Resource utilization of waste tailings: Simulated removal of nitrogen from secondary effluent by autotrophic denitrification based on pyrite tailings
- 1Engineering Research Center of Ministry of Education, Anhui Jianzhu University, Hefei, China
- 2Key Laboratory of Water Pollution Control and Wastewater Recycling of Anhui Province, Hefei, China
- 3School of Environmental and Energy Engineering, Anhui Jianzhu University, Hefei, China
- 4School of Mechanical and Electrical Engineering, Anhui Jianzhu University, Hefei, China
- 5Nanjing University Environmental Planning and Design Research Institute Group Co., Nanjing, China
- 6Anhui Magang Zhangzhuang Mining Co., Ltd., Luan, China
- 7Energy Saving Research Institute, Anhui Jianzhu University, Hefei, China
In this report, eco-friendly and low-cost pyrite tailings are utilized as denitrification materials, while pyrite and magnetite are used as the control experimental groups to explore the denitrification performance of pyrite tailings. The results show that the removal effect of pyrite tailings on NO3− is slightly lower than that of pyrite. However, the concentration of NH4+ decreased, and the effluent SO42- concentration was lower than that of pyrite. In addition, pyrite with different particle sizes is prepared by the ball milling method, and the effect of particle size on the denitrification performance of pyrite is studied. The results show that with the decrease in particle size, microorganisms are more likely to use S in tailings as electron donors, the denitrification rate is faster, and the denitrification effect was better at the same time.
Introduction
Lake eutrophication is a global water ecological environment problem (Hans W. Paerl, et al., 2015), and it is also one of the main challenges to water ecological environment governance and protection in China (Chen and Li. 2006). In recent years, lake eutrophication caused by excessive input of nitrogen and phosphorus has become increasingly prominent, seriously threatening the ecological security and drinking water security of lake basins (Farshad Shafiei. 2021). At present, although domestic sewage treatment has reached the drainage standard, there is no nitrogen and phosphorus environmental capacity for the eutrophic water (Chen. 2020). Therefore, with the development of cheap and efficient denitrification and dephosphorization materials and technology (Song. 2021), the municipal sewage treatment plant secondary effluent more strict denitrification and dephosphorization processes can ensure the ecological environment of water (Cao, et al., 2020).
Secondary effluent of municipal wastewater treatment plants is typical low C/N wastewater (Sun et al., 2019). The conventional heterotrophic denitrification process requires the addition of external carbon sources, which increases the treatment cost and complexity of operation (Fu, 2022). However, autotrophic denitrification does not require organic carbon sources and can use inorganic carbon and reducing sulfur compounds as electronic donors, which can reduce the operating cost of municipal wastewater treatment plants and effectively remove NO3− in secondary effluent with low treatment cost. According to different electron donors, autotrophic denitrification can be divided into hydrogen autotrophic denitrification, iron autotrophic denitrification, and sulfur autotrophic denitrification (Zhou et al., 2022). Sulfur autotrophic denitrification with elemental sulfur as the electron donor has a fast denitrification rate, but a large amount of reaction by-product SO42- will be produced during the reaction process, which affects its promotion in practical application (Jiaoyang, 2014). In recent years, pyrite (FeS2) has also been found to be an electron donor for chemical autotrophic denitrification (Pang, et al., 2020). Garcia-Gil et al. (Garcia-Gil, et al., 1993) found that adding FeS to the sediments of the Rhone Delta in France could change the denitrification rate and monitor the oxidation process of S2- in FeS. Haaijer et al. (Haaijer et al., 2007) reported that autotrophic denitrification based on iron sulfide can remove nitrate in freshwater system and compared the denitrification of two different iron sulfides (pyrite and ferrous sulfide) as substrates, proving that ferrous sulfide can remove nitrate more effectively under the action of denitrifying bacteria. Bosch et al. (Bosch et al., 2012) proposed that while thiobacillus denitrificans use sulfur in iron sulfide as an electron donor, they may also use iron in iron sulfide as an electron donor to reduce nitrate. Yang et al. (Zhang et al., 2020) also found that synthetic or natural pyrrhotite (FeS) autotrophic denitrification (PAD) had excellent simultaneous nitrogen and phosphorus removal performance. At present, many scholars have turned their attention toward the autotrophic denitrification technology using pyrite as the electron donor to remove NO3− in water (Kong, et al., 2015), or the autotrophic denitrification and heterotrophic denitrification combined with pyrite and elemental sulfur or other mineral materials to remove nitrogen and phosphorus in sewage (Wang, et al., 2019). However, pyrite is an important mineral resource, and if directly used in a large amount of water treatment, there is also a large economic cost. Mine tailings have become the most produced and stored solid waste in China. The total amount of metal mine tailings accounts for 34% of general industrial solid waste and is a major hazard and pollution source (Kong, et al., 2016). A large number of tailings are piled up in the tailings pond and cannot be used, resulting in a large amount of waste of resources. The accumulation of these wastes also seriously affects the ecological environment and threatens people’s health (Theo. 2021). Pyrite tailings, by-products after mining, contain the same effective components as pyrite, which can provide electrons for autotrophic denitrification, while tailings, as substrates for denitrification reaction, provide a new way for waste utilization of tailings (Tong, et al., 2021). Therefore, in this study, pyrite tailings were selected as autotrophic denitrifying electron donors, and pyrite and magnetite were used as control groups to explore the feasibility of removing nitrogen and phosphorus substances from secondary effluent from tailings. At the same time, this study obtained pyrite tailings with different particle size scales using the ball milling method and discussed the influence of particle size scales on their nitrogen and phosphorus removal performance.
