the use of living organisms, primarily microorganisms to degrade the environmental pollutants into less toxic forms (Figures 1, 2). It detoxifies hazardous substances of the environment. The microorganisms used in these methods may be indigenous to a contaminated area or they may be isolated from elsewhere and brought to the contaminated site. Metabolic reaction of living organisms transforms contaminant compounds. Biodegradation of a compound is often a result of the actions of multiple organisms. Microorganisms must attack contaminants enzymatically and convert them to harmless compounds for effective bioremediation. Bioremediation can only be successful if the environment is congenial for microbial growth and activity. Therefore, it may be necessary to manipulate environmental parameters to speed up microbial growth and degradation (Nadim et al., 2000; Zhou, 2002; Atagana et al., 2003; Zhou & Hua, 2004).
Bioremediation procedures are often less expensive than traditional methods such as incineration. Some pollutants can be treated on-site, decreasing the danger of exposure to clean-up staff and potentially wider exposure as a result of transportation mishaps. Bioremediation is more widely accepted than other approaches because it is based on natural attenuation. Most bioremediation systems are operated under aerobic circumstances, but operating one under anaerobic conditions may allow microbial organisms to digest compounds that are otherwise resistant to degradation. Tolerance to pathogens can develop in contaminated environments. Some microorganisms or plants with a high degradability or hyperaccumulative feature of some contaminants will be easier to screen. In contrast, it will always be difficult to obtain miracle microbes or the hyperaccumulative plants required in bioremediation procedures in a clean environment. From the standpoint of biological evolution, contaminated environments have certain good benefits in terms of identifying specific bacteria and accumulative plants (Ryeom et al., 2000; Vidali, 2001; Zhou & Hua, 2004; Juwarkar et al., 2010).
Bioremediation is a cost-effective and environmentally acceptable alternative to advanced engineering-based approaches. However, using organisms like bacteria and fungi in bioremediation comes with a number of obstacles, including metabolic adaptation of microorganisms to the target pollutants. The organophosphorus insecticide chlorpyrifos is an example of this. The metabolites chlorpyrifosoxon and 3,5,6-trichloro-2-pyridinol are formed when this insecticide is degraded by chemical hydrolysis and microbiological activity. These metabolites, however, may be hazardous to microorganisms, limiting their mineralization capacity and the parent compound’s biodegradation. In addition to metabolic adaptation, bioremediation using microorganisms usually necessitates some form of biostimulation in order to keep the bacteria alive over time or even to speed up the destruction of the contaminants (Springael and Top, 2004; Singh and Walker, 2006; Cummings 2010).
The success of bioremediation may be harmed by ecological interactions between microbial populations, such as competition and predation. The use of pure preparations of detoxifying enzymes isolated from microorganisms is one novel technique for avoiding the negative impacts of ecological interactions on bioremediation. However, this strategy has some drawbacks, including the high cost of cell-free enzyme manufacturing, enzyme breakdown, and pesticide accessibility to the active region of enzymes. Together, these drawbacks and potential bioremediation solutions point to an integrated approach in which multiple biological pollutant degradation vectors are applied to the contaminated site simultaneously. If extracellular enzymes, microorganisms, and plants, as well as other potential soil decomposers (e.g., earthworms and springtails), are required for pesticide degradation, using a combination of these biological entities would be the ideal way to facilitate pesticide bioaccessibility, dispersion, uptake, and metabolism (Masciandaro et al., 2013; Megharaj and Naidu, 2017; Nair and Jayachandran 2017; Sharma et al., 2018).
Earthworms as candidates for remediation
Earthworms are considered soil engineers because of their influence on the physicochemical and biological qualities of soil. Their constant burrowing and feeding activities are a major driving force in improving and generating soil microhabitats, with far-reaching implications on both the below and aboveground systems. The most noticeable physical changes caused by earthworms are enhanced soil porosity, water infiltration, soil aeration, and plant root development (Capowiez et al., 2014). Earthworms create burrows as they crawl through the soil. These burrows can stay in the earth for a very long time. The amount of air and water that enter the soil rises as a result of earthworm burrows increasing soil porosity. Additionally, increased porosity reduces bulk density and promotes root development. Casts from earthworms, which include nitrogen, phosphorous, potassium, and magnesium, improve soil fertility. Microorganisms are also present in earthworm casts, and their numbers rise when organic waste is broken down in their stomachs. Plant growth is facilitated by the recirculation of nutrients from organic debris and the growth of microbes (Akhila and Entoori, 2022). Earthworms can boost plant productivity by encouraging the growth of both plant roots and shoots. Xiao et al. (2018) reported that plant growth increased by 20% as a result of changes in soil texture, nutrient mineralization influenced by earthworms. These animals encourage plant resistance to pests by increasing plant chemical defences (Wurst, 2010; Xiao et al., 2018; Sanchez-Hernandez, 2019).
Earthworms most often exert their activity on soil microorganisms. Earthworms play a vital role in the dispersion and proliferation of microbes. It is generally observed that the soil influenced by earthworms is characterized by high levels of microbial activity and biomass (Hoang et al., 2016).
Pesticides can be degraded by earthworms in two ways: directly through the release of pesticide-detoxifying enzymes in their gastrointestinal system, or indirectly through the stimulation and dispersion of pesticide degrading microbes. Pesticide breakdown by earthworms, on the other hand, is limited to the gastrointestinal system and the soil modified by worms such as casts and burrows. As a result, not all earthworm species will have the same impact on pesticide degradation, which will be determined by the ecological categories of earthworms. Endogeic and anecic earthworms will have a bigger impact on pesticide degradation than epigeic earthworms, because the former ingests more soil and engage in more burrowing activity. The role of earthworms in pesticide degradation considers two compartments of the drilosphere: the luminal microenvironment of the digestive canal (internal processes), and the microenvironment associated with the burrow walls (external processes). The earthworm body, casts, burrows, and diapause chambers are all part of the drilosphere (Hickman and Reid, 2008; Andriuzzi et al., 2013; Rodriguez-Campos et al., 2014). The methods of earthworm induced bioremediation include two processes: Internal and external (Figure 3).
