Diversified crop rotations and organic amendments as strategies for increasing soil carbon storage and stabilisation in UK arable systems
- 1School of Natural and Environmental Sciences, Newcastle University, Newcastle upon Tyne, United Kingdom
- 2ITAP, INRAE, Institut Agro, Université de Montpellier, Montpellier, France
- 3Organic Research Centre, Trent Lodge, United Kingdom
Adaptations in crop rotation with the inclusion of temporary grass-clover leys and organic amendments, have been promoted as effective ways to improve soil carbon (C) sequestration and mitigate climate change in agricultural systems. However, there are still a lot of uncertainties related to i) the combined effects of different crop rotations and different fertilisation sources, e.g., organic amendments, on soil C stocks; and ii) their potential effect on C stabilisation. The objective of this study was to evaluate the effect of different arable crop rotations with varying degrees of diversity in crop type and lengths of grass-clover ley periods and fertilisation sources on soil C stocks and C stabilisation down to 0.60 m soil depth. This was investigated in a long-term factorial field experiment-combining different crop rotation (cereal-intensive conventional vs. diversified legume-intensive organic) with different lengths of grass-clover ley periods (2 vs. 3 years), fertilisation sources (mineral vs. compost), and years (samples taken at the beginning and at the last year of one complete cycle of rotation; 8 years apart)-to explore their individual and interactive effect on soil C stock and C stabilisation at two soil depths (0–0.30 and 0.30–0.60 m). Soil C stabilisation was assessed using a unique combination of three different techniques: physical fractionation for separation of C associated to organic and mineral fractions, thermal analysis combined with differential scanning calorimetry and a quadrupole mass spectrometry (TG-DSC-QMS) for physical-chemical aspects, and pyrolysis coupled with gas chromatography-mass spectrometry (Py-GC/MS) for molecular structural information. The findings showed higher soil C stocks under the diversified organic rotation with 3 years of grass-clover ley period at both soil depths, regardless of the fertilisation source or sampling year. However, the organic rotation seemed to deliver stable soil C stocks only in the subsoil layer. Compost fertilisation, in turn, increased topsoil C stocks between the two sample dates under both rotations, and it appears to be stable. These results suggested that combining a diversified organic rotation with 3 years grass-clover ley with compost fertilisation could be one way for agricultural systems to deliver stable soil C sequestration.
1 Introduction
Accumulation of soil organic carbon (SOC) has been highlighted as a biological approach to draw down atmospheric CO2 and reduce associated climate change effects (Paustian et al., 2016). Grasslands are frequently proposed as important ecosystems for soil carbon (C) cycling and consequently their storage (Bai and Cotrufo, 2022), while agricultural systems have been deemed C sources (Lal, 2004). In this sense, the inclusion of temporary grass-clover leys in a more diverse crop rotation, and other soil management practices, e.g., organic amendments, have been promoted as strategies for agricultural systems to increase soil organic matter (SOM) levels and improving soil quality aspects that foster SOC accumulation mechanisms (Zani et al., 2021; 2022; Guest et al., 2022). In addition to potential SOC benefits, diversified crop rotation, ley periods, and organic amendments are also related to other functions and ecosystem services that are essential for achieving sustainable management of agricultural soils, including but not limited to, high-quality crops, water resources, and nutrient cycling, among others (Tuomisto et al., 2012; Chen et al., 2022; Zhao et al., 2022; Zhu et al., 2022). While previous studies have confirmed that higher SOM inputs result in higher SOC stocks, further evidence is needed to understand the relative impacts of rotational crop-grass-clover leys and fertilisation strategies and especially how these practices might affect SOM quality, i.e., its composition and C stability.
Soil C stability can be simply defined as the resistance of SOC to decomposition. The decomposition of SOC is affected by the nature and composition (chemical and physical) of the input material, soil properties (e.g., physical), biological activities, and environmental conditions, as well as the quantity of the inputs to the given ecosystem (Dixon et al., 1994; Trumbore, 1997). The resistance of SOC to decomposition can be controlled by various biological, physicochemical and structural factors including its disconnection from microbes, soil aggregation and physical protection as well as chemical recalcitrance, where the SOC-mineral association is considered a significant factor controlling stability (Lützow et al., 2006; Schmidt et al., 2011; Basile-Doelsch et al., 2020). Overall, it has been suggested that if SOM consists of easily degradable material, e.g., due to its low C:N ratio, its decomposition rate is high because microorganisms decompose it relatively rapidly, while the opposite holds true for SOM with higher C:N ratio (excess of carbon) (Powlson et al., 2011; Li et al., 2018). This is, of course, also dependent on several other factors such as temperature, soil moisture, drying-wetting cycles, aeration, etc (Lal et al., 2015). Different crop rotations with different lengths of grass-clover leys and fertilisation sources are expected to show variation in these factors, as well as the quantity and quality of inputs, i.e., the amount of organic materials that will become SOM, and its composition. Such change can hence have a consequential effect on losses of SOM (through decomposition processes) and consequently SOC stocks and C stabilisation (Lal et al., 2015). Understanding how management practices affect SOM composition and proportions of SOC within pools with differing stability is crucial as this will help to design sustainable farming systems in the future which build stocks of soil C that are stable and permanent.
