Microtopographic reconstruction improves soil erosion resistance and vegetation characteristics on the slopes of large dump sites in semi-arid areas
- 1Yinshanbeilu Grassland Eco-Hydrology National Observation and Research Station, China Institute of Water Resources and Hydropower Research, Beijing, China
- 2Soil and Water Conservation Laboratory, Ministry of Water Resources, Research Institute of Pastoral Water Resources Science, Hohhot, China
In view of the management of slopes in large mine dumps in semi-arid regions, this study explored different methods for controlling soil erosion and improving the microenvironment of the surface of such slopes. Focusing on microtopography modifications and vegetation measures on the slopes of a large mine dump in a semi-arid region, the soil erosion resistance of the slope was continuously monitored using installed observation plots. In addition, the characteristics of plant communities that thrived on the dump were investigated. The results indicate that the soil erosion resistance, soil organic matter content, and biodiversity increased to different degrees in the large mine dump that experienced microtopography modifications and implemented vegetation measures compared with those of slopes managed only by soil cover and planting. Compared with that in the control plot, under the same vegetation restoration measures, the macroaggregate content in the four plots that implemented microtopography modifications increased by 20%, 24%, 21%, and 30%; the soil erodibility (K-factor) decreased by 7.8%, 8.5%, 10%, and 10.7%; and the soil organic matter increased by 2%, 4.5%, 3.4%, and 4.7%. Microtopography modification consisting of U-shaped blocking boards and fixed rods embedded in the slope, combined with vegetation measures, resulted in a protective effect, which in turn induced the highest diversity, evenness, and richness index values of 2.35, 0.87, and 1.94, respectively. The study results revealed that a combination of microtopography modifications and vegetation measures can be used to achieve effective vegetation restoration, prevent soil erosion, and create different microhabitats, indicating that our approach is an effective way to control critical issues affecting large mine dumps in semi-arid regions.
1 Introduction
Mine dumps are a necessary by-product of surface mining (Monjezi et al., 2009; Franco-Sepúlveda et al., 2019; Shi et al., 2021), and the operation and management of such dumps have a profound impact on soil erosion, surface vegetation, and landscapes (Chen et al., 2019; Blake et al., 2018; Esmali Ouri and Kateb 2020). Owing to an increasing emphasis on ecological conservation in China, the number of studies on soil erosion control and vegetation restoration techniques associated with mine dumps has increased. Previous studies have shown that the biodiversity of mine dumps depends on vegetation restoration efforts (Feng et al., 2019; Tang et al., 2022). Suitable vegetation restoration techniques improve the nutrient contents and activity in the surface soil of mine dumps (Yuan et al., 2018; Raghunathan et al., 2021). Furthermore, soil aggregates are the basic structural units of soil. They are closely related to soil erosion, and their size and stability significantly affect soil erodibility. Thus, they are an important factor for evaluating the soil erosion resistance environment (Nie et al., 2018; De Laurentiis et al., 2019). Composition and stability of soil aggregates are the main indicators of soil structure and quality (Hao Wang et al., 2018; Lehmann et al., 2020). The formation of surface soil aggregates and improvement of erosion resistance of mine dumps also require appropriate vegetation restoration (Guo et al., 2020; Ke et al., 2021; Ma et al., 2022).
Large mine dump slopes represent soil erosion and vegetation restoration sites between platforms (flat sections (steps) and step slopes), whereby soil erosion and vegetation restoration have contrasting effects of intensification and suppression of soil erosion, respectively. Slopes are key areas of vegetation restoration and soil erosion control in large mine dumps, but vegetation restoration on the slopes of mine dumps is difficult to achieve using soil and vegetation cover alone. Furthermore, these slopes are important for the ecological conservation of mining areas, and it is important to overcome chronic soil and water conservation issues in large mine dumps.
To overcome these shortcomings in soil and water conservation in mine dumps, experts and scholars have carried out theoretical and practical analyses covering various topics, such as vegetation, engineering, and soil improvement measures to manage mining wastelands (Fischer et al., 2013; Chen et al., 2018; Luo et al., 2018). Previous studies have indicated that vegetation restoration and soil improvement measures can significantly control soil erosion in mining areas (Ouyang et al., 2018; Neuenkamp et al., 2019), but relying solely on the natural restoration of soil and vegetation through natural succession to shrubs and grasses requires 15–30 years, which can extend to more than 100 years in forest communities (Groninger et al., 2017; Mander et al., 2017; Chen et al., 2020). Poorly considered measures can increase the slope erosion more than that observed on bare land (Vinci et al., 2017; Guo et al., 2019). As a result, it is important to adopt appropriate measures and methods during the ecological restoration of large mine dumps. Some studies have shown that microtopography modifications affect rainfall infiltration, control soil erosion (Prosdocimi et al., 2017; Eltner et al., 2018; Zhang et al., 2018; Caviedes-Voullième et al., 2021), improve surface habitat, and promote water and soil conservation as well as vegetation restoration (Perreault et al., 2017; Yixia Wang et al., 2018; Wang et al., 2020; Mata-González et al., 2022). Following microtopography modifications, vegetation measures can significantly increase the content of nitrogen, phosphorus, potassium, and other organic matter in the damaged soil of mining areas, which further improves the physical and chemical properties of this soil, as well its erosion resistance (Orgiazzi and Panagos 2018; Zhao et al., 2018). To date, studies on the slope management of large mine dumps tended to focus on a single vegetation restoration model, and the selection of suitable vegetation species plays an important role in these studies. Only a few studies have analyzed the effects of microtopography modifications in conjunction with appropriate vegetation restoration on the regulation of soil erosion on the slopes of mine dumps in semi-arid areas.
Based on the shortcomings in slope management of large-scale mine dumps in semi-arid areas, in the present study, we analyzed the efficacy of microtopography modifications used in combination with appropriate vegetation measures and proposed viable soil and water conservation measures to control the chronic issues faced by large surface mine dumps in semi-arid areas. The results of the present study can be used to guide the ecological restoration of soil dumps and promote the sustainable exploitation of large surface mine dumps.