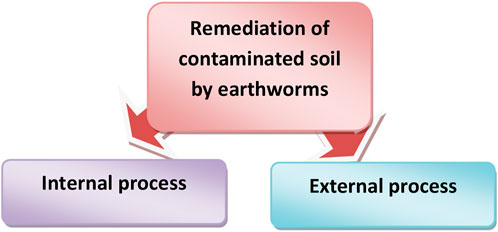
FIGURE 3. Biochemical mechanism used by earthworm to degrade pollutants. Internal process includes bioremediation in gastrointestinal tract of earthworm. External process includes degradation of pollutant using enzymes of cast.
Internal processes of pesticide detoxification
Variations in soil characteristics such as pH, dissolved organic carbon, and microbial proliferation are primarily responsible for earthworm-induced changes in metal speciation. However, the exact methods through which earthworms contribute to the rehabilitation of metal-polluted soils remain unknown. In case of organic pollutants, collaboration between earthworms and microbes is critical for their breakdown. Organic pollutants such as crude oils, polycyclic aromatic hydrocarbons, polychlorinated biphenyls, and pesticides may be degraded by symbiotic bacteria inhabiting the earthworm gastrointestinal system (Sanchez-Hernandez, 2019). The organochlorine insecticide endosulfan was degraded by a bacterial strain (Rhodococcus MTCC) isolated from gastrointestinal system of Metaphire posthuma (Hickman and Reid, 2008; Sizmur and Hodson, 2009; Rodriguez-Campos et al., 2014).
The gastrointestinal tract of earthworms can generate glycolytic digestive enzymes together with enzymes produced by gut symbionts, which contribute to the breakdown of ingested organic materials. Sanchez-Hernandez et al. (2009) demonstrated the importance of gastrointestinal secretion of carboxylesterases as pesticide-detoxifying enzymes, validating previous findings in L. terrestris. The activity of carboxylesterases is significant in the metabolism of xenobiotic compounds (Hatfield et al., 2016). Organophosphorus, carbamates, and synthetic pyrethroid pesticides interact with these esterases, assisting in their detoxification. Carboxylesterases catalyse the hydrolysis of synthetic pyrethroid pesticides to produce the alcohol and carboxylic acid. Carboxylesterases inactivate the oxidised metabolite of organophosphorus insecticides, known as “oxon” (Chambers et al., 2010), by irreversibly attaching the oxon molecule to the enzyme’s active site. The enzyme will be non-functional as well as the hazardous metabolite remains inactivated. As a result, this organophosphorus detoxification method is considered non-catalytic, and it is extremely reliant on the pesticide’s affinity for the enzyme’s active site and the amount of carboxylesterase molecules capable of interacting with the pesticide. Carboxylesterases interact with carbamate pesticides in the same way they interact with organophosphorus pesticides, however the degree of inhibition varies widely depending on the species and pesticide (Jackson et al., 2011; Nozaki et al., 2013).
The activity of luminal carboxylesterase in earthworms has been shown to be sensitive to oxon metabolites of organophosphorus insecticides (Sanchez-Hernandez et al., 2009). Extracellular detoxification reduces intestinal uptake of these hazardous compounds. Sanchez-Hernandez et al. (2014) found that luminal carboxylesterases are effective molecular scavengers of chlorpyrifos if the organophosphorus pesticide has been converted to the hazardous metabolite chlorpyrifos-oxon. Earthworm activity, on the other hand, has been shown in certain experiments to have no effect on pesticide persistence in the soil (Sanchez-Hernandez et al., 2018). Pesticide absorption is possibly hampered by changes in soil aggregates and greater organic carbon content in casts and burrow walls. Although many studies have suggested that earthworms play a substantial role in the degradation of pesticides such as atrazine, lindane, and chlorpyrifos, the true potential of earthworms to breakdown pesticides in the field has yet to be ascertained.
External processes of pesticide detoxification
Drilosphere components with significant microbiological and enzymatic activity include earthworm casts and burrows. Protease, glucosidase, alkaline phosphatase, and dehydrogenase activities are significantly higher in earthworm casts than in bulk soil. Casts have a higher concentration of extracellular enzymes than bulk soil due to their physicochemical features (high organic matter content and abundance of small particles). When extracellular enzymes bind to soil organomineral complexes, they become stable and active. This is likely to be the case with earthworm casts as well. Nonetheless, extracellular enzymes appear to be less active in older casts, possibly because to antagonistic microbial interactions and food loss. Clause et al. (2014), reported that the physicochemical parameters such as pH, K and Mg concentrations and total N content are significantly influenced by the soil type and the earthworm species, with Lumbricus. Terrestris having greater impact than Allolobophora chlorotica and Aporrectodea rosea. Although these workers did not measure any microbial properties, it can be correlated that casts with different physicochemical properties encourage different types of microbial activity and community structures. Anecic earthworms build middens around the entrance of their burrows by accumulating plant litter mixed with castings. These structures are microhabitats in which decomposers from the meso- and microfauna assist in the decomposition of organic materials. Middens should be viewed as regional hotspots for pollution degradation because of the significant microbial activity present in these locations (Furlong et al., 2002). Indeed, burrowing activity of L. terrestris has influence on microbial communities in the soil resulting in increased microbial activity and biomass, as well as associated extracellular enzyme activities (Hoang et al., 2016; Athmann et al., 2017).
The linings of the burrows are made up of fragmented litter which is combined with mucus and casts excreted by the earthworms as they move through the burrows. The adsorption of pesticides is facilitated by the accumulation of organic debris in the burrow linings. The chemical composition of earthworm burrows affects pesticide mobility because of two complementary processes: sorption on organic ligands and biodegradation. The presence of L. terrestris in the soil reduced the possibility for atrazine and metabolite leakage (Farenhorst and Bowman, 2000; Farenhorst et al., 2000). It was observed that the feeding activity of this earthworm species, as well as the higher proportion of organic carbon in burrow walls compared to bulk soil, are determining factors in limiting vertical atrazine transmission and increasing the non-extractable fraction of this herbicide.