Several methods have been developed to assess the C content, composition and stability within SOM. Examples of analytical techniques used include i) physical fractionation of SOM into organic and mineral-associated fractions (Christensen, 1992), ii) thermogravimetry-differential scanning calorimetry coupled with quadrupole mass spectrometry (TG-DSC-QMS) (Lopez-Capel et al., 2005a; Fernández et al., 2012), and iii) pyrolysis coupled with gas chromatography-mass spectrometry (Py-GC-MS) (Mason et al., 2012; Abbott et al., 2013). The quantification of organic and mineral-associated fractions through physical fractionation of the SOM is used for understanding soil C dynamics, turnover and stability (Christensen, 1992). In this particular approach, SOM fractions can be associated with either a cellulosic material (composed of polysaccharides) or with a lignin-like material (composed of a mixture of aromatic, cross-linked phenolic C compounds) (Dell’Abate et al., 2002). Particulate organic matter fractions (POM) (i.e., the more labile available component for decomposition) represents the former, while the latter is composed of a more refractory (resistant to decomposition) material characterised by mineral-associated organic matter (Manning et al., 2005). Thermal analytical techniques (i.e., TG-DSC-QMS), in turn, involve programmed temperature change to monitor physical and/or chemical properties of a sample (Langier-Kuźniarowa, 2002). In this sense, TG-DSC-QMS can define SOM stability as a function of its bulk chemical composition and the degree of humification and mineral association of the SOM (Plante et al., 2009). As for the fractionation approach, this technique can provide insight into the proportions of active and more stable SOM components (Lopez-Capel et al., 2005b). Coupling TG-DSC to a QMS allows the chemical identification, characterisation and proportions of major evolved gas species from the target sample (Lopez-Capel et al., 2006). Variability in shape, area, and temperature of TG-DSC-QMS traces can reveal differences in thermal stability and chemical structure of the sample. As stated by Manning et al. (2005), this technique allows the determination of all the C present within a sample in a single heating analysis. Lastly, the use of Py-GC-MS can provide detailed molecular structural information, which is not provided by the other two techniques, in a simple and rapid manner. It aims to degrade macromolecules into small fragments (relative to the large macromolecules) and simultaneously identify structural information (Meier and Faix, 1992; Leinweber and Schulten, 1993).
In this context, in order to fully understand the potential for crop rotations with different lengths of grass-clover leys and fertilisation sources in delivering SOC accumulation, which is potentially stable and persistent, qualitative (size separation of SOM into fractions and chemical composition of SOM) and quantitative (SOC stocks) data must be investigated. Combining qualitative and quantitative data can provide novel insights about the composition of SOC stocks that might control stability and are still unknown. It could also help to elucidate the largely unknown processes in subsoil layers (i.e., > 0.30 m depth), which might represent more stable and long SOC turnovers as a result of reduced microbial activity, suboptimal environmental conditions, energy scarcity and less accessibility to the SOM (Rumpel and Kögel-Knabner, 2010). Until now, there have not been any investigations of SOM composition and C stability that compare crop rotation with different lengths of grass-clover leys and fertilisation sources that include both qualitative and quantitative data, specifically for long-term experiments (i.e., potential temporal changes) and subsoil layers.
The objectives of this study were to i) assess SOC stock changes in the top- (0–0.30 m) and subsoil layers (0.30–0.60 m) after one complete cycle of contrasting rotations (conventional vs. organic) that included either 2 or 3 years under grass-clover ley periods associated with different fertilisation sources (mineral vs. compost), and ii) characterise the SOM composition and make inferences about C stabilisation in these contrasting systems using SOM physical fractionation, TG-DSC-QMS, and Py-GC/MS-TMAH analyses. It was hypothesised that such management practices will significantly change SOC stocks on top- and subsoil layers, for which the unique combination of techniques will allow inferences about the processes of C stabilisation.