2 Materials and methods
2.1 Study area overview
Our study area was the southern mine dump of the East No. 2 Shengli Surface Coal Mine in the northeast city of Xilinhot in the Inner Mongolia Autonomous Region of China (44°3′29.68″–44°3′39.36″ N and 116°14′23.75″–116°14′36.03″ E; Figure 1). The soil and water conservation area is designated as the Xilinhot Plateau Soil Conservation Ecological Maintenance Area. The original terrain of the mine dump site was relatively flat, with an altitude range of 990–1,021 m. The relative height range of the mine dump was 100–120 m, with steps every 20 m. The dumping period was 10 years. The angle of slope repose was 33°. The steps had a longitudinal slope of 8°–10° and a transverse slope of 3°–5°. The test area was a slope containing bare patches. The average thickness of the surface layer of the slope was 20 cm. In terms of physical and chemical properties of the upper soil layer (0–20 cm), the bulk density was 1.53 g cm−3, soil particle size was mainly medium sand >0.25 mm, and soil quality was poor. Total nitrogen content in the 0–20-cm soil layer of the slope was 0.12 g kg−1; available potassium content was 0.08 g kg−1, available phosphorus content was 0.92 mg kg−1, organic matter content was 2.0 g kg−1, and pH was 8.47.
The study area was located in the hinterland of the Inner Mongolia Plateau, which is known to have a mid-temperate semi-arid climate. The mean annual precipitation was 289.2 mm, 69% of which occurred from June to August. The maximum 24 h rainfall was 85.3 mm in the past 10 years and 111.5 mm in the past 20 years. The mean annual evaporation was 1805.1 mm, which was six times higher than the mean annual precipitation. The annual dominant wind direction was southwest, mean annual wind speed was 3.5 m s−1, and number of strong wind days (≥17 m/s) was 58. The zonal soil in the study area was sandy loam, and the non-zonal soil was chestnut soil. The vegetation type was typical grassland vegetation, with Stipa krylovii and Leymus chinensis as the main constructive species in the region. The vegetation coverage was 35–50%.
2.2 Experiment installation
On the third step of the southern mine dump of the East No. 2 Shengli Surface Coal Mine, we chose the middle section, which had a uniform slope, to select five runoff plots. Plots 1, 2, 3, and 4 were microtopography modification plots, and Plot five was a control plot (see Table 1; Figure 2 for details). Each plot was laid out in sequence along the longitudinal direction of the slope, and 0.6 m wide brick walkways were created between the plots to allow observations and sampling. The size of each plot was 20 × 5 m.
The microtopography modification method of Plot one involved embedding straight blocking boards (plywood boards) on the slope surface, with blocking boards laid in a zigzag shape along the longitudinal direction of the plot. The straight blocking boards were 60 cm long, 60 cm wide, and 0.5 cm thick. The horizontal spacing of the straight blocking boards was 60 cm, and the vertical spacing was 120 cm. The blocking boards were inserted in pre-cut grooves perpendicular to the slope at a depth of 30 cm. After they were buried, we ensured that the edges of the blocking boards were levelled with the slope. The main function of the blocking boards was to reduce the downhill movement of surface soil so that plant seeds would be evenly distributed in the slope surface layer before the vegetation had an effect on soil and water conservation as well as to avoid bare patches during the process of vegetation restoration. Once the blocking boards were in place, the open space of the plot was planted with Caragana korshinskii from top to bottom, with the inter-plant spacing of 120 cm and row spacing of 120 cm. The planting holes had a depth of 5 cm and radius of 5 cm, with a semi-circular cross section. The rest of the open space of the plots was scattered with a mix of Medicago sativa and Elymus dahuricus in a mixing ratio of 1:1.
The microtopography modification method of Plot two was to embed straight blocking boards and insert fixed rods in the slope. The layout positions and method of the blocking boards and the specifications were the same as those in Plot 1. The fixed rods were sand willow sticks, with a diameter of 10 mm and length of 35 cm. They were wedged into the slope along the back of the blocking boards so that their tops were flush with the edge of the boards. Once the blocking boards and rods were installed, the plot was levelled.
The microtopography modification method applied in Plot three consisted of U-shaped blocking boards (also plywood boards) arranged in a zigzag shape across the entire plot. The U-shaped blocking boards were 60 cm in length, 60 cm in width, and 0.5 cm in thickness. The layout and method of installing the U-shaped blocking boards were the same as those in Plot 1. After the blocking boards were installed, the plot was levelled.
The microtopography modification method of Plot 4 consisted of embedding U-shaped blocking boards and fixed rods in the slope. The layout positions and method of the blocking boards and the specifications were the same as those in Plot 1. The blocking board specifications were the same as those in Plot 3. The material, length, and installation method of the fixed rods were the same as those in Plot 2. Once the blocking boards and the rods were installed, the plot was levelled.
Plot 5 was the control plot. No microtopography modifications were applied to the plot, and only shrubs and grasses were planted after the topsoil was restored, which is the most common restoration method used in mine dumps. The thickness of the soil cover and the method of planting shrubs and grasses were the same as those in Plot 1. The implementation of the methods in all five plots was completed on 16 May 2019. Notably, the vegetation selection and planting methods for all the plots were the same.
2.3 Data processing
2.3.1 Soil sample collection and analysis
In this study, only the surface layer (0–20 cm) of the mine dump, which was covered with surface soil, was sampled. Below 20 cm, the lithology consisted of a mixture of clay, shale, and coal gangue; therefore, no sampling was carried out below this depth. Soil sampling were taken at the end of the plant growth season (mid to late September) in the period between 2019 and 2021. In each plot, nine soil samples of the 0–20-cm soil were randomly collected (i.e., three samples from the upper, middle, and lower parts of the slope, respectively) using a soil drill. In each plot, the samples collected from the upper, middle, and lower parts were mixed respectively and analyzed in a laboratory. The undisturbed soil was segregated into portions with diameters of 5 cm based on their structure. Plants, fine roots, stones, and other debris were removed, and the samples were placed in a ventilated place to dry naturally. After being air-dried, the soil samples from each plot were mixed, sieved, and stored in Ziplock bags for indoor analysis.
The dry sieving method was used to sort the soil according to the particle size, so that the contents of soil aggregates could be measured at all levels. One air-dried soil sample (240 g) was dry sieved, and the weight of soil aggregates in the particle sizes of 0.5, 0.1–0.25, 0.05–0.1, and <0.05-mm was measured to calculate the weight proportions for each soil aggregate particle grade. Another air-dried soil sample (50 g) were wet sieved and analyzed using an aggregate analysis meter. The water-stable aggregate content of the 0.25–0.5, 0.1–0.25, 0.05–0.1, and <0.05-mm grades were measured. After drying in an oven at a constant temperature of 4°C, the samples were weighed to calculate the percentage of water-stable aggregates in the soil for each grade.