The metabolically active microenvironments of earthworm casts, middens, and burrow walls may increase pesticide breakdown and immobilisation. The key mechanisms could have a significant impact on environmental fate of pesticides. First, as organic matter accumulates in these microsites, hydrophobic pesticides bind to organic ligands, decreasing their bioavailability to microorganisms (biodegradation) and increasing their persistence. Second, earthworm activity may promote the growth of native soil microbes that can breakdown pesticides (Sanchez-Hernandez et al., 2014; Katagi and Ose, 2015). Extracellular enzymes such as laccases, peroxidases, and carboxylesterases can metabolise phenolic chemicals, polycyclic aromatic hydrocarbons, polychlorinated biphenyls, azo dyes, and pesticides with a variety of chemical structures (Gianfreda et al., 2016). Furthermore, carboxylesterases have the ability to hydrolyze certain polyester polymers (Wei and Zimmermann, 2017). Soil carboxylesterase activity can hydrolyze malathion and many organophosphate pesticides. The stability and reactivity of carboxylesterases in soils infected with earthworms have been studied (Sanchez-Hernandez et al., 2014). In comparison to earthworm-free soils, L. terrestris induced soil carboxylesterase activity. The esterase activity remained constant for a long time even after removal of earthworms from soil. This is most likely because the extracellular enzyme was attached to soil organomineral complexes, ensuring its stability (Sanchez-Hernandez et al., 2014). Interestingly, the carboxylesterase activity caused by earthworms acted as a molecular scavenger of organophosphorus insecticides, rendering the deadly oxon metabolites inactive (Sanchez-Hernandez et al., 2015). This is one of the few examples indicating the direct interaction of soil enzymes and insecticides.
Earthworm species suitable as bioremediating agents
Earthworm niche types have inherent distinctions that influence their responses and behaviour to soil type, contaminant type, and a variety of other environmental factors. As a result, selecting the right earthworm species for the right conditions would be crucial. Certain approaches used in bioremediation utilizing earthworms are as follows.
1) Direct application of earthworms to polluted soils.
2) Co-application of earthworms to contaminated soils with an organic media, such as compost.
3) Feeding earthworms contaminated media as part of a feeding regimen.
A wide range of earthworms have been evaluated as potential remediating agents. The effects of earthworms on the behaviour, fate, and loss in response to the organic pollutants have also been studied. Earthworm assisted bioremediation increases hydrocarbon availability and remobilizes dichlorodiphenyltrichloroethane (DDT) and hexachlorocyclohexane (HCH) bound residues (Eijsackers et al., 2001). However, these findings contradicted those of According to Jiang et al. (2021), earthworm casts are a good choice for the affordable and simple remediation technique of glyphosate-contaminated soil. That could also enhance the quality of the farmed land and encourage crop growth. Farenhorst et al. (2000) found that earthworm (Lumbricus terrestris) activity increased pesticide sorption, effectively translocating, spreading, and mixing 14C-atrazine. The ability of earthworm gut microbiota to influence soil contaminant degradation has also been studied. Verma et al. (2006) reported the susceptibility of the earthworm Metaphire posthuma gut bacteria to the pesticide Endosulfan. Ramteke and Hans (1992) isolated microbes from the stomach of Pheretima posthuma treated with HCH and observed considerable HCH degradation.
Gevao et al. (2001) used the earthworm Aporrectodea longa at a rate of five individuals per 2 kg in soils polluted with non-extractable pesticide residues (14C-isoproturon, 14C-dicamba, and 14C-atrazine) for 28 days to assess subsequent breakdown, release, and uptake. They found that due to the physical activity of earthworms, previously bound pesticide residue was liberated in treatments with earthworms relative to those without earthworms. When the investigation was applied to newly introduced pesticides, it was observed that the non-extractable residues of 14C-isoproturon, 14C-dicamba, and 14C-atrazine in the non-earthworm treatment increased. Earthworms prevented the creation of the bound fraction while also promoting the release and mineralisation of bound residues (Hickman & Reid, 2008).
Elyamine and Hu (2020) demonstrated the favourable interaction between earthworms, Eisenia fetida and Aporrectodea caliginosa and rice straw in soil. Their interaction with soil microbes helped to promote phenanthrene breakdown in the soil. Both earthworms and rice straw increased the population of certain bacteria.
(Pseudomonas, Luteimonas, Rhodanobacter, Sphingomonas, Gemmatimonas, Flavobacterium, and Leifsonia), which have a strong and direct link to phenanthrene levels in soil. Alteration in soil’s nutrients and structure with the addition of earthworms and rice straw could change the microbial community’s structure which may enhance the breakdown of phenanthrene. Another study suggested the ability of E. eugeniae in combination with L. terrestris to remediate DDVP contaminated soil more effectively than E. eugeniae alone (Njoku et al., 2018). Sanchez-Hernandez et al. (2015) reported that the earthworm Lumbricus terrestris stimulated carboxylesterases (CbEs) activity and therefore could be considered as an efficient bio scavenger for organophosphorus pesticides. Another study revealed the role of three species of indigenous bacteria Corynebacterium sp., Sphingobacterium gobiense, and Kocuria flava and earthworms (at 5 and 10 earthworms/kg soil) to remove chlorpyrifos from contaminated soil in Sudan. The effect of bacterial consortia with high density earthworms on the percentage elimination of chlorpyrifos was 71.22% (Ahmed et al., 2020).
Using molecular docking as a tool to study the interaction between contaminants using earthworm model
During both academic and industrial trials, from the past decades molecular docking has been widely employed as a rapid and inexpensive technique. The method is based on determining the preferred orientation of small molecules in the active sites of their target receptors (Lengauer and Rarey, 1996). The scoring functions select the best conformation based on binding energies and binding affinities generated from a series of possible complexes. This preferred orientation has been found to be useful in predicting the optimal conformation between small molecules and biomolecule binding sites (Alonso et al., 2006). Despite the fact that this discipline has had enough time to consolidate, many aspects remain difficult, and there is still no simple or accurate way to quickly identify true ligands among a group of molecules, or to precisely identify the correct ligand conformation within the binding pocket of a given target molecule other than docking approach.
A group of experts has been studying the elimination mechanism of biodegradation at the molecular level for the past 2 decades (Ren et al., 2018). Molecular docking approach will aid in discovering the binding mechanism which is essential to gain a clear understanding on biodegradation of organic toxins and the role of organisms in the process. The pollutants which are discarded in agricultural soils might lead to increased contamination and pose a threat to environment. Furthermore, these contaminants can have a significant impact on the activities of soil organisms (Ghori et al., 2019). Microorganisms are involved in the majority of soil biochemical activities, and contaminants, surprisingly, can alter the metabolic processes of microbial communities (Li et al., 2020). A few soil organisms, particularly earthworms commonly known as “ecological engineers” (Singh et al., 2016), can effectively battle heavy metals and other pollution and are known as soil bio-indicators, which contribute to various ecosystem processes. Earthworms living on the surface of the soil can produce a rich amount of humic substances that stimulate the ion exchange process in heavy metals by forming hydrophobic mediated interaction between heavy metals and MTs protein of earthworm (Binding score: Cd=Cr = Cu = 980, and Zn = 372.) which was evident through docking process (Yuvaraj et al., 2021).