2 Materials and methods
2.1 Field site, experimental design, and treatments
The study used the Nafferton Factorial Systems Comparison (NFSC) trial based at Newcastle University’s Nafferton Farm, located 12 miles west of Newcastle-upon-Tyne in North-East England (54º59′09″N; 1º43′56″W, 60 m a.s.l). According to the Köppen classification system, the site experiences a marine west coast climatic condition, with the average annual temperature and total annual precipitation 8.6°C and 638.6 mm respectively, with a maximum monthly temperature of 22°C and a minimum of 0°C (Supplementary Figure S1, average record between a weather station located ∼5 miles away from the farm and by an on-site automated weather station). The soil in the experiment is classified predominantly as a uniform Dystric Stagnosol (WRB, 2015), slowly permeable, seasonally wet, acidic loamy and clayey soil that is naturally low in fertility (Farewell et al., 2011; Cranfield University, 2021); Cambic Stagnogley (Mückenhausen, 1981); Stagnic Cambisol (WRB, 2015). Analysis at the establishment of the experiment indicated an average SOC content of ∼3%, pH of 6.3, p = 62.9 mg kg−1, C/N = 8.6, and particle-size distribution with an average of 60.5, 22.5, and 17% of sand, silt and clay, respectively (sandy clay loam) (Cooper J. et al., 2011; Bilsborrow et al., 2013). The soil mineralogical composition across the farm is predominantly composed of quartz, with 1:1 clay minerals (kaolinite) and minor illite, and alkali feldspar, particularly in subsoil layers (>0.30 m) (Supplementary Table S1). Coal fragments, derived from till via glacial erosion of Carboniferous rocks, occurs in the soil, but insufficiently to confound measurement of non-geological SOC (Wang et al., 2022).
The experiment was established in 2001 in an area previously in a conventional arable rotation. It compares organic versus conventional crop rotation (RT), fertility sources (FS) and crop protection (CP) in a factorial design following either current United Kingdom conventional farming best practices (Red Tractor Assurance, 2015) or certified organic production practices (Soil Association, 2019). Full details on the design and past management of the trial can be found in several previous publications (Cooper J. M. et al., 2011; Cooper et al., 2011 J.; Eyre et al., 2011; 2012; McKenzie et al., 2011; Bilsborrow et al., 2013). Briefly, there are four replicate blocks in the field, each containing four sub-experiments with rotations beginning in different years. This was done to allow a diversity of crops to be present in the field in any given year. This study used samples from experiment No. 1 only. The 8-year conventional rotation (CONV-RT) is cereal-intensive beginning with 2 years of grass-clover ley while the organic rotation (ORG-RT) is more diverse consisting of 3 years of grass-clover ley followed by a mixture of cereals, spring beans, potatoes and vegetables (Table 1). The two levels of fertility source consist of mineral (MINE-FS) inorganic NPK fertiliser applied at rates recommended in the England Nutrient Management Guide (RB209, 2011) vs. compost (COMP-FS) fertilisation where only composted dairy manure is applied to the arable crops in the rotation at N rates equivalent to the MINE-FS treatment based on compost total N contents. While the NFSC includes both organic and conventional crop protection treatments, for the purposes of this study only plots under conventional crop protection were included (Supplementary Table S2). The main crop rotation plots are each 12 m × 96 m in size. These are subdivided into two crop protection sub-plots (12 m × 48 m) and further subdivided into two fertility source sub-sub-plots (12 m × 24 m). Figure 1 shows the layout of these plots in Block 1 of the experiment as an example. A full layout of the NFSC experiment is provided in Supplementary Figure S2. Further details of treatments used during the timeframe of this study including crops grown in the rotations every year and rates of fertilisation, are given in Table 1. Crop protection details are shown in Supplementary Table S2.