The air-dried soil samples were sieved through a 2 mm sieve, and the samples were ground before being sieved again. The content of soil organic matter was determined using the potassium dichromate-volumetric method (external heating method).
2.3.2 Calculation methods for soil erosion resistance index
The specific formulas for calculating the mean weight diameter (MWD; mm) of soil aggregates (calculated using wet sieve aggregate size) and soil erodibility K-factor (in the case of limited soil physical and chemical properties, only the geometric mean soil particle size was considered) (van Bavel 1950; Shirazi et al., 1988) were as follows:
where xi is the diameter of the aggregate at each particle grade level (mm), wi is the percentage of aggregate mass, and GMD is the geometric mean diameter of the soil aggregate.
2.3.3 Plant community survey
In mid-August 2021, three quadrats (1 × 1 m) were randomly selected from the upper, middle, and lower parts of the five plots, and the individual plant height and density were measured; additionally, the cover of each plant species in each quadrat was measured, and the plant species present in the quadrats were recorded (Zhang 2007).
Species diversity was analyzed using three indices: Shannon–Wiener index of diversity, Pielou’s evenness index, and Margalef’s richness index. The calculation formulas for the indexes are given below (Clobert et al., 2018):
where Pi is the relative importance value, that is, Pi = N/Ni; Ni is the importance value of species i; N is the sum of importance values of species in the surveyed quadrat; and S is the number of species in the surveyed quadrat.
The calculation method of importance value (I) was calculated using the following equation:
where RC is the relative cover, RF is the relative frequency, and RD is the relative density.
We analyzed the data using SPSS Statistics V22.0 (IBM. Corp., Armonk, NY, United States) and plotted the data using Microsoft Excel 2016 (Microsoft Corp., Redmond, WA, United States).
3 Results and analysis
3.1 Effects of microtopography modification on soil aggregate structure
As shown in Figure 3, in Plots 3 and 4, after 3 years of vegetation restoration, the content of aggregates larger than 2.0 mm constituted 30–40% of the soil, whereas that of aggregates larger than 2.0 mm in the other plots constituted only 15–20% of the soil. The microtopography modification method, consisting of U-shaped blocking boards and fixed rods (Plot 4), resulted in different degrees of increase in macroaggregates after vegetation restoration.
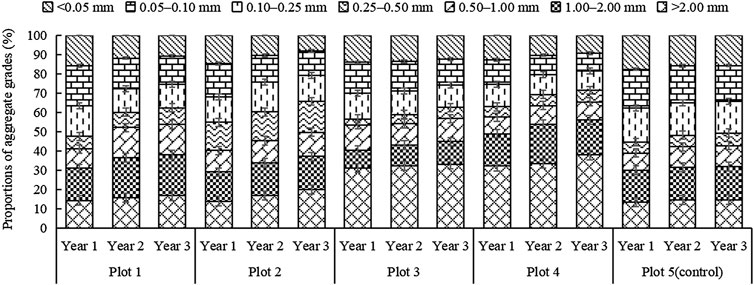
FIGURE 3. Changes in the soil aggregate grain sizes in the plots corresponding to different microtopography modifications.
As shown in Figure 3, the trends for the content aggregates larger than 0.25 mm in plot 1 to plot 4 were basically similar, increasing year by year in each plot; however, there was no obvious trend for aggregates of grade 0–0.25 mm. Following microtopography reconstruction on the slope surface of the mine dump site and the implementation of similar vegetation measures, the order of the plots, from large to small, in terms of the content of aggregates larger than 0.25 mm was: Plot 4 > Plot 3 > Plot 2 > Plot 1 > Plot 5 (control) in the first year; Plot 4 > Plot 2 > Plot 1 > Plot 3 > Plot 5 (control) in the second year; and Plot 4 > Plot 2 > Plot 3 > Plot 1 > Plot 5 (control) in the third year. The analysis revealed that during the same vegetation restoration periods, the plots with microtopography modifications had higher contents of aggregates larger than 0.25 mm than those of the control plot (Plot 5).
3.2 Effects of microtopography modifications on the MWD of soil aggregates
As shown in Figure 4A, the MWDs of the plots with microtopography modifications as well as that of the control plot increased more slowly with increasing vegetation restoration. During the same vegetation restoration periods, the soil MWDs of the plots managed by microtopography modifications were greater than that of Plot 5. This indicated that even though we adopted the same vegetation restoration measures, microtopography modifications were more effective in gradually increasing the amounts of soil aggregates. Under the same vegetation restoration measures, the order of the plots, from large to small, in terms of soil MWD was: Plot 4 > Plot 3 > Plot 2 = Plot 1 > Plot 5 (control) in the first year; Plot 4 > Plot 3 > Plot 1 > Plot 2 > Plot 5 (control) in the second year; and Plot 4 > Plot 3 > Plot 2 > Plot 1 > Plot 5 (control) in the third year. Even though the vegetation restoration measures were the same, in any given year, the U-shaped blocking boards and fixed rod modification method (Plot 4) resulted in the greatest MWD, followed by the plot that only used U-shaped blocking boards (Plot 3).