Bioremediation has been investigated by computational methodologies as a result of the use of computation in biological testing. One of the pillars of computational assisted bioremediation is the molecular docking. It aids in determining whether the active site can accommodate pollutant molecules or not. The stearic hindrance and nature of the active site pocket of earthworm enzymes or pesticides are critical in interacting with pollutants. The identification of consequential active site residues and binding features of these compounds under investigation could be used for site-directed mutagenic testing.
Limitation of vermiremediation
• Earthworms may not be able to thrive in very toxic soils, hence vermiremediation is limited to soils with a lower level of contamination (Javed & Hashmi, 2021).
• Another constraint could be the large number of earthworms needed for the remediation process.
• Bioremediation is confined to biodegradable chemicals. Not all chemicals are capable of complete and rapid breakdown.
• It is difficult to extrapolate from bench and pilot-scale investigations to full-scale field operations.
• The existence of metabolically competent earthworm species and suitable levels of nutrients and pollutants are important site characteristics required for success.
• Bioremediation using earthworms takes longer time than alternative treatment techniques like excavation and soil removal, or cremation (Vidali, 2001).
Concluding remarks
In spite of certain limitations, earthworms can be used directly in bioremediation procedures to increase the biodegradation of pollutants like pesticides because of their biological, chemical, and physical effects. Earthworms have been demonstrated to aerate soils, enhance microbial activity as well as increase nutrient availability and fertility, all of which are factors that have been linked to bioremediation. Earthworms have also been demonstrated to slow down the binding of organic pollutants to soils, release previously soil-bound toxins for degradation, and stimulate and disseminate organic contaminant-degrading microbes. This review attempts to emphasize on the effects of earthworms on pesticide contaminated soil environment hence boosting bioremediation potential. Molecular docking studies could help to evaluate the binding efficiency of organics and agrochemicals and their transport to organisms. Furthermore, earthworms have the potential to be used not only in the bioremediation of contaminated soils, but also in the rehabilitation of the soil structure and nutritional status. Further research is needed to develop site specific and sustainable bioremediation technologies using earthworms to yield better results (Bolan and Baskaran, 1996).
Author contributions
CSKM has evaluated and edited the manuscript. SS has written the manuscript and RRS has given ber efforts in Molecular docking part.
Conflict of interest
The authors declare that the research was conducted in the absence of any commercial or financial relationships that could be construed as a potential conflict of interest.
Publisher’s note
All claims expressed in this article are solely those of the authors and do not necessarily represent those of their affiliated organizations, or those of the publisher, the editors and the reviewers. Any product that may be evaluated in this article, or claim that may be made by its manufacturer, is not guaranteed or endorsed by the publisher.
References
Abong'o, D., Wandiga, S., Jumba, I., Madadi, V., and Kylin, H. (2014). Impacts of pesticides on human health and environment in the River Nyando catchment, Kenya. Int. J. Humanit. Arts, Med. Sci. 2 (3), 1–14.
Ahmed, N. A. M., Ali, S. H. N., Hammad, A. M. A., and Abdelbagi, A. O. (2020). Bacterial and vermi-remediation of soil contaminated with chlorpyrifos insecticide. Afr. J. Biotechnol. 19 (1), 26–32. doi:10.5897/AJB2019.16962
Akhila, A., and Entoori, K. (2022). Role of earthworms in soil fertility and its impact on agriculture: A review. Int. J. Fauna Biol. Stud. 9 (3), 55–63. doi:10.22271/23940522.2022.v9.i3a.907
Aktar, W., Sengupta, D., and Chowdhury, A. (2009). Impact of pesticides use in agriculture: Their benefits and hazards. Interdiscip. Toxicol. 2 (1), 1–12. doi:10.2478/v10102-009-0001-7
Alonso, H., Bliznyuk, A. A., and Gready, J. E. (2006). Combining docking and molecular dynamic simulations in drug design. Med. Res. Rev. 26 (5), 531–568. doi:10.1002/med.20067
Andriuzzi, W. S., Bolger, T., and Schmidt, O. (2013). The drilosphere concept: Fine-scale incorporation of surface residue-derived N and C around natural Lumbricus terrestris burrows. Soil Biol. Biochem. 64, 136–138. doi:10.1016/j.soilbio.2013.04.016
Arias-Estévez, M., López-Periago, E., Martínez-Carballo, E., Simal-Gándara, J., Mejuto, J. C., and García-Río, L. (2008). The mobility and degradation of pesticides in soils and the pollution of groundwater resources. Agric. Ecosyst. Environ. 123 (4), 247–260. doi:10.1016/j.agee.2007.07.011
Atagana, H. I., Haynes, R. J., and Wallis, F. M. (2003). Optimization of soil physical and chemical conditions for the bioremediation of creosote-contaminated soil. Biodegradation 14 (4), 297–307. doi:10.1023/a:1024730722751
Athmann, M., Kautz, T., Banfield, C., Bauke, S., Hoang, D. T., Lüsebrink, M., et al. (2017). Six months of L. terrestris L. activity in root-formed biopores increases nutrient availability, microbial biomass and enzyme activity. Appl. Soil Ecol. 120, 135–142. doi:10.1016/j.apsoil.2017.08.015
Atwood, D., and Paisley-Jones, C. (2017). Pesticides industry sales and usage: 2008–2012 market estimates. Washington, DC: US Environmental Protection Agency, 20460.
Bharagava, R. N. (Editor) (2017). Environmental pollutants and their bioremediation approaches (Boca Raton: CRC Press).
Bolan, N. S., Adriano, D. C., Kunhikrishnan, A., James, T., McDowell, R., and Senesi, N. (2011). “Dissolved organic matter: Biogeochemistry, dynamics, and environmental significance in soils,”. Advances in agronomy (Academic Press), 110, 1–75.