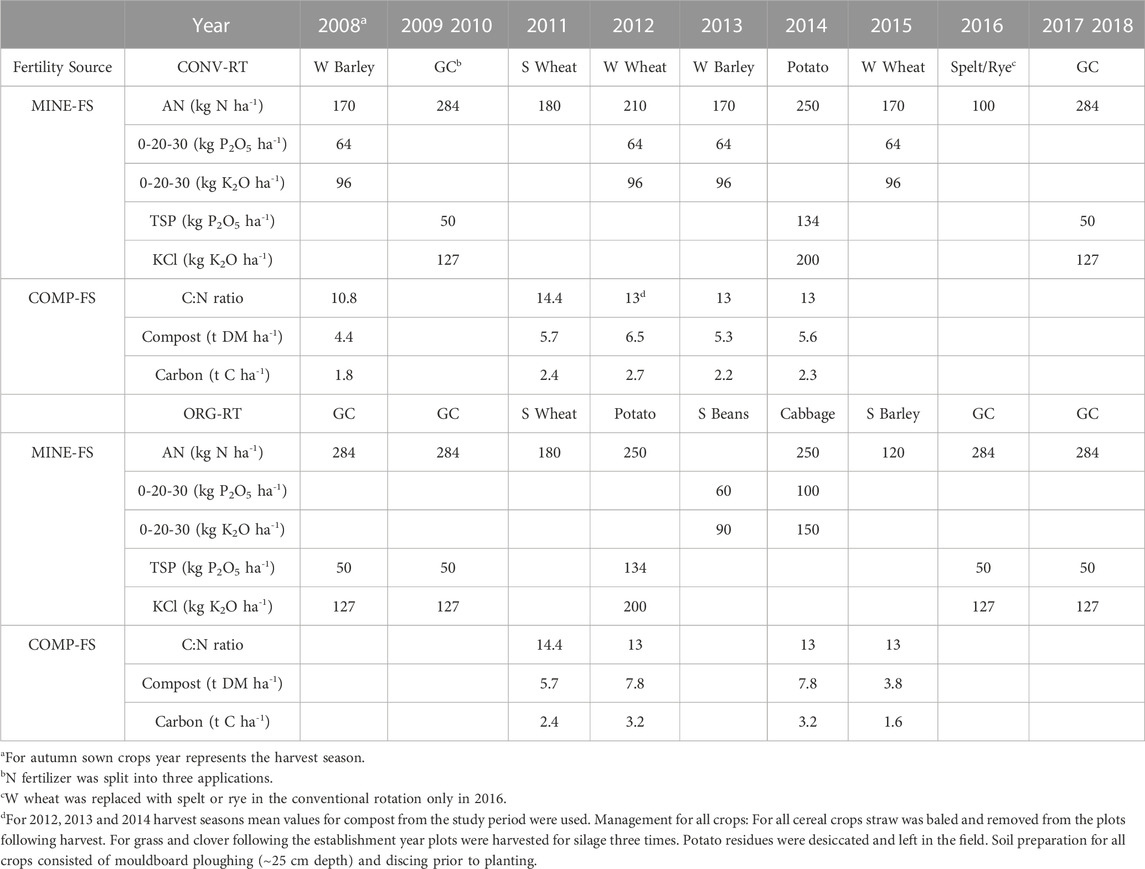
TABLE 1. Crop sequence, fertility inputs, and management details for conventional and organic rotations (conventional-CONV-RT vs. organic-ORG-RT) and fertility sources (mineral-MINE-FS vs. compost-COMP-FS) in the NFSC trial between 2008 and 2018. GC: perennial ryegrass/white clover ley; W = winter; S = spring; AN = ammonium nitrate; TSP = triple super phosphate; KCL = muriate of potash; 0-20-30 compound fertiliser containing 20% P2O5 and 30% K2O.
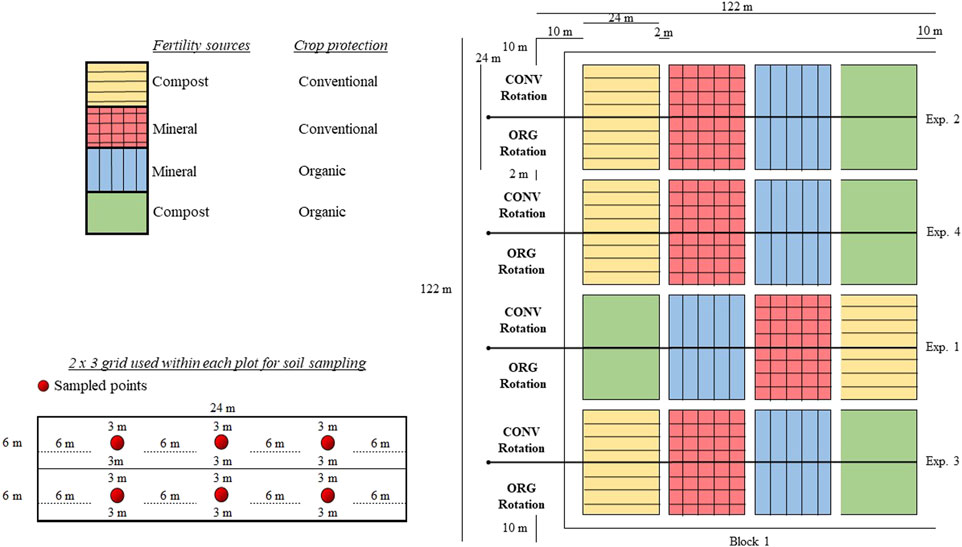
FIGURE 1. Nafferton Factorial Systems Comparison (NFSC) main block layout (122 m × 122 m) and experimental design used for soil sampling (bottom left). The 2 × 3 sampling grid is zoomed in for one-half of the plot layout (i.e. 12 × 24 m). Schematic soil sampling location within each half-plot is represented by red points. Crop rotation is divided into conventional (CONV) and organic (ORG) rotation levels. The main block layout is repeated four times in the field. Exp. refers to experiments (1, 2, 3, and 4). Full layout design is provided in Supplementary Figure S2.