Materials and methods
Materials
Raw pyrite and pyrite tailings were obtained from Longqiao Mine in Tongling, Anhui province, China, while magnetite tailings were collected from Zhangzhuang Mine in Huqiu, Anhui Province, China. Then, the tailings were grounded into particles with a size of 0.10–0.15 mm using a high-speed blender, washed in deionized water several times to clarify the supernatant, followed by ultrasonic shock for 1 h, and washed in deionized water again. Finally, the mineral particles were dried at 70°C for 6 h in the oven. Nitrogen removal reaction system with pyrite, pyrite tailings, and magnetite tailings as the substrate was denoted by PM, PT, and MT, respectively.
Preparation of materials
Preparation of pyrite tailings
The pyrite tailings were mixed with water at the mass ratio of 1:1, and the mixed solution was poured into the planetary ball mill and mixed with large beads (3 mm) and small beads (2 mm) at a ratio of 1:1. Three kinds of pyrite tailings with different particle sizes were prepared by different operation settings of the ball mill. The mixture was transferred into the beaker after the ball grinding and made to stand for half an hour, then the supernatant was collected and washed in deionized water several times to clarify, and then, the mineral particles were dried at 70°C.
Simulation of sewage quality
The secondary effluent of the laboratory simulated sewage plant was prepared with deionized water. The simulated sewage quality was configured according to the actual secondary effluent concentration of the sewage plant. The concentration of nitrate–nitrogen in the experimental sewage prepared by potassium nitrate was 15 mg/L; and the concentration of ammonia nitrogen prepared by ammonium chloride was 10 mg/L. Sodium bicarbonate was used as an autotrophic denitrification carbon source with a concentration of 90 mg/L.
Methods
A total of 800 ml synthetic sewage was added to a 1000 ml conical flask, and 30 g mineral particles and 5 ml cleaned sludge were added to the flask, respectively. After the rubber plug was fastened, the flask was placed in a constant temperature shaker, which continued to run at 150 r/min and 30°C. Samples were taken at 0, 12, 24, 36, 48, 60, 84, 108, and 132 h, respectively, and the concentrations of NO3−-N, NH4+-N, NO2−-N, and SO42− in the water were measured. Three parallel experiments were set up for each group.
Thirty grams of mineral particles with different particle sizes were weighed and placed in a 1000 ml conical flask, 800 ml simulated secondary effluent was added, and then 5 ml cleaned sludge was added. After the rubber plug was fastened, the particles were placed in a constant temperature shaker, which continued to run at 150 r/min and 30°C. Samples were taken at 0, 12, 24, 36, 48, 60, 84, 108, and 132 h, respectively, and the concentrations of NO3−-N, NH4+-N, NO2−-N, and SO42− in the water were measured. Three parallel experiments were set up.
Characterization
To analyze the structure and element composition of the three minerals, the micromorphology and the element composition of the three mineral particles were observed using scanning electron microscopy equipped with an energy dispersive spectrometer (SEM-EDS). The mineral particles mixed with water after drying were collected, and the mixed solution was slowly added to the laser particle size analyzer at a speed of 2200 r/min. The dried mineral particles were placed in an X-ray powder diffractometer (XRD) for crystal structure analysis, and the angle of diffraction (2θ) was set in the range of 10–80°. The dried mineral particles were mixed with deionized water, and the mineral surface potential was determined using the Zeta potential analyzer.
Water sample analysis was performed according to Water and Water Monitoring Analysis Method 4th Edition (2002). The specific indicators and analysis methods are shown in Table 1.
Results and discussions
Study on nitrogen removal from the secondary effluent by autotrophic denitrification of three minerals
Characterization of three minerals
SEM images and elemental mappings of PM, PT, and MT are shown in Figure 1. SEM images clearly indicated that PM has a smooth, crystalline surface with large particles, while PT has a rough, granular surface with small particles. Meanwhile, elemental mappings verified that the areas of S in PM and PT were significantly larger than that of Fe, further confirming the pyrite structure. In addition, the surface of MT was rough, granular, and larger, and the mineral contained less Fe, which was due to the fact that the tailings contained less Fe2O3.