Bolan, N. S., and Baskaran, S. (1996). Characteristics of earthworm casts affecting herbicide sorption and movement. Biol. Fertil. Soils 22 (4), 367–372. doi:10.1007/bf00334585
Capowiez, Y., Sammartino, S., and Michel, E. (2014). Burrow systems of endogeic earthworms: Effects of earthworm abundance and consequences for soil water infiltration. Pedobiologia 57 (4-6), 303–309. doi:10.1016/j.pedobi.2014.04.001
Chagnon, M., Kreutzweiser, D., Mitchell, E. A., Morrissey, C. A., Noome, D. A., and Van der Sluijs, J. P. (2015). Risks of large-scale use of systemic insecticides to ecosystem functioning and services. Environ. Sci. Pollut. Res. 22 (1), 119–134. doi:10.1007/s11356-014-3277-x
Chambers, J. E., Meek, E. C., and Chambers, H. W. (2010). “The metabolism of organophosphorus insecticides,” in Hayes' handbook of pesticide toxicology (Academic Press), 1399–1407.
Clause, J., Barot, S., Richard, B., Decaëns, T., and Forey, E. (2014). The interactions between soil type and earthworm species determine the properties of earthworm casts. Appl. Soil Ecol. 83, 149–158. doi:10.1016/j.apsoil.2013.12.006
Disner, G. R., Falcão, M. A. P., Andrade‐Barros, A. I., Leite dos Santos, N. V., Soares, A. B. S., Marcolino‐Souza, M., et al. (2021). The toxic effects of glyphosate, chlorpyrifos, abamectin, and 2, 4‐D on animal models: A systematic review of Brazilian studies. Integr. Environ. Assess. Manag. 17 (3), 507–520. doi:10.1002/ieam.4353
Eijsackers, H., Van Gestel, C. A. M., De Jonge, S., Muijs, B., and Slijkerman, D. (2001). Polycyclic aromatic hydrocarbon-polluted dredged peat sediments and earthworms: A mutual interference. Ecotoxicology 10 (1), 35–50. doi:10.1023/a:1008954706150
Elyamine, A. M., and Hu, C. (2020). Earthworms and rice straw enhanced soil bacterial diversity and promoted the degradation of phenanthrene. Environ. Sci. Eur. 32 (1), 124–212. doi:10.1186/s12302-020-00400-y
Fang, H., Yunlong, Y. U., Xiaoqiang, C. H. U., Xiuguo, W. A. N. G., Xiaoe, Y. A. N. G., and Jingquan, Y. U. (2009). Degradation of chlorpyrifos in laboratory soil and its impact on soil microbial functional diversity. J. Environ. Sci. 21 (3), 380–386. doi:10.1016/s1001-0742(08)62280-9
Farenhorst, A., and Bowman, B. T. (2000). Sorption of atrazine and metolachlor by earthworm surface castings and soil. J. Environ. Sci. Health Part B 35 (2), 157–173. doi:10.1080/03601230009373261
Farenhorst, A., Topp, E., Bowman, B. T., and Tomlin, A. D. (2000). Earthworm burrowing and feeding activity and the potential for atrazine transport by preferential flow. Soil Biol. Biochem. 32 (4), 479–488. doi:10.1016/s0038-0717(99)00177-7
Furlong, M. A., Singleton, D. R., Coleman, D. C., and Whitman, W. B. (2002). Molecular and culture-based analyses of prokaryotic communities from an agricultural soil and the burrows and casts of the earthworm Lumbricus rubellus. Appl. Environ. Microbiol. 68 (3), 1265–1279. doi:10.1128/aem.68.3.1265-1279.2002
Gavrilescu, M. (2005). Fate of pesticides in the environment and its bioremediation. Eng. Life Sci. 5 (6), 497–526. doi:10.1002/elsc.200520098
Gevao, B., Mordaunt, C., Semple, K. T., Piearce, T. G., and Jones, K. C. (2001). Bioavailability of nonextractable (bound) pesticide residues to earthworms. Environ. Sci. Technol. 35 (3), 501–507. doi:10.1021/es000144d
Ghori, N. H., Ghori, T., Hayat, M. Q., Imadi, S. R., Gul, A., Altay, V., et al. (2019). Heavy metal stress and responses in plants. Int. J. Environ. Sci. Technol. (Tehran). 16 (3), 1807–1828. doi:10.1007/s13762-019-02215-8
Gianfreda, L., Rao, M. A., Scelza, R., and De La Luz Mora, M. (2016). “Role of enzymes in environment cleanup/remediation,” in Agro-industrial wastes as feedstock for enzyme production (Academic Press), 133–155.
Gomes, H. D. O., Menezes, J. M. C., da Costa, J. G. M., Coutinho, H. D. M., Teixeira, R. N. P., and do Nascimento, R. F. (2020). Evaluating the presence of pesticides in bananas: An integrative review. Ecotoxicol. Environ. Saf. 189, 110016. doi:10.1016/j.ecoenv.2019.110016
Gunstone, T., Cornelisse, T., Klein, K., Dubey, A., and Donley, N. (2021). Pesticides and soil invertebrates: A hazard assessment. Front. Environ. Sci. 9, 122. doi:10.3389/fenvs.2021.643847
Hatfield, M. J., Umans, R. A., Hyatt, J. L., Edwards, C. C., Wierdl, M., Tsurkan, L., et al. (2016). Carboxylesterases: General detoxifying enzymes. Chemico-biological Interact. 259, 327–331. doi:10.1016/j.cbi.2016.02.011
Herrero-Hernández, E., Rodríguez-Cruz, M. S., Pose-Juan, E., Sánchez-González, S., Andrades, M. S., and Sánchez-Martín, M. J. (2017). Seasonal distribution of herbicide and insecticide residues in the water resources of the vineyard region of La Rioja (Spain). Sci. Total Environ. 609, 161–171. doi:10.1016/j.scitotenv.2017.07.113
Hickman, Z. A., and Reid, B. J. (2008). Earthworm assisted bioremediation of organic contaminants. Environ. Int. 34 (7), 1072–1081. doi:10.1016/j.envint.2008.02.013
Hilbeck, A., Binimelis, R., Defarge, N., Steinbrecher, R., Székács, A., Wickson, F., et al. (2015). No scientific consensus on GMO safety. Environ. Sci. Eur. 27 (1), 4–6. doi:10.1186/s12302-014-0034-1
Hoang, D. T., Pausch, J., Razavi, B. S., Kuzyakova, I., Banfield, C. C., and Kuzyakov, Y. (2016). Hotspots of microbial activity induced by earthworm burrows, old root channels, and their combination in subsoil. Biol. Fertil. Soils 52 (8), 1105–1119. doi:10.1007/s00374-016-1148-y
Jackson, C. J., Oakeshott, J. G., Sanchez-Hernandez, J. C., and Wheelock, C. E. (2011). Carboxylesterases in the metabolism and toxicity of pesticides. In Editors T. Satoh, and R. C. Gupta (Anticholinesterase Pesticides: John Wiley and Sons, Inc.), 57–75. doi:10.1002/9780470640500.ch5
Javed, F., and Hashmi, I. (2021). Vermiremediation–remediation of soil contaminated with oil using earthworm (Eisenia fetida). Soil Sediment Contam. Int. J. 30 (6), 1–25. doi:10.1080/15320383.2021.1885343
Jiang, L., Pan, B., Liang, J., Wang, B., Yang, Y., and Lin, Y. (2021). Earthworm casts restrained the accumulation and phytotoxicity of soil glyphosate to cowpea (Vigna unguiculata (L.) Walp.) plants. Chemosphere 279, 130571.