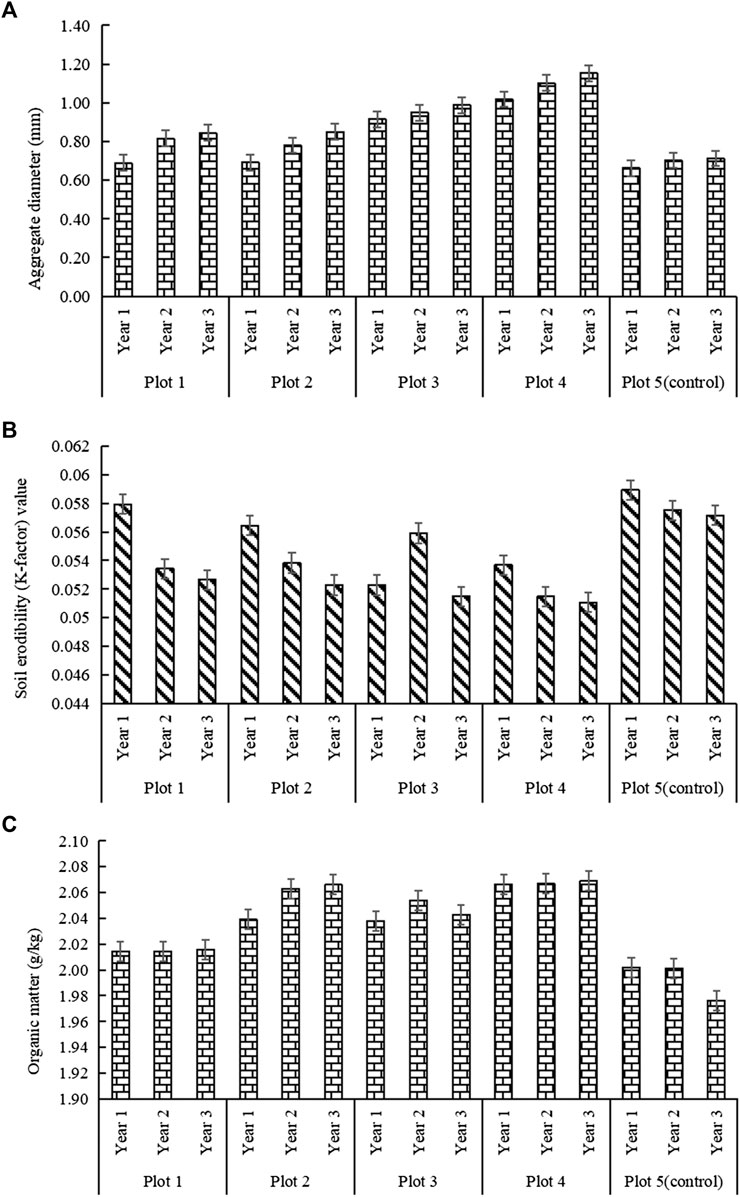
FIGURE 4. (A) Changes in mean weight diameter (MWD) of the soil aggregates in the four plots corresponding to different microtopography modifications. (B) Changes in soil erodibility in the plots corresponding to different microtopography modifications. (C) Changes in organic matter in the plots corresponding to different microtopography modifications.
3.3 Comparison of soil erodibility of plots under different microtopography modifications
Soil erodibility (K-factor) values are used as comprehensive indicators of soil resistance to water erosion. The higher the K-factor value, the weaker the soil erosion resistance and vice versa. As shown in Figure 4B, except for the K-factor value of Plot 3, which increased and then decreased with time (after vegetation restoration), the K-factor values of the other plots gradually decreased with time (after vegetation restoration). Following vegetation restoration at the same time point, the order of K-factor values of the plots, from small to large, with respect to various microtopography modifications was: Plot 3 < Plot 4 < Plot 2 < Plot 1 < Plot 5 (control) in the first year; Plot 4 < Plot 1 < Plot 2 < Plot 3 < Plot 5 (control) in the second year; and Plot 4 < Plot 3 < Plot 2 < Plot 1 < Plot 5 (control) in the third year. After the vegetation restoration measures were implemented, the K-factor value of Plot 4 decreased at the quickest rate, decreasing by 10% in the second year and 11% in the third year compared to that of the control plot. This indicated that with respect to the same vegetation restoration measures, the K-factor value of the plot with microtopography modifications consisting of U-shaped blocking boards and fixed rods decreased gradually with time, indicating an overall increase in soil erosion resistance.
3.4 Effects of microtopography modifications on soil organic matter content
Previous studies have reported that an increase in the soil organic matter content can promote soil cementation, strengthen the adsorption between particles, and increase the amount of macroaggregates, thereby improving the soil structure, aeration and water permeability, and erosion resistance (Šimanský et al., 2019; Chung et al., 2021; Liu et al., 2021). As shown in Figure 4C, with respect to the same vegetation restoration measures, the organic matter content in the surface soil of the plots that underwent microtopography modifications was higher than that in the control plot in the second and third years. With the exception of Plots 3 and 1, the surface soil organic matter content in the modified plots had the same change trend. After vegetation restoration, the organic matter content in the soil showed a slow increasing trend, consistent with the changes in soil erosion resistance. Under the same vegetation restoration measures, the order of organic matter content of the four plots, from large to small, under various microtopography modifications was: Plot 4 > Plot 2 > Plot 3 > Plot 1 > Plot 5 (control) in the first year; Plot 4 > Plot 2 > Plot 3 > Plot 1 > Plot 5 (control) in the second year; and Plot 4 > Plot 2 > Plot 3 > Plot 1 > Plot 5 (control) in the third year. After vegetation restoration, the surface organic matter content in the soil of Plot 4 increased slowly, up 3.2% in the first year, 3.2% in the second year, and 4.7% in the third year, compared with that in the control plot. After 3 years of vegetation recovery, the implementation of microtopography modifications increased the organic matter content in the slope surface soil from 2.0 to 2.06 g/kg. This may be related to the fact that microtopography modifications created a stable environment for plant growth. Vegetation restoration increased the soil organic matter content, and the organic matter content increased the soil resistance to erosion (Fonseca et al., 2017; Herrick et al., 2018; Guo et al., 2020).
3.5 Effects of microtopography modifications on plant community diversity
Previous studies have demonstrated that appropriate microtopography modifications can enhance the effects of vegetation restoration (García-Ávalos et al., 2018; Qiu et al., 2019; Wentzell et al., 2021). Furthermore, studies have shown that microtopography modifications are closely linked to vegetation restoration status and the corresponding soil and water conservation and biodiversity (Melnik et al., 2018; Tukiainen et al., 2019). In mid-August 2021, we conducted a plant community survey in the four plots wherein we implemented microtopography modifications, as well as in the control plot. In the five plots, 15 plant species were identified, and they belonged to five families and 14 genera. The plant species and their importance values (Pi) are shown in Table 2, and the results of the species diversity index analysis are shown in Table 3.
Species diversity is a comprehensive quantitative indicator of species richness and distribution uniformity in a habitat; thus, it is a good reflection of community composition (Tonkin et al., 2017; Hubbard et al., 2018). As shown in Table 3, compared to those in the control plot (Plot 5), the number of species, diversity (H′), evenness (J), and richness (R) indexes were all higher in the four plots where we implemented microtopography modifications. Among the four modified plots, Plot 4 had the highest diversity value (2.35), while Plots 2, 3, and 1 had the second, third, and fourth highest diversity values of 2.25, 2.20, and 2.10, respectively. In general, the evenness index indicates the evenness of plant distribution in a community. In the present study, Plot 4 had the highest evenness index, with a value of 0.87, followed by Plots 2, 3, and 1, with evenness index values of 0.83, 0.82, and 0.79, respectively. Generally, the species richness index indicates the total number of species in a community. In the present study, Plot 4 had the highest richness index value among the plots having microtopography modifications, with a value of 1.94, followed by Plots 2, 3, and 1, with richness index values of 1.89, 1.86, and 1.78, respectively. The Shannon–Wiener index of diversity objectively reflects the changes in species of a plant community, while taking into account any insufficiencies in species richness and evenness. Thus, it can indicate the diversity of a community more effectively than other indices. The plant community in Plot 4, which underwent microtopography modifications, had a higher species diversity than that of the other plots.