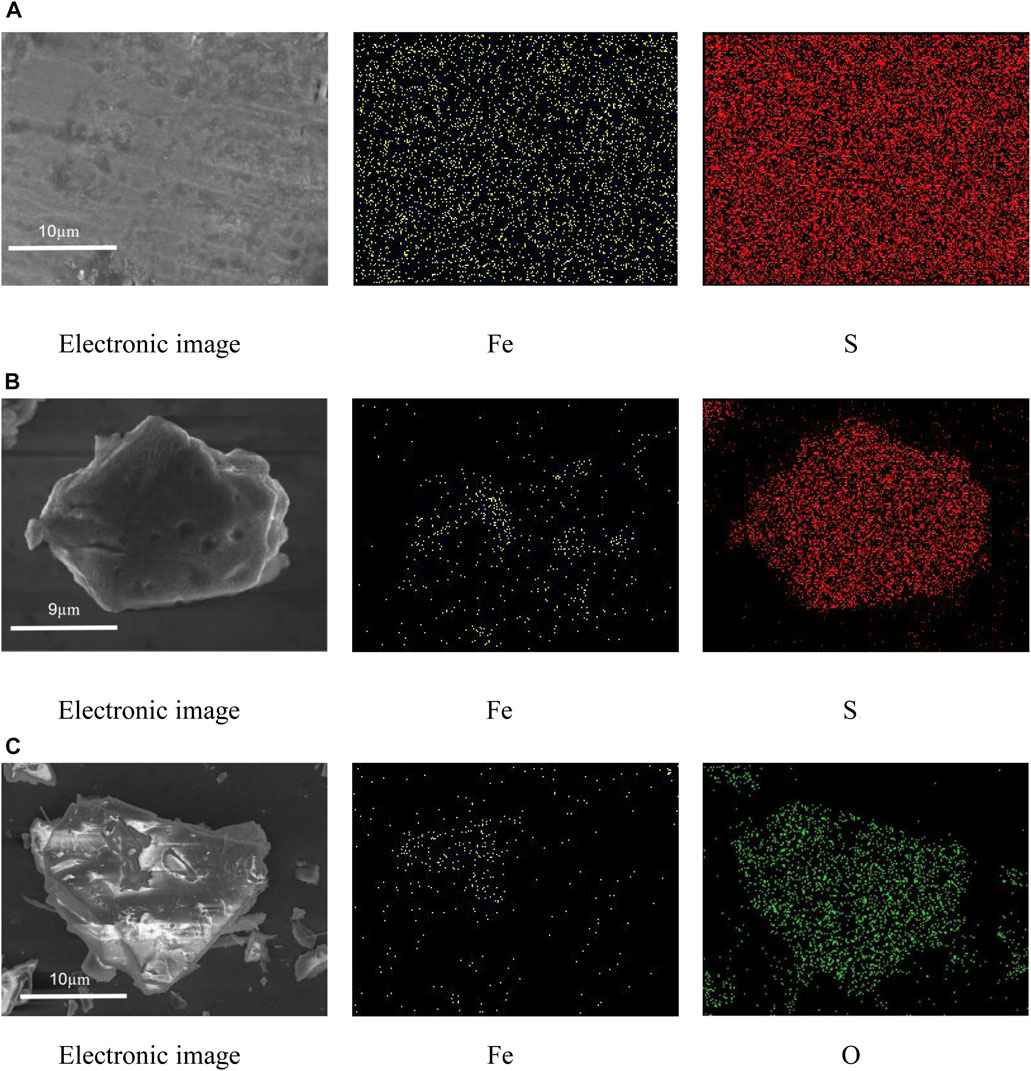
FIGURE 1. SEM images and elemental mappings of pyrite (A), pyrite tailings (B), and magnetite tailings (C).
XRD analysis of PT, PM, and MT was conducted for crystal structure analysis, and it can be seen from Figure 2 that all of the three minerals have obvious crystal structures. The diffraction peaks (2θ) of PT and PM at 28.6°, 33.1°, 37.2°, 40.9°, 47.6°, and 56.4° are assigned to the (111), (200), (210), (211), (220), and (311) crystal planes of FeS2 (JCPDS card no. 01-1295), respectively. Moreover, the diffraction peak position (2θ) of MT is 15.2°, 24.8°, 26.7°, 26.8°, 28.7°, 47.0°, 57.6°, and 50.4°, corresponding to the orthorhombic crystal structure; all the diffraction peaks were well matched with JCPDS card no: 89-2810.