John, E. M., and Shaike, J. M. (2015). Chlorpyrifos: Pollution and remediation. Environ. Chem. Lett. 13 (3), 269–291. doi:10.1007/s10311-015-0513-7
Juwarkar, A. A., Singh, S. K., and Mudhoo, A. (2010). A comprehensive overview of elements in bioremediation. Rev. Environ. Sci. Biotechnol. 9 (3), 215–288. doi:10.1007/s11157-010-9215-6
Katagi, T., and Ose, K. (2015). Toxicity, bioaccumulation and metabolism of pesticides in the earthworm. J. Pestic. Sci. D15-003, 69–81. doi:10.1584/jpestics.d15-003
Kibblewhite, M. G., Ritz, K., and Swift, M. J. (2008). Soil health in agricultural systems. Phil. Trans. R. Soc. B 363 (1492), 685–701. doi:10.1098/rstb.2007.2178
Köck-Schulmeyer, M., Ginebreda, A., Postigo, C., Garrido, T., Fraile, J., de Alda, M. L., et al. (2014). Four-year advanced monitoring program of polar pesticides in groundwater of Catalonia (NE-Spain). Sci. total Environ. 470, 1087–1098. doi:10.1016/j.scitotenv.2013.10.079
Köhne, J. M., Köhne, S., and Šimůnek, J. (2009). A review of model applications for structured soils: B) pesticide transport. J. Contam. hydrology 104 (1-4), 36–60. doi:10.1016/j.jconhyd.2008.10.003
Kumar, S., Kaushik, G., and Villarreal-Chiu, J. F. (2016). Scenario of organophosphate pollution and toxicity in India: A review. Environ. Sci. Pollut. Res. 23 (10), 9480–9491. doi:10.1007/s11356-016-6294-0
Lengauer, T., and Rarey, M. (1996). Computational methods for biomolecular docking. Curr. Opin. Struct. Biol. 6 (3), 402–406. doi:10.1016/s0959-440x(96)80061-3
Leong, W. H., Teh, S. Y., Hossain, M. M., Nadarajaw, T., Zabidi-Hussin, Z., Chin, S. Y., et al. (2020). Application, monitoring and adverse effects in pesticide use: The importance of reinforcement of Good Agricultural Practices (GAPs). J. Environ. Manag. 260, 109987. doi:10.1016/j.jenvman.2019.109987
Li, S., Zhao, B., Jin, M., Hu, L., Zhong, H., and He, Z. (2020). A comprehensive survey on the horizontal and vertical distribution of heavy metals and microorganisms in soils of a Pb/Zn smelter. J. Hazard. Mater. 400, 123255. doi:10.1016/j.jhazmat.2020.123255
Malla, M. A., Dubey, A., Yadav, S., Kumar, A., Hashem, A., and Abd_Allah, E. F. (2018). Understanding and designing the strategies for the microbe-mediated remediation of environmental contaminants using omics approaches. Front. Microbiol. 9, 1132. doi:10.3389/fmicb.2018.01132
Malla, M. A., Dubey, A., Kumar, A., and Yadav, S. (2022a). Metagenomic analysis displays the potential predictive biodegradation pathways of the persistent pesticides in agricultural soil with a long record of pesticide usage. Microbiol. Res. 261, 127081. doi:10.1016/j.micres.2022.127081
Malla, M. A., Dubey, A., Raj, A., Kumar, A., Upadhyay, N., and Yadav, S. (2022b). Emerging frontiers in microbe mediated pesticide remediation: Unveiling role of omics and in silico approaches in engineered environment. Environ. Pollut. 299, 118851. doi:10.1016/j.envpol.2022.118851
Malla, M. A., Dubey, A., Kumar, A., Vennapu, D. R., Upadhyay, N., Pradhan, D., et al. (2022c). Process optimization of cypermethrin biodegradation by regression analysis and parametric modeling along with biochemical degradation pathway. Environ. Sci. Pollut. Res. Int. 219, 1–10. doi:10.1007/s11356-022-21191-0
Mandal, A., Sarkar, B., Mandal, S., Vithanage, M., Patra, A. K., and Manna, M. C. (2020). “Impact of agrochemicals on soil health,” in Agrochemicals detection, treatment and remediation (Butterworth-Heinemann), 161–187.
Masciandaro, G., Macci, C., Peruzzi, E., Ceccanti, B., and Doni, S. (2013). Organic matter–microorganism–plant in soil bioremediation: A synergic approach. Rev. Environ. Sci. Biotechnol. 12 (4), 399–419. doi:10.1007/s11157-013-9313-3
Megharaj, M., and Naidu, R. (2017). Soil and brownfield bioremediation. Microb. Biotechnol. 10, 1244–1249. doi:10.1111/1751-7915.12840
Megharaj, M., Ramakrishnan, B., Venkateswarlu, K., Sethunathan, N., and Naidu, R. (2011). Bioremediation approaches for organic pollutants: A critical perspective. Environ. Int. 37 (8), 1362–1375. doi:10.1016/j.envint.2011.06.003
Menchen, A., Heras, J. D. L., and Alday, J. J. G. (2017). Pesticide contamination in groundwater bodies in the Júcar River European Union pilot basin (SE Spain). Environ. Monit. Assess. 189, 146. doi:10.1007/s10661-017-5827-4
Nadim, F., Hoag, G. E., Liu, S., Carley, R. J., and Zack, P. (2000). Detection and remediation of soil and aquifer systems contaminated with petroleum products: An overview. J. Petroleum Sci. Eng. 26 (1-4), 169–178. doi:10.1016/s0920-4105(00)00031-0
Nair, I. C., and Jayachandran, K. (2017). “Enzymes for bioremediation and biocontrol,” in Bioresources and bioprocess in biotechnology (Singapore: Springer), 75–97.