In Plot 4, we applied the microtopography modifications of U-shaped blocking boards and fixed rods. This combination prevented slope soil from slipping and additionally increased surface infiltration. The blocking boards embedded in the slope prevented the surface soil from separating from the slope, and the fixed rods reinforced the blocking boards. Thus, these modifications could provide a safe and stable environment for plant growth. They protected the slope before the vegetation measures took full effect and played an important role in improving the soil organic matter content as well as its erosion resistance. Therefore, Plot 4 had the highest plant community diversity, evenness, and richness index values.
In Plot 2, we applied straight blocking boards and fixed rods, which refined the space within the plot and ensured the initial evenness of plant species distribution. Although it prevented the surface soil from separating from the slope, the surveys revealed that it suffered more longitudinal erosion than Plot 4 did, which may have accounted for its diversity index value being lower than that of Plot 4. Plots 1 and 3 used single microtopography modification methods, and the surveys revealed that they contained bare patches ranging from 0.01 to 0.09 m2 in area. Owing to fewer species and more bare patches, the biodiversity of Plots one and three was lower than that of Plot 4. Plot 5 (control) only had soil cover and planted vegetation, with no protection methods for the surface soil on the slope. Therefore, the slope surface soil suffered severe slippage, indicating that the vegetation measures were not effective and did not stop slope erosion; thus, the bare patches on the slope increased over the duration of the study period. This plot had bare patches ranging from 0.5 to 1.0 m2 in area, which increased in size each year; additionally, compared to the other plots, this plot had fewer species and the least diverse plant community. By collating the surveys from the 3 years, we concluded that the plant species in the plots were the same in 2019, consisting primarily of artificially planted species, with minimal gradation and a single-layer structure. By 2021, the number of species in Plots one to four increased, and gradation appeared, indicating that microtopography modifications can allow vegetation restoration to take effect.
4 Discussion
4.1 Effects of microtopography modifications on soil erodibility
In the plots with microtopography modifications, the amount of macroaggregates increased every year. In 2021, compared with those in the control plot, aggregates larger than 0.25 mm increased by 20, 24, 21, and 30% in plots 1, 2, 3 and 4, respectively. Plot 4 exhibited the fastest increase in the amount of aggregates larger than 0.25 mm, followed by Plot 2. Some studies have reported that the water-stable aggregate content, particularly the content of aggregates larger than 0.25 mm, is one of the best indicators of soil erodibility (Hao Wang et al., 2018; Lehmann et al., 2020). The plots with microtopography modifications have different manifestations in terms of the three indicators of MWD, erosion resistance, and mine dump surface soil organic matter content. By 2021, compared with those in the control plot, the MWD in plots 1, 2, 3, and 4 increased by 15, 16, 27, and 38%; the soil erodibility (K-factor) values decreased by 7.8, 8.5, 10, and 10.7%, and the soil organic matter content increased by 2, 4.5, 3.4, and 4.7%, respectively. The results revealed that if the values surpassed these increments, the soil organic matter increased, soil erodibility decreased, and the MWD of soil aggregates increased. Previous studies have also demonstrated that soil organic matter is an important indicator of soil quality (Šimanský et al., 2019; Chung et al., 2021). It is also a binding material that forms soil aggregates and is important for increasing soil erosion resistance. Thus, the number and characteristics of soil aggregates reflects soil erosion resistance. This was consistent with the findings of Chung et al. (2021) and Šimanský et al. (2019).
In the present study, we proposed different combinations of microtopography modification materials and methods known to have good soil and water conservation effects. However, our study was limited to soil erosion resistance studied for a period of 3 years. Therefore, it lacks long-term observations of erosion prevention and control. Future studies should focus on the quantitative analysis of vegetation restoration and soil erosion on the slopes of mine dumps following the implementation of microtopography modifications. Compared with the control plot, plot 4 with the microtopography modification method of embedding U-shaped blocking boards and fixed rods in the slope was most effective at enhancing soil erosion resistance. Our study was based on the field survey of soil erosion resistance. However, we did not consider the process of the wind erosion; hence, increased observation of the amount of wind erosion is required in the future.
4.2 Effects of microtopography modifications on biodiversity
The implementation of microtopography modifications can create conditions for vegetation restoration and enhance biodiversity (Liu et al., 2020; Miller et al., 2021). In the present study, we investigated the characteristics of plant communities and showed that compared with the control plot, Plot 4 had the highest number of species (15), along with the highest Shannon–Weiner diversity (2.35), evenness (0.87), and richness indices (1.94). All four plots with microtopography modifications showed a higher plant diversity compared to that in the control (Plot 5). This indicated that single or combined microtopography modifications can create different microhabitats on the slopes of mine dumps. Before vegetation measures have an effect on soil and water conservation, modifications provide a safe and stable environment for plant growth, creating suitable conditions for species reproduction. This was consistent with the findings of Qiu et al. (2019) and Wentzell et al. (2021). In terms of vegetation restoration, we observed a close link between microtopography combinations and biodiversity. Nevertheless, as our investigation was limited to studying plant community characteristics in mid-August 2021, during a period of vigorous plant growth, it remains unclear whether microtopography modifications promote positive succession of plant communities during the other months of the year. The order of plots, from high to low, in terms of diversity, evenness, and richness were Plot 4 > Plot 2 > Plot 3 > Plot 1 > Plot 5 (control). This result was based on a survey of only a few plant community characteristics, and future studies should consider the dynamic changes in plant communities caused by single and combined microtopography modification methods.