Variation of effluent nitrogen and sulfate concentration
In order to explore the denitrification effect of pyrite tailings, we conducted a comparative study on the NO3−-N, NO2−-N, and NH4+-N removal performance of simulated sewage by three reaction systems of PM, MT, and PT. It can be seen from Figure 3A that the concentration of NO3−-N in PT, PM, and MT reaction systems first increased and then decreased at the initial stage of microbial growth and adaptation. During the 24–36 h period, the concentration of NO3−-N in the three systems reduced rapidly for a short time, which is probably due to the heterotrophic denitrification caused by the sewage with a low content of organic carbon. Subsequently, the concentration of NO3−-N in PT and PM shows a stable downward trend, while the concentration of NO3−-N in MT shows no significant change. After 132 h of reaction, the NO3−-N removal rates of PT, PM, and MT were 60.0, 69.0, and 36.94%, respectively. Figure 3B reveals the variation of effluent NH4+-N concentration over time in the reaction system. The effluent NH4+-N concentration of the three reaction systems shows a great difference. During the 24–36 h stage, the concentration of NH4+-N in PT, PM, and MT decreased from 3.10, 2.20, and 4.80 mg/L to 0.96, 0.76, and 3.50 mg/L, respectively. After 36h, the concentration of NH4+-N in the three reaction systems increased, which may result from the increase in NH4+ concentration caused by the death of heterotrophic microorganisms after the completion of heterotrophic denitrification. Furthermore, the autotrophic denitrification of PT and PM requires partial utilization of NH4+; so, the concentration of NH4+ in PT and PM gradually descended at the later stage of the reaction. Moreover, compared with PT, PM has a high content of FeS2 and more electron donors, and its demand for NH4+ is lower. Therefore, it can be seen from Figure 3B that PT has higher removal efficiency for NH4+-N. We simultaneously recorded the NO2−-N changes of three reaction systems. It can be observed from Figure 3C that NO2− was first accumulated and then reduced in all three systems, mainly because microorganisms reduced NO3− preferentially, and NO3− had an inhibitory effect on the synthesis and activity of NO2− reductase. However, at the end of the reaction, the accumulation of NO2−-N in PT and PM was 1.02 mg/L and 1.50 mg/L, respectively. The concentration of NO2−-N in MT was reduced to below the detection limit, and MT had the best removal effect on NO2−-N. Figure 3D reveals the concentration of SO42-- in the three systems. As one of the products in the process of sulfur autotrophic denitrification, the massive accumulation of SO42- is the limitation for the application of elemental sulfur autotrophic denitrification. In consequence, we monitored the concentration of SO42- in the three reaction systems, and the experimental results implied that the concentration of SO42- in MT did not change significantly over time, which was attributed to the fact that MT contained no sulfur element and could not undergo autotrophic denitrification of sulfur. On the contrary, the concentration of SO42- in PT and PM ascended gradually along with time, but the formation of SO42- in PT declined relatively, indicating that the use of pyrite tailings as nitrogen and phosphorus removal materials can reduce the amount of SO42-, without changing the water quality significantly.
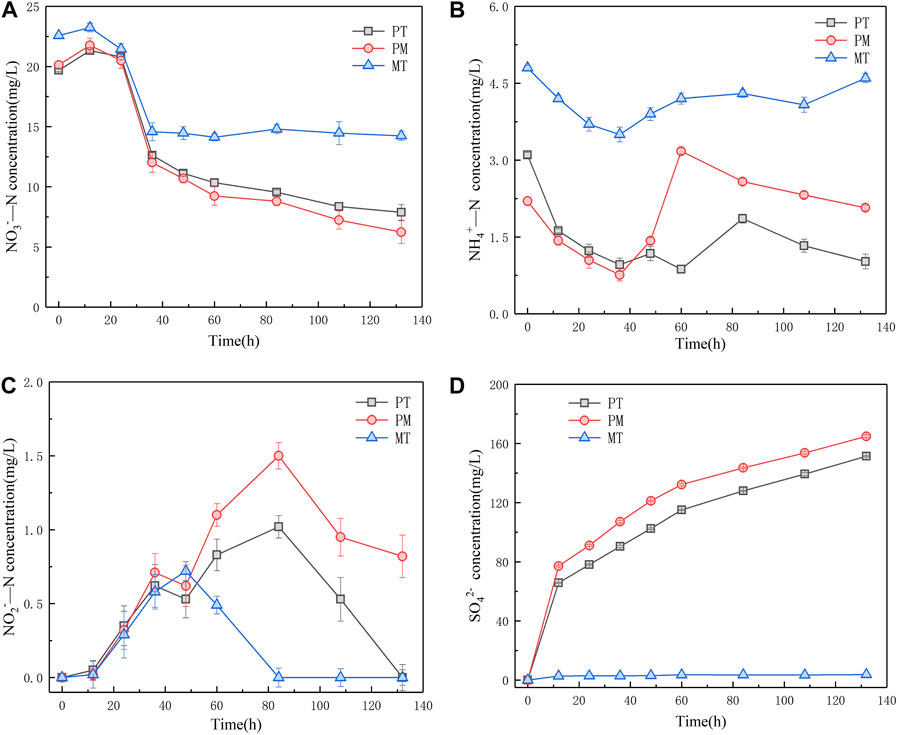
FIGURE 3. Variation of NO3−-N concentration (A), NH4+-N concentration (B), NO2−-N concentration (C), and SO42−concentration (D) in denitrification system of three minerals.