2.2 Soil sampling and sample preparation for soil analyses
Soil sampling was conducted in the same sub-experiment No. 1 at the beginning of the rotation (March 2011) and at the last year of the rotation (March 2018). In both years, soil sampling was conducted during the grass-clover ley periods (i.e., in 2011, it was carried out just before wheat planting and in 2018, just before the ley phase-out). In each one of the 16-target treatment plots, six intact soil cores (0–0.60 m depth) were taken in 2 × 3 grid spaced at 6 m apart to obtain a representative sample for the plot (Figure 1). The soil cores were collected using a hydraulic soil sampler (Atlas Copco Ltd., Hemel Hempstead, Hertfordshire, United Kingdom) and a metallic tube (1 m length, 0.30 m inner diameter). The collected cores were separated into two soil depth intervals (0–0.30 and 0.30–0.60 m) totalling 384 soil samples (192 collected in 2011 and 192 collected in 2018). Each one of the soil samples was gently mixed and passed through a 4 mm sieve; large stones were removed and weighed plant remains were discarded. The weight of the sieved fresh soil was then recorded. A subsample of the sieved soil (∼5 g) was used for determination of gravimetric water content. The soil bulk density (BD) was calculated using the core method adjusting for the weight and volume of large stones (Blake and Hartge, 1986). Soil pH of samples was measured in H2O (1: 2.5 soil: solution), following analytical procedures described in Mc Lean (1982). After BD determination and pH measurement, the six soil samples taken from the same plot, sampling year and depth interval were pooled into a composite sample. This resulted in 64 composite soil samples, 32 for each sampled year, which were wet sieved through a 2 mm sieve and air-dried before further analyses.
2.3 Total carbon and stocks calculation
Soil carbon (C) concentration was determined by dry combustion (Nelson and Sommers, 1996), post-combustion and reduction tubes in an Elementar Vario Macro Cube analyser (furnace at 960°C in pure oxygen). SOC stocks per unit of area (Mg ha−1) were calculated for each depth interval (i.e. 0-0.30 and 0.30–0.60 m) on an equivalent soil mass basis (Wendt and Hauser, 2013) using the 2011 samples as a reference. The difference in SOC stocks between 2011 and 2018 samples were used to calculate accumulation or reduction rate during one rotation cycle (i.e., in 8-year period).
2.4 Physical fractionation of SOM
The method of SOM fractionation used in this study was primarily used by Christensen (1985), later described by Cambardella and Elliott (1992) and further adjusted by C. Feller and Beare (1997). The method is also further discussed and clarified in Christensen (1992). The method separates soil particles, often by using high-density liquids (often between 1.6–2.0 g cm−3), into organic (particulate organic matter; POM >53 μm) and mineral-associated fractions (heavy fraction and silt and clay fraction; HF > 53 μm and SC < 53 μm, respectively) by dispersion, wet sieving, flotation and sedimentation, followed by a subsequent mass balance check (Christensen, 1992; 2001). In this study, however, it was chosen to not use any chemicals-based high density liquid but only Milli-Q water during the procedures, to minimise disruption of the chemical structure of the original SOM and reduce risk of chemical contamination (Lehmann and Kleber, 2015). This approach also avoids possible interferences in TG-DSC-QMS and Py-GC/MS-TMAH analysis. While the use of Milli-Q water, rather than high-density liquids, has raised some concerns, Poeplau et al. (2018) pointed out that the use of a liquid with density of 1 g cm−3 performed in the best top 5 out of 20 methods. The only potential drawback is that soil particles might not be completely dispersed, which can result in inconsequential retention of mass and/or C in fractions to which they do not belong (von Lützow et al., 2007; Lavallee et al., 2019).