4.3 The practicality of microtopography modification methods
The barrier plates and fixed piles used in the present study are all wooden materials. Following the application of soil and water conservation measures, such wooden materials begin to decay and are degraded into the soil. Before the plant measures come into effect, the slope surface material can be fixed to prevent the surface soil from separating from the slope and to provide a safe and stable environment for plant growth. In the project area, a large area of the slope with conventional plant measures emerged as an exposed open space. As the surface soil of the slope declined, which made the surface soil thinner, the slope vegetation cover decreased, and the erosion ditch density increased because there are no fixed measures for maintenance of the slope surface material, and consequently, the soil and water conservation treatment effects cannot be achieved. In the present study, the resources (1.2–1.5 fold higher) invested in the test plots were greater than those in the control plot (Plot 5). Moreover, a large amount of work was invested to address the problem of soil dump slope surface material separation; however, further studies are required to determine the quantitative transformation relationship between investment benefit and ecological benefit.
5 Conclusion
Using plot-based observation facilities, we studied the effects of microtopography modifications on the soil erosion resistance and biodiversity of a large mine dump in a semi-arid region. Our findings can serve as a reference for methodological support and production practices. The major conclusions of our study are explained below.
Soil erosion resistance and the biodiversity index under single or combined microtopography modifications, along with vegetation measures, on the slopes of the investigated large mine dump in a semi-arid region, were greater than those under soil cover and vegetation only. The combination of microtopography modifications and vegetation measures may prevented the slippage and separation of surface soil from the slope, creating a safe and stable micro-environment for plant growth. Thus, our study showed that a combination of microtopography modification measures and vegetation planting is effective at improving soil erosion and vegetation restoration along the slopes of large mine dumps.
Data availability statement
The original contributions presented in the study are included in the article/supplementary material; further inquiries can be directed to the corresponding author.
Author contributions
Conception: XT and JW. Analysis and interpretation of data: XT. Drafting of the manuscript: XT, RY, JW, LD, BC, HL, and NG.
Funding
This study was supported by the Special scientific research project of the China Institute of Water Resources and Hydropower Research (Grant number: MK 2019J11); central funds to guide local science and technology development program, “Analysis of the coupling mechanisms of water, salt, and nitrogen in drip irrigation under film in saline-alkali farmland in the Yellow River irrigation area, Inner Mongolia” (Grant number: 2021ZY0031).
Acknowledgments
The authors are indebted to the members of the Shengli Coal Mine who helped collect the samples.
Conflict of interest
The authors declare that the research was conducted in the absence of any commercial or financial relationships that could be construed as a potential conflict of interest.
Publisher’s note
All claims expressed in this article are solely those of the authors and do not necessarily represent those of their affiliated organizations, or those of the publisher, the editors and the reviewers. Any product that may be evaluated in this article, or claim that may be made by its manufacturer, is not guaranteed or endorsed by the publisher.
References
Blake, W. H., Rabinovich, A., Wynants, M., Kelly, C., Nasseri, M., Ngondya, I., et al. (2018). Soil erosion in East Africa: An interdisciplinary approach to realising pastoral land management change. Environ. Res. Lett. 13, 124014. doi:10.1088/1748-9326/aaea8b
Caviedes‐Voullième, D., Ahmadinia, E., and Hinz, C. (2021). Interactions of microtopography, slope and infiltration cause complex rainfall‐runoff behavior at the hillslope scale for single rainfall events. Water Resour. Res. 57, e2020. doi:10.1029/2020WR028127
Chen, H., Zhang, X., Abla, M., Lü, D., Yan, R., Ren, Q., et al. (2018). Effects of vegetation and rainfall types on surface runoff and soil erosion on steep slopes on the Loess Plateau, China. CATENA 170, 141–149. doi:10.1016/j.catena.2018.06.006
Chen, F., Yang, Y., Mi, J., Liu, R., Hou, H., Zhang, S., et al. (2019). Effects of vegetation pattern and spontaneous succession on remediation of potential toxic metal-polluted soil in mine dumps. Sustainability 11, 397. doi:10.3390/su11020397
Chen, X., Liang, Z., Zhang, Z., and Zhang, L. (2020). Effects of soil and water conservation measures on runoff and sediment yield in red soil slope farmland under natural rainfall. Sustainability 12, 3417. doi:10.3390/su12083417
Chung, H., Kim, S. H., and Nam, K. (2021). Application of microbially induced calcite precipitation to prevent soil loss by rainfall: Effect of particle size and organic matter content. J. Soils Sediments 21, 2744–2754. doi:10.1007/s11368-020-02757-2
Clobert, J., Chanzy, A., Le Galliard, J.-F., Chabbi, A., Greiveldinger, L., Caquet, T., et al. (2018). How to integrate experimental research approaches in ecological and environmental studies: AnaEE France as an example. Front. Ecol. Evol. 6, 43. doi:10.3389/fevo.2018.00043
De Laurentiis, V., Secchi, M., Bos, U., Horn, R., Laurent, A., Sala, S., et al. (2019). Soil quality index: Exploring options for a comprehensive assessment of land use impacts in LCA. J. Clean. Prod. 215, 63–74. doi:10.1016/j.jclepro.2018.12.238
Eltner, A., Maas, H. G., and Faust, D. (2018). Soil micro-topography change detection at hillslopes in fragile Mediterranean landscapes. Geoderma 313, 217–232. doi:10.1016/j.geoderma.2017.10.034