Influence of particle size on nitrogen removal
Characterization of three tailings with different particle sizes
Based on the fact that pyrite tailings have a certain nitrogen removal effect, we were then interested in studying the effect of particle size of tailings on nitrogen removal from effluent. By grinding the mineral for 30, 25, and 20 min, three tailing samples with different particle sizes were obtained (denoted as PT-1, PT-2, and PT-3, respectively). The particle size distribution is shown in Figure 4A, and the particle sizes (D50) of three kinds of minerals are 3.80, 7.59, and 2.89 μm, respectively. As the ball milling process is accompanied by high temperature and high pressure, the crystal structure of the minerals may be changed; so, XRD analysis was conducted on three kinds of minerals. It can be seen from Figure 4B that all of the minerals had obvious crystal structure, and the diffraction peaks (2θ) at 28.6°, 33.1°, 37.2°, 40.9°, 47.6°, and 56.4° correspond to the (111), (200), (210), (211), (220), and (311) crystal planes of FeS2, respectively. XRD results indicated that particle size had no influence on the crystal structure of the minerals. However, Zeta data (Figure 4C) demonstrated that the surface potential of the three groups of minerals was 4.34 mV, −0.12 mV, and −0.72 mV, respectively, indicating that the surface charge distribution of minerals would be changed during the mechanical stripping process of minerals by ball milling.
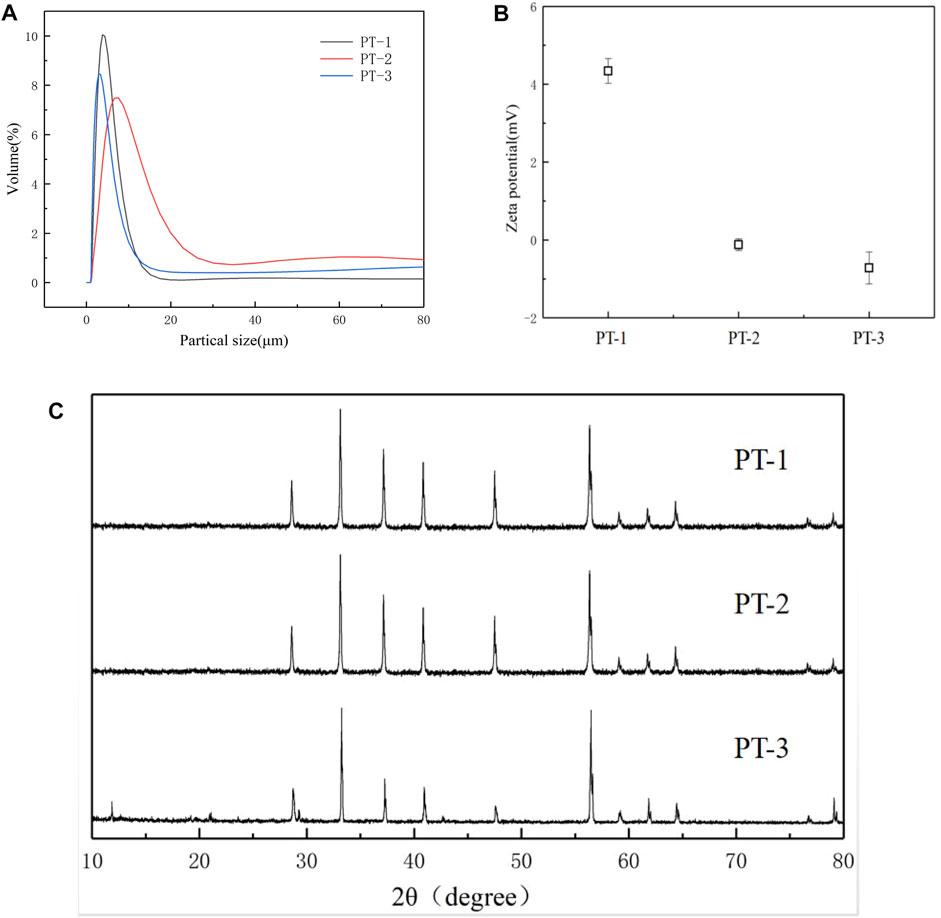
FIGURE 4. Particle size distribution spectrum (A) and XRD spectrum (B), Zeta potential (C) of pyrite tailings with different sizes.