Njoku, K. L., Ogwara, C. A., Adesuyi, A. A., and Akinola, M. O. (2018). Vermiremediation of pesticide contaminated soil using Eudrilus euginae and Lumbricus terrestris. EnvironmentAsia 11 (3), 133–147. doi:10.14456/ea.2018.43
Nozaki, M., Ito, K., Miura, C., and Miura, T. (2013). Examination of digestive enzyme distribution in gut tract and functions of intestinal caecum, in megascolecid earthworms (Oligochaeta: Megascolecidae) in Japan. Zoological Sci. 30 (9), 710–715. doi:10.2108/zsj.30.710
Nyirenda, S. P., Sileshi, G. W., Belmain, S. R., Kamanula, J. F., Mvumi, B. M., Sola, P., et al. (2011). Farmers’ ethno-ecological knowledge of vegetable pests and pesticidal plant use in Malawi and Zambia. Afr. J. Agric. Res. 6 (6), 1525–1537. doi:10.5897/AJAR.9000346
Odukkathil, G., and Vasudevan, N. (2013). Toxicity and bioremediation of pesticides in agricultural soil. Rev. Environ. Sci. Biotechnol. 12 (4), 421–444. doi:10.1007/s11157-013-9320-4
Palm, C., Sanchez, P., Ahamed, S., and Awiti, A. (2007). Soils: A contemporary perspective. Annu. Rev. Environ. Resour. 32, 99–129. doi:10.1146/annurev.energy.31.020105.100307
Paumgartten, F. J. (2020). Pesticides and public health in Brazil. Curr. Opin. Toxicol. 22, 7–11. doi:10.1016/j.cotox.2020.01.003
Perrings, C., Jackson, L., Bawa, K., Brussaard, L., Brush, S., Gavin, T., et al. (2006). Biodiversity in agricultural landscapes: Saving natural capital without losing interest. Conserv. Biol. 20 (2), 263–264. doi:10.1111/j.1523-1739.2006.00390.x
Ramadass, M., and Thiagarajan, P. (2017). Effective pesticide nano formulations and their bacterial degradation. IOP Conf. Ser. Mat. Sci. Eng. 263, 022050. doi:10.1088/1757-899x/263/2/022050
Ramteke, P. W., and Hans, R. K. (1992). Isolation of hexachlorocyclohexane (HCH) degrading microorganisms from earthworm got. J. Environ. Sci. Health Part A Environ. Sci. Eng. Toxicol. 27 (8), 2113–2122. doi:10.1080/10934529209375844
Ren, X., Zeng, G., Tang, L., Wang, J., Wan, J., Feng, H., et al. (2018). Effect of exogenous carbonaceous materials on the bioavailability of organic pollutants and their ecological risks. Soil Biol. Biochem. 116, 70–81. doi:10.1016/j.soilbio.2017.09.027
Rodriguez-Campos, J., Dendooven, L., Alvarez-Bernal, D., and Contreras-Ramos, S. M. (2014). Potential of earthworms to accelerate removal of organic contaminants from soil: A review. Appl. Soil Ecol. 79, 10–25. doi:10.1016/j.apsoil.2014.02.010
Ryeom, T. K., Lee, I. G., Son, S. Y., and Ahn, T. Y. (2000). Degradation of phenanthrene by Sphingomonas sp. 1-21 isolated from oil-contaminated soil. J. Microbiol. Biotechnol. 10 (5), 724–727.
Samal, S., Sahoo, S., and Mishra, C. S. K. (2019). Evaluating the effect of monocrotophos and glyphosate on microbial population and certain important exoenzyme activities in soil. J. Environ. Biol. 40 (2), 226–234. doi:10.22438/jeb/40/2/mrn-822
Sanchez-Hernandez, J. C. (2019). “Bioremediation of pesticide-contaminated soils by using earthworms,” in Bioremediation of agricultural soils (Boca Raton: CRC Press), 165–192.
Sanchez-Hernandez, J. C., Mazzia, C., Capowiez, Y., and Rault, M. (2009). Carboxylesterase activity in earthworm gut contents: Potential (eco) toxicological implications. Comp. Biochem. Physiology Part C Toxicol. Pharmacol. 150 (4), 503–511. doi:10.1016/j.cbpc.2009.07.009
Sanchez-Hernandez, J. C., Morcillo, S. M., del Pino, J. N., and Ruiz, P. (2014). Earthworm activity increases pesticide-sensitive esterases in soil. Soil Biol. Biochem. 75, 186–196. doi:10.1016/j.soilbio.2014.04.015
Sanchez-Hernandez, J. C., del Pino, J. N., and Domínguez, J. (2015). Earthworm-induced carboxylesterase activity in soil: Assessing the potential for detoxification and monitoring organophosphorus pesticides. Ecotoxicol. Environ. Saf. 122, 303–312. doi:10.1016/j.ecoenv.2015.08.012
Sanchez-Hernandez, J. C., Del Pino, J. N., Capowiez, Y., Mazzia, C., and Rault, M. (2018). Soil enzyme dynamics in chlorpyrifos-treated soils under the influence of earthworms. Sci. Total Environ. 612, 1407–1416. doi:10.1016/j.scitotenv.2017.09.043
Schreinemachers, P., Afari-Sefa, V., Heng, C. H., Dung, P. T. M., Praneetvatakul, S., and Srinivasan, R. (2015). Safe and sustainable crop protection in southeast Asia: Status, challenges and policy options. Environ. Sci. Policy 54, 357–366. doi:10.1016/j.envsci.2015.07.017
Scott, C., Begley, C., Taylor, M. J., Pandey, G., Momiroski, V., French, N., et al. (2011). “Free-enzyme bioremediation of pesticides: A case study for the enzymatic remediation of organophosphorous insecticide residues,” in Pesticide mitigation strategies for surface water quality (American Chemical Society), 155–174.