Esmali Ouri, A., and Kateb, F. (2020). Study of soil erosion potential using landscape measurements (case study: Sharif Beiglou watershed, Ardabil Province). Hydrogeomorphology 7, 145–164. doi:10.22034/HYD.2020.41552.1543
Feng, Y., Wang, J., Bai, Z., and Reading, L. (2019). Effects of surface coal mining and land reclamation on soil properties: A review. Earth. Sci. Rev. 191, 12–25. doi:10.1016/j.earscirev.2019.02.015
Fischer, L. K., Lippe, Mvd, Rillig, M. C., and Kowarik, I. (2013). Creating novel urban grasslands by reintroducing native species in wasteland vegetation. Biol. Conserv. 159, 119–126. doi:10.1016/j.biocon.2012.11.028
Fonseca, F., de Figueiredo, T., Nogueira, C., and Queirós, A. (2017). Effect of prescribed fire on soil properties and soil erosion in a Mediterranean mountain area. Geoderma 307, 172–180. doi:10.1016/j.geoderma.2017.06.018
Franco-Sepúlveda, G., Del Rio-Cuervo, J. C., and Pachón-Hernández, M. A. (2019). State of the art about metaheuristics and artificial neural networks applied to open pit mining. Resour. Policy 60, 125–133. doi:10.1016/j.resourpol.2018.12.013
García-Ávalos, S., Rodriguez-Caballero, E., Miralles, I., Luna, L., Domene, M. A., Solé-Benet, A., et al. (2018). Water harvesting techniques based on terrain modification enhance vegetation survival in dryland restoration. CATENA 167, 319–326. doi:10.1016/j.catena.2018.05.004
Groninger, J., Skousen, J., AngelAngel, P., Barton, C., Burger, J., and Zipper, C. (2017). Mine reclamation practices to enhance forest development through natural succession the forestry reclamation approach: Guide to successful reforestation of mined lands, in eds. M. B. Adams. Gen. Tech. Rep. NRS-169 (Newtown Square, PA: United States Department of Agriculture, Forest Service, Northern Research Station), 1–7. doi:10.1016/0169-2046(89)9022-4
Guo, M., Wang, W., Shi, Q., Chen, T., Kang, H., Li, J., et al. (2019). An experimental study on the effects of grass root density on gully headcut erosion in the gully region of China’s Loess Plateau. Land Degrad. Dev. 30, 2107–2125. doi:10.1002/ldr.3404
Guo, M., Wang, W., Wang, T., Wang, W., and Kang, H. (2020). Impacts of different vegetation restoration options on gully head soil resistance and soil erosion in loess tablelands. Earth Surf. Process. Landf. 45, 1038–1050. doi:10.1002/esp.4798
Hao Wang, H., Zhang, G. H., Li, N. N., Zhang, B., and Yang, H. (2018). Soil erodibility influenced by natural restoration time of abandoned farmland on the Loess Plateau of China. Geoderma 325, 18–27. doi:10.1016/j.geoderma.2018.03.037
Herrick, J. E., Weltz, M. A., Reeder, J. D., Schuman, C. E., and Simanton, J. R. (2018). “Rangeland soil erosion and soil quality: Role of soil resistance, resilience, and disturbance regime,” in Soil quality and soil erosion. Editor R. Lal (Boca Raton, FL: CRC Press), 209–233.
Hubbard, C. J., Brock, M. T., Van Diepen, L. T., Maignien, L., Ewers, B. E., Weinig, C., et al. (2018). The plant circadian clock influences rhizosphere community structure and function. ISME J. 12, 400–410. doi:10.1038/ismej.2017.172
Ke, W., Zhang, X., Zhu, F., Wu, H., Zhang, Y., Shi, Y., et al. (2021). Appropriate human intervention stimulates the development of microbial communities and soil formation at a long-term weathered bauxite residue disposal area. J. Hazard. Mat. 405, 124689. doi:10.1016/j.jhazmat.2020.124689
Lehmann, J., Bossio, D. A., Kögel-Knabner, I., and Rillig, M. C. (2020). The concept and future prospects of soil health. Nat. Rev. Earth Environ. 1, 544–553. doi:10.1038/s43017-020-0080-8
Liu, Y., Du, J., Xu, X., Kardol, P., and Hu, D. (2020). Microtopography-induced ecohydrological effects alter plant community structure. Geoderma 362, 114119. doi:10.1016/j.geoderma.2019.114119
Liu, B., Xie, Y. H., Tang, C. S., Pan, X-H., Jiang, N.-J., Singh, D. N., et al. (2021). Bio-mediated method for improving surface erosion resistance of clayey soils. Eng. Geol. 293, 106295. doi:10.1016/j.enggeo.2021.106295
Luo, C., Deng, Y., Inubushi, K., Liang, J., Zhu, S., Wei, Z., et al. (2018). Sludge biochar amendment and alfalfa revegetation improve soil physicochemical properties and increase diversity of soil microbes in soils from a rare Earth element mining wasteland. Int. J. Environ. Res. Public Health 15, 965. doi:10.3390/ijerph15050965
Ma, R., Hu, F., Xu, C., Liu, J., and Zhao, S. (2022). Response of soil aggregate stability and splash erosion to different breakdown mechanisms along natural vegetation restoration. CATENA 208, 105775. doi:10.1016/j.catena.2021.105775
Mander, M., Jewitt, G., Dini, J., Glenday, J., Blignaut, J., Hughes, C., et al. (2017). Modelling potential hydrological returns from investing in ecological infrastructure: Case studies from the Baviaanskloof-Tsitsikamma and uMngeni catchments, South Africa. Ecosyst. Serv. 27, 261–271. doi:10.1016/j.ecoser.2017.03.003
Mata-González, R., Averett, J. P., Abdallah, M. A. B., and Martin, D. W. (2022). Variations in groundwater level and microtopography influence desert plant communities in shallow aquifer areas. Environ. Manage. 69, 45–60. doi:10.1007/s00267-021-01526-2
Melnik, K., Landhäusser, S. M., and Devito, K. (2018). Role of microtopography in the expression of soil propagule banks on reclamation sites. Restor. Ecol. 26, S200–S210. doi:10.1111/rec.12587
Miller, V. S., Naeth, M. A., and Wilkinson, S. R. (2021). Micro topography, organic amendments and an erosion control product for reclamation of waste materials at an arctic diamond mine. Ecol. Eng. 172, 106399. doi:10.1016/j.ecoleng.2021.106399
Monjezi, M., Shahriar, K., Dehghani, H., and Samimi Namin, F. (2009). Environmental impact assessment of open pit mining in Iran. Environ. Geol. 58, 205–216. doi:10.1007/s00254-008-1509-4
Neuenkamp, L., Prober, S. M., Price, J. N., Zobel, M., and Standish, R. J. (2019). Benefits of mycorrhizal inoculation to ecological restoration depend on plant functional type, restoration context and time. Fungal Ecol. 40, 140–149. doi:10.1016/j.funeco.2018.05.004