Variation of effluent nitrogen and sulfate concentration
According to the XRD results, we confirmed that the change in particle size had no effect on the crystal structure of pyrite tailings. Therefore, we further investigated the feasibility and difference of NO3−-N, NO2−-N, and NH4+-N removal from secondary effluent of tailings with three different particle sizes. Variations in NO3−-N concentration of the three systems are shown in Figure 5A. At the initial stage of microbial growth and adaptation, the reaction rates of the three systems are relatively low. During 36–84 h period, the concentrations of NO3−-N in the three systems show a stable decreasing trend, and the reduction rates are 1.95, 2.40, and 2.43 mg/(L*d), respectively. The NO3−-N reduction rate decreased with the increase in pyrite particle size. At this stage, a heterotrophic denitrification reaction mainly occurred. The results indicate that the larger the pyrite tailing particles are, the more difficult it is to be utilized by autotrophic microorganisms, which leads to the slowdown of autotrophic denitrification rate and affects the NO3−-N removal in the water. As the reaction progressed, the death of some heterotrophic microorganisms was due to the inadaptability to the solution, releasing nitrate nitrogen and slightly increasing the concentration of NO3−-N in the solution. According to these results, we consider that particle size has no significant impact on the NO3−-N removal ability of pyrite tailing particles. Figure 5B displays that the change in NH4+-N concentration in the three systems is similar, and it rises at the initial stage of the reaction. Subsequently, the concentrations of NH4+-N in the three systems decrease because a part of NH4+ is consumed in the heterotrophic denitrification stage, while a part of NH4+ is also consumed by the growth of microorganisms themselves. At the end of the reaction, the concentrations of NH4+-N in the three systems rise, which is attributed to the fact that the dissimilatory nitrate reduction to ammonium (DNRA) process occurs in the autotrophic denitrification stage, which transforms NO3− into NH4+. In the system, the processes affecting the concentration of NH4+ mainly include the synthesis and utilization of microorganisms and the DNRA. It can be seen that the particle size of pyrite has no significant influence on the abovementioned processes. The accumulation amount of NO2−-N is shown in Figure 5C. All of the concentrations of NO2−-N in the three systems reach the maximum accumulation amount at 72 h, and there is little difference in NO3−-N concentration in the three systems at this time, indicating that the particle size of pyrite tailings did not affect the concentration of NO3−-N, which may inhibit the synthesis and activity of NO2− reductase in the denitrification system. As can be seen from Figure 5D, the accumulation amounts of SO42- in the three systems within 12–132 h are 93.46, 97.64, and 96.52 mg/L, respectively. With the decrease in particle size, the larger the contact surface between particles and air, the more easily S on the surface is oxidized into SO42-, leading to the higher concentration of SO42- in the initial reaction. Therefore, at the end of the experiment, more SO42- particles were produced.
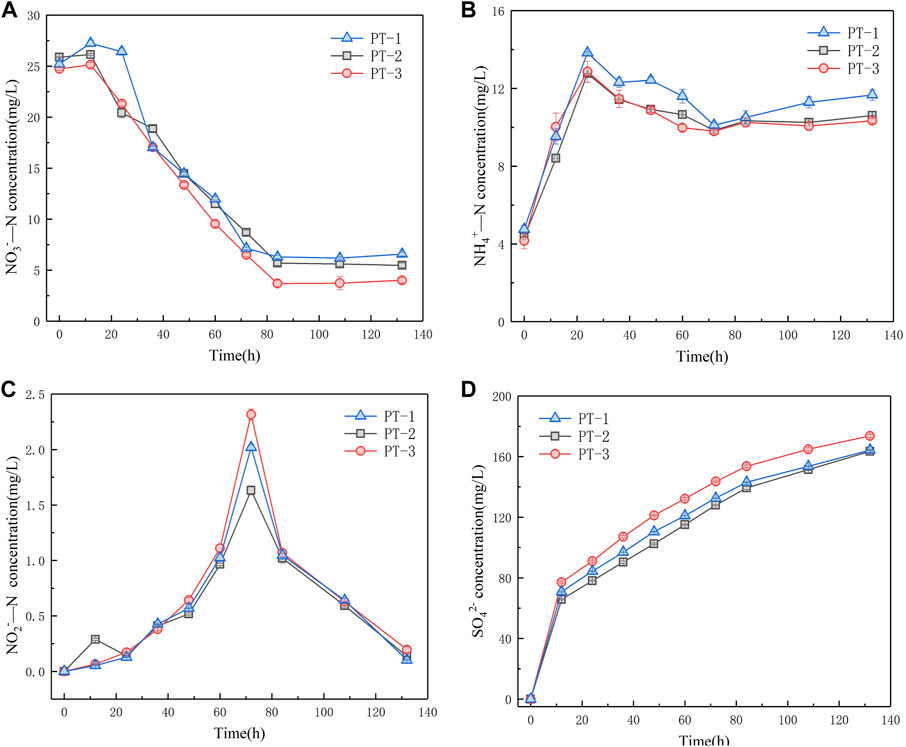
FIGURE 5. Variation of NO3−-N concentration (A), NH4+-N concentration (B), NO2−-Nconcentration (C), and SO42−concentration (D) in three granular denitrification systems of pyrite tailings.