Sharma, B., Dangi, A. K., and Shukla, P. (2018). Contemporary enzyme based technologies for bioremediation: A review. J. Environ. Manag. 210, 10–22. doi:10.1016/j.jenvman.2017.12.075
Sharma, A., Kumar, V., Shahzad, B., Tanveer, M., Sidhu, G. P. S., Handa, N., et al. (2019). Worldwide pesticide usage and its impacts on ecosystem. SN Appl. Sci. 1 (11), 1–16. doi:10.1007/s42452-019-1485-1
Singh, B. K., and Walker, A. (2006). Microbial degradation of organophosphorus compounds. FEMS Microbiol. Rev. 30 (3), 428–471. doi:10.1111/j.1574-6976.2006.00018.x
Singh, B. K., Walker, A., Morgan, J. A. W., and Wright, D. J. (2003). Effects of soil pH on the biodegradation of chlorpyrifos and isolation of a chlorpyrifos-degrading bacterium. Appl. Environ. Microbiol. 69 (9), 5198–5206. doi:10.1128/aem.69.9.5198-5206.2003
Singh, S., Singh, J., and Vig, A. P. (2016). Earthworm as ecological engineers to change the physico-chemical properties of soil: Soil vs vermicast. Ecol. Eng. 90, 1–5. doi:10.1016/j.ecoleng.2016.01.072
Sizmur, T., and Hodson, M. E. (2009). Do earthworms impact metal mobility and availability in soil?–A review. Environ. Pollut. 157 (7), 1981–1989. doi:10.1016/j.envpol.2009.02.029
Song, N. H., Chen, L., and Yang, H. (2008). Effect of dissolved organic matter on mobility and activation of chlorotoluron in soil and wheat. Geoderma 146 (1-2), 344–352. doi:10.1016/j.geoderma.2008.05.031
Springael, D., and Top, E. M. (2004). Horizontal gene transfer and microbial adaptation to xenobiotics: New types of mobile genetic elements and lessons from ecological studies. Trends Microbiol. 12 (2), 53–58. doi:10.1016/j.tim.2003.12.010
Sutherland, T. D., Horne, I., Weir, K. M., Coppin, C. W., Williams, M. R., Selleck, M., et al. (2004). Enzymatic bioremediation: From enzyme discovery to applications. Clin. Exp. Pharmacol. Physiol. 31 (11), 817–821. doi:10.1111/j.1440-1681.2004.04088.x
Toccalino, P. L., Gilliom, R. J., Lindsey, D., and Rupert, M. G. (2014). Pesticides in groundwater of the United States: Decadal‐scale changes, 1993–2011. Groundwater 52 (S1), 112–125. doi:10.1111/gwat.12176
Tyagi, M., da Fonseca, M. M. R., and de Carvalho, C. C. (2011). Bioaugmentation and biostimulation strategies to improve the effectiveness of bioremediation processes. Biodegradation 22 (2), 231–241.
Verma, K., Agrawal, N., Farooq, M., Misra, R. B., and Hans, R. K. (2006). Endosulfan degradation by a Rhodococcus strain isolated from earthworm gut. Ecotoxicol. Environ. Saf. 64 (3), 377–381. doi:10.1016/j.ecoenv.2005.05.014
Vidali, M. (2001). Bioremediation. an overview. Pure Appl. Chem. 73 (7), 1163–1172. doi:10.1351/pac200173071163
Wei, R., and Zimmermann, W. (2017). Microbial enzymes for the recycling of recalcitrant petroleum‐based plastics: How far are we? Microb. Biotechnol. 10 (6), 1308–1322. doi:10.1111/1751-7915.12710
Wurst, S. (2010). Effects of earthworms on above-and belowground herbivores. Appl. Soil Ecol. 45 (3), 123–130. doi:10.1016/j.apsoil.2010.04.005
Xiao, Z., Wang, X., Koricheva, J., Kergunteuil, A., Le Bayon, R. C., Liu, M., et al. (2018). Earthworms affect plant growth and resistance against herbivores: A meta‐analysis. Funct. Ecol. 32 (1), 150–160. doi:10.1111/1365-2435.12969
Yuvaraj, A., Govarthanan, M., Karmegam, N., Biruntha, M., Kumar, D. S., Arthanari, M., et al. (2021). Metallothionein dependent-detoxification of heavy metals in the agricultural field soil of industrial area: Earthworm as field experimental model system. Chemosphere 267, 129240. doi:10.1016/j.chemosphere.2020.129240
Zhang, H., Yuan, X., Xiong, T., Wang, H., and Jiang, L. (2020). Bioremediation of co-contaminated soil with heavy metals and pesticides: Influence factors, mechanisms and evaluation methods. Chem. Eng. J. 398, 125657. doi:10.1016/j.cej.2020.125657
Zhou, Q., and Hua, T. (2004). Bioremediation: A review of applications and problems to be resolved. Prog. Nat. Sci. 14 (11), 937–944. doi:10.1080/10020070412331344601
Keywords: bioremediation, earthworm, microbe, soil, agrochemical
Citation: Mishra CSK, Samal S and Samal RR (2022) Evaluating earthworms as candidates for remediating pesticide contaminated agricultural soil: A review. Front. Environ. Sci. 10:924480. doi: 10.3389/fenvs.2022.924480
Received: 20 April 2022; Accepted: 11 October 2022;
Published: 25 October 2022.
Edited by:
Jaswinder Singh, Khalsa College, Amritsar, IndiaReviewed by:
Swarndeep Hundal, Punjab Agricultural University, IndiaMuneer Ahmad Malla, Dr. Hari Singh Gour University, India
Copyright © 2022 Mishra, Samal and Samal. This is an open-access article distributed under the terms of the Creative Commons Attribution License (CC BY). The use, distribution or reproduction in other forums is permitted, provided the original author(s) and the copyright owner(s) are credited and that the original publication in this journal is cited, in accordance with accepted academic practice. No use, distribution or reproduction is permitted which does not comply with these terms.
*Correspondence: Suryasikha Samal, c3VyeWFzaWtoYS43NzdAZ21haWwuY29t