Nie, X., Li, Z., Huang, J., Liu, L., Xiao, H., Liu, C., et al. (2018). Thermal stability of organic carbon in soil aggregates as affected by soil erosion and deposition. Soil Tillage Res. 175, 82–90. doi:10.1016/j.still.2017.08.010
Orgiazzi, A., and Panagos, P. (2018). Soil biodiversity and soil erosion: It is time to get married: Adding an earthworm factor to soil erosion modelling. Glob. Ecol. Biogeogr. 27, 1155–1167. doi:10.1111/geb.12782
Ouyang, W., Wu, Y., Hao, Z., Zhang, Q., Bu, Q., Gao, X., et al. (2018). Combined impacts of land use and soil property changes on soil erosion in a mollisol area under long-term agricultural development. Sci. Total Environ. 613–614, 798–809. doi:10.1016/j.scitotenv.2017.09.173
Perreault, L. M., Yager, E. M., and Aalto, R. (2017). Effects of gradient, distance, curvature and aspect on steep burned and unburned hillslope soil erosion and deposition. Earth Surf. Process. Landf. 42, 1033–1048. doi:10.1002/esp.4067
Prosdocimi, M., Burguet, M., Di Prima, S., Sofia, G., Terol, E., Rodrigo Comino, J. R., et al. (2017). Rainfall simulation and Structure-from-Motion photogrammetry for the analysis of soil water erosion in Mediterranean vineyards. Sci. Total Environ. 574, 204–215. doi:10.1016/j.scitotenv.2016.09.036
Qiu, D., Yan, J., Ma, X., Luo, M., Wang, Q., Cui, B., et al. (2019). Microtopographical modification by a herbivore facilitates the growth of a coastal saltmarsh plant. Mar. Pollut. Bull. 140, 431–442. doi:10.1016/j.marpolbul.2019.01.066
Raghunathan, K., Marathe, D., Singh, A., and Thawale, P. (2021). Organic waste amendments for restoration of physicochemical and biological productivity of mine spoil dump for sustainable development. Environ. Monit. Assess. 193, 599. doi:10.1007/s10661-021-09379-2
Shi, W., Wang, J., Li, X., Xu, Q., and Jiang, X. (2021). Multi-fractal characteristics of reconstructed landform and its relationship with soil erosion at a large opencast coal-mine in the loess area of China. Geomorphology 390, 107859. doi:10.1016/j.geomorph.2021.107859
Shirazi, M. A., Boersma, L., and Hart, J. W. (1988). A unifying quantitative analysis of soil texture: Improvement of precision and extension of scale. Soil Sci. Soc. Am. J. 52, 181–190. doi:10.2136/sssaj1988.03615995005200010032x
Šimanský, V., Juriga, M., Jonczak, J., Uzarowicz, Ł., and Stępień, W. (2019). How relationships between soil organic matter parameters and soil structure characteristics are affected by the long-term fertilization of a sandy soil. Geoderma 342, 75–84. doi:10.1016/j.geoderma.2019.02.020
Tang, J., Liang, J., Yang, Y., Zhang, S., Hou, H., Zhu, X., et al. (2022). Revealing the structure and composition of the restored vegetation cover in semi-arid mine dumps based on LiDAR and hyperspectral images. Remote Sens. (Basel). 14, 978. doi:10.3390/rs14040978
Tonkin, J. D., Bogan, M. T., Bonada, N., Rios-Touma, B., and Lytle, D. A. (2017). Seasonality and predictability shape temporal species diversity. Ecology 98, 1201–1216. doi:10.1002/ecy.1761
Tukiainen, H., Kiuttu, M., Kalliola, R., Alahuhta, J., and Hjort, J. (2019). Landforms contribute to plant biodiversity at alpha, beta and gamma levels. J. Biogeogr. 46, 1699–1710. doi:10.1111/jbi.13569
van Bavel, C. H. M. V. (1950). Mean weight-diameter of soil aggregates as a statistical index of Aggregation. Soil Sci. Soc. Am. J. 14, 20–23. doi:10.2136/sssaj1950.036159950014000C0005x
Vinci, A., Todisco, F., Brigante, R., Mannocchi, F., and Radicioni, F. (2017). A smartphone camera for the structure from motion reconstruction for measuring soil surface variations and soil loss due to erosion. Hydrol. Res. 48, 673–685. doi:10.2166/nh.2017.075
Wang, G., Lv, J., Han, G., Zhu, S., Liu, X., Wang, A., et al. (2020). Ecological restoration of degraded supratidal wetland based on microtopography modification: A case study in the Yellow River delta. Wetlands 40, 2659–2669. doi:10.1007/s13157-020-01351-z
Wentzell, B. M., DeVito, E. D., and Shebitz, D. J. (2021). Effects of restoration strategies on vegetation establishment in retired cranberry bogs. Plant Ecol. 222, 897–913. doi:10.1007/s11258-021-01150-4
Yixia Wang, Y., Ran, L., Fang, N., and Shi, Z. (2018). Aggregate stability and associated organic carbon and nitrogen as affected by soil erosion and vegetation rehabilitation on the Loess Plateau. CATENA 167, 257–265. doi:10.1016/j.catena.2018.05.005
Yuan, Y., Zhao, Z., Niu, S., Li, X., Wang, Y., Bai, Z., et al. (2018). Reclamation promotes the succession of the soil and vegetation in opencast coal mine: A case study from Robinia pseudoacacia reclaimed forests, pingshuo mine, China. CATENA 165, 72–79. doi:10.1016/j.catena.2018.01.025
Zhang, J. E. (2007). Common experimental research methods and techniques in ecology (in Chinese). Beijing, China: Chemical Industry Press.
Zhang, X., Hu, M., Guo, X., Yang, H., Zhang, Z., Zhang, K., et al. (2018). Effects of topographic factors on runoff and soil loss in Southwest China. CATENA 160, 394–402. doi:10.1016/j.catena.2017.10.013
Keywords: semi-arid region, mine dump, microtopography modification, soil erosion, species diversity
Citation: Tian X, Yin R, Wang J, Dong L, Cheng B, Liu H and Ge N (2022) Microtopographic reconstruction improves soil erosion resistance and vegetation characteristics on the slopes of large dump sites in semi-arid areas. Front. Environ. Sci. 10:930519. doi: 10.3389/fenvs.2022.930519
Received: 28 April 2022; Accepted: 11 July 2022;
Published: 08 August 2022.
Edited by:
Huawei Pi, Henan University, ChinaReviewed by:
Zhenhong Wang, Guizhou University, ChinaJinman Wang, China University of Geosciences, China
Copyright © 2022 Tian, Yin, Wang, Dong, Cheng, Liu and Ge. This is an open-access article distributed under the terms of the Creative Commons Attribution License (CC BY). The use, distribution or reproduction in other forums is permitted, provided the original author(s) and the copyright owner(s) are credited and that the original publication in this journal is cited, in accordance with accepted academic practice. No use, distribution or reproduction is permitted which does not comply with these terms.
*Correspondence: Jian Wang, 304406378@qq.com