Conclusion
In conclusion, we compare the denitrification effects of three kinds of mineral materials. The results show that magnetite had a limited ability to remove nitrogen in water and mainly depended on heterotrophic denitrification. Pyrite has the best effect on NO3− removal in water, but there is still a certain amount of NO2− in water after the reaction, and the concentration of NH4+ in water fluctuates within a certain range, which is almost the same as the initial value. However, the NO3− removal effect of pyrite tailings is slightly worse than that of pyrite, but the overall NH4+ concentration in the reaction process shows a downward trend, and the effluent SO42- concentration is lower than that of the pyrite experimental group. Therefore, in view of the economic benefits of pyrite tailings, it has greater potential in the application of denitrification and nitrogen removal in wastewater. In addition, pyrite tailings with different particle sizes are prepared using the ball milling method to study the effect of particle sizes on nitrogen removal. Through XRD and Zeta potential analysis, it can be seen that the main composition of the pyrite tailings after ball milling process does not change except particle size. With the decrease in particle size, microorganisms are more likely to use S in tailings as electron donors, and the denitrification rate will be faster and the denitrification effect will be better at the same time.
Data availability statement
The original contributions presented in the study are included in the article/Supplementary Material, and further inquiries can be directed to the corresponding author.
Author contributions
Conceptualization: JM and SZ; formal analysis: JM, MH, HC, CC, YQ, YC, TW, WW, BS, and SZ; funding acquisition: JM and BS; investigation: MH, HC, CC, YQ, YC, TW, WW, BS, and SZ; methodology: JM, MH, HC, CC, YQ, YC, TW, WW, XW, and SZ; project administration: SZ; validation: SZ; visualization: JM; writing—original draft, JM, MH, and XW; and writing—review and editing, HC, CC, YQ, YC, TW, WW, and BS.
Funding
This research was supported by the Project of the National Key Research and Development Program (2019YFC0408505); the National Natural Science Foundation of China (52103104); the Scientific Research Start-up Foundation for Introduction of Talent, Anhui Jianzhu University (2020QDZ30); the Natural Science Research Project of the Higher Education Institutions of Anhui Province (KJ 2021A0616); the Fujian Provincial Key Laboratory of Fire Retardant Materials, College of Materials, Xiamen University (FH202103); the 2021 National Innovation Training Program for College Students (202110878024); and the 2021 National Innovation Training Program for College Students (202210878026).
Conflict of interest
XW was employed by the company Nanjing University Environmental Planning and Design Research Institute Group Co., and WW was employed by the company Anhui Magang Zhangzhuang Mining Co., Ltd.
The remaining authors declare that the research was conducted in the absence of any commercial or financial relationships that could be construed as a potential conflict of interest.
Publisher’s note
All claims expressed in this article are solely those of the authors and do not necessarily represent those of their affiliated organizations, or those of the publisher, the editors, and the reviewers. Any product that may be evaluated in this article, or claim that may be made by its manufacturer, is not guaranteed or endorsed by the publisher.
References
Bosch, J., Lee, K. Y., Jordan, G., Kim, K. W., and Meckenstock, R. U. (2012). Anaerobic, nitrate-dependent oxidation of pyrite nanoparticles by thiobacillus denitrificans. Environ. Sci. Technol. 46 (4), 2095–2101. doi:10.1021/es2022329
Cao, Shenbin, Du, R., Peng, Y., Li, B., and Wang, S. (2020). Corrigendum to “Novel two stage partial denitrification (PD)-Anammox process for tertiary nitrogen removal from low carbon/nitrogen (C/N) municipal sewage” [Chem. Eng. J. 362 (2019) 107–115]. Chem. Eng. J. 395, 124810. doi:10.1016/j.cej.2020.124810
Chen, Jing. (2020). Research on technical problems of urban domestic sewage treatment under environmental protection form. IOP Conf. Ser. Earth Environ. Sci. 450, 012066. doi:10.1088/1755-1315/450/1/012066
Chen, Xiaoying, and Li, Shijie (2006). An analysis on the evolvement processes of lake eutrophication and their characteristics of the typical lakes in the middle and lower reaches of Yangtze River. Chin. Sci. Bull. 51 (13), 1603–1613. doi:10.1007/s11434-006-2005-4
Fu, X. (2022). Application of external carbon source in heterotrophic denitrification of domestic sewage: A review. Sci. total Environ., 817. doi:10.1016/J.SCITOTENV.2022.153061
Garcia-Gil, L. J., and Golterman, H. (1993). Kinetics of FeS-mediated denitrification in sediments from the Camargue (Rhone delta, southern France). FEMS Microbiol. Ecol. 13 (2), 85–91. doi:10.1111/j.1574-6941.1993.tb00054.x
Haaijer, S. C. M., Lamers, L. P. M., Smolders, A. J. P., Jetten, M. S. M., and Op den Camp, H. J. M. (2007). Iron sulfide and pyrite as potential electron donors for microbial nitrate reduction in freshwater wetlands. Geomicrobiol. J. 24 (5), 391–401. doi:10.1080/01490450701436489
Jiaoyang, P. (2014). Pyrite-based autotrophic denitrification for remediation of nitrate contaminated groundwater. Bioresour. Technol. 173, 113. doi:10.1016/j.biortech.2014.09.092
Kong, Zhe, Li, L., Feng, C., Chen, N., Dong, S., and Hu, W. (2015). Soil infiltration bioreactor incorporated with pyrite-based (mixotrophic) denitrification for domestic wastewater treatment. Bioresour. Technol. 187, 14–22. doi:10.1016/j.biortech.2015.03.052
Kong, Zhe, Li, L., Feng, C., Dong, S., and Chen, N. (2016). Comparative investigation on integrated vertical-flow biofilters applying sulfur-based and pyrite-based autotrophic denitrification for domestic wastewater treatment. Bioresour. Technol. 211, 125–135. doi:10.1016/j.biortech.2016.03.083
Paerl, Hans W., Xu, H., Hall, N. S., Rossignol, K. L., Joyner, A. R., Zhu, G., et al. (2015). Nutrient limitation dynamics examined on a multi-annual scale in lake taihu, China: Implications for controlling eutrophication and harmful algal blooms. J. Freshw. Ecol. 30, 5–24. doi:10.1080/02705060.2014.994047
Pang, Y., and Wang, J. (2020). Insight into the mechanism of chemoautotrophic denitrification using pyrite (FeS2) as electron donor. Bioresour. Technol. 318, 124105. doi:10.1016/j.biortech.2020.124105
Shafiei, Farshad (2021). Nutrient mass balance of a large riverine reservoir in the context of water residence time variability. Environ. Sci. Pollut. Res. 28, 39082–39100. doi:10.1007/S11356-021-13297-8
Song, Tao (2021). A review of research progress of heterotrophic nitrification and aerobic denitrification microorganisms (HNADMs). Sci. Total Environ. 801, 149319. doi:10.1016/J.SCITOTENV.2021.149319
Sun, S., Lin, H., Lin, J., Quan, Z., Zhang, P., and Ma, R. (2019). Underground sewage treatment plant: A summary and discussion on the current status and development prospects. Water Sci. Technol. 80, 1601–1611. doi:10.2166/wst.2019.429
Theo, Henckens (2021). Scarce mineral resources: Extraction, consumption and limits of sustainability. Resour. Conserv. Recycl. 169, 105511. doi:10.1016/J.RESCONREC.2021.105511
Tong, Liu, Hu, Y., Chen, N., He, Q., and Feng, C. (2021). High redox potential promotes oxidation of pyrite under neutral conditions: Implications for optimizing pyrite autotrophic denitrification. J. Hazard. Mater. 416, 125844. doi:10.1016/J.JHAZMAT.2021.125844
Wang, Wei, Wei, D., Li, F., Zhang, Y., and Li, R. (2019). Sulfur-siderite autotrophic denitrification system for simultaneous nitrate and phosphate removal: From feasibility to pilot experiments. Water Res. 160, 52–59. doi:10.1016/j.watres.2019.05.054
Zhang, Yang, Yu, J., Wu, Y., Li, M., Zhao, Y., Zhu, H., et al. (2020). Efficient production of chemicals from microorganism by metabolic engineering and synthetic biology. Chin. J. Chem. Eng. 13, 14–28. doi:10.1016/J.CJCHE.2020.12.014
Keywords: pyrite tailings, denitrification, ball milling, secondary effluent, resource utilization
Citation: Mao J, He M, Qin Y, Chen Y, Wang X, Che H, Cheng C, Wang T, Wang W, Sun B and Zhu S (2022) Resource utilization of waste tailings: Simulated removal of nitrogen from secondary effluent by autotrophic denitrification based on pyrite tailings. Front. Environ. Sci. 10:949618. doi: 10.3389/fenvs.2022.949618
Received: 21 May 2022; Accepted: 29 June 2022;
Published: 05 August 2022.
Edited by:
Shengsen Wang, Yangzhou University, ChinaReviewed by:
Chuan Chen, Harbin Institute of Technology, ChinaLingzhao Kong, Chinese Academy of Sciences (CAS), China
Copyright © 2022 Mao, He, Qin, Chen, Wang, Che, Cheng, Wang, Wang, Sun and Zhu. This is an open-access article distributed under the terms of the Creative Commons Attribution License (CC BY). The use, distribution or reproduction in other forums is permitted, provided the original author(s) and the copyright owner(s) are credited and that the original publication in this journal is cited, in accordance with accepted academic practice. No use, distribution or reproduction is permitted which does not comply with these terms.
*Correspondence: Shuguang Zhu, zhushuguang@ahjzu.edu.cn