In summary, 20 g of each air-dried soil sample (i.e., for all 64 samples) was sonicated in 70 mL of Milli-Q water at 500 W for 15 min (providing approximately13 J per sample or 144 J mL−1) using an ultrasonic processor (Model VC-505; Sonics Vibra Cell). After sonication, the sample was wet sieved through a 53 μm sieve using Milli-Q water. The HF and POM fractions were retained in the sieve and were separated by flotation and sedimentation using Milli-Q water (1 g cm−3). This procedure generated 192 fraction samples (64 samples x 3 fractions). Each fraction was oven-dried at 40°C and their weights recorded. SOC concentration of each fraction was determined following the preparation and dry combustion methods described above. For quality assurance, the final recovery of the soil mass was checked against the original 20 g and the recovery of the elemental analysis for the fractions was checked against SOC concentrations from the <2 mm samples (Supplementary Table S3 and Supplementary Figures S3, S4). SOC concentration and the masses of each fraction were used for the calculation of SOC in each fraction and the results were reported on a per kilogram bulk soil basis (g C kg−1). SOC concentrations of the individual fractions and their recovery soil masses are given in Supplementary Table S4.
2.5 Thermogravimetry-differential scanning calorimetry-quadrupole mass spectrometry (TG-DSC-QMS)
Thermal analysis was used to examine the relative proportions of different “fractions” of C in the soil samples, termed the labile, recalcitrant and refractory fractions following the methods described by Lopez-Capel et al. (2005a); Fernández et al. (2012). The samples were analysed using thermogravimetry (TG) and differential scanning calorimetry (DSC), combined with quadrupole mass spectrometry (QMS) analysis of the gas evolved during thermal decomposition. While TG and DSC data quantifies the weight change and the gain/loss in energy of the sample during heating, QMS analysis provides data on the chemical composition of the gaseous combustion products, which can be used to characterise the sample in terms of its organic and inorganic components.
Sixteen soil samples were selected for TG-DSC-QMS analysis, one composite soil sample per treatment per depth per year (i.e., considering the combination of treatment factors): i) conventional crop rotation with mineral fertilisation source (CONV-M); ii) conventional crop rotation with compost fertilisation source (CONV-C); iii) organic crop rotation with mineral fertilisation source (ORG-M); and iv) organic crop rotation with compost fertilisation source (ORG-C). The samples were selected with reference to the mean total C content obtained by the dry combustion method such that the sample selected for analysis had a total C content closest to the mean (Supplementary Table S5).
An aliquot of each sample (ca. 50 mg) was weighed accurately into an alumina crucible and analysed using a Netzsch Jupiter STA 449C thermogravimetry-differential scanning calorimetry (TG-DSC) analyser. Samples were heated from 25°C to 1,000°C at a rate of 10°C min−1 in an (oxidizing) atmosphere of 20% oxygen and 80% helium (purge gas, flow rate 30 mL min−1). The protective gas was helium (flow rate 20 mL min-1). TG and DSC data were acquired and processed using Netzsch Proteus 61 software. For mass spectrometric analysis, the evolved gas stream was sampled continuously through a fused silica capillary transfer line connected to a Netzsch Aeolos 403C quadrupole mass spectrometer (QMS). Adapter heads and the transfer line (between the Jupiter and Aeolos) were at 150°C. The QMS was operated in full scan mode over the range m/z 10–160 and the dwell time was 0.2 s, giving a sampling rate of ca. 1 scan per 5°C increase in temperature. Mass spectrometric data were acquired and processed using Aeolos software.
In short, TG-DSC was used to determine the relative proportions of labile, recalcitrant and refractory C fractions by comparing the total weight loss over the temperature range 200°C–750°C (Exotot) with its relative proportions from the defined intervals: i) 200°C–350°C (Exo 1), ii) 350°C–500°C (Exo 2) and iii) 500°C–750°C (Exo 3). These temperatures were established based on the first derivatives of the DSC traces (i.e., distinct exothermic reactions), in accordance with the methods described by Dell’Abate et al. (2000); Dell’Abate et al. (2002). Additionally, we have used m/z 18 (water) to distinguish the different organic matter pools (Supplementary Figure S5). Briefly, thermal decomposition between 200°C to approximately 350°C will release relatively volatile and labile forms of C, whilst decomposition between 350°C–650°C will release more recalcitrant and refractory C forms, such as lignin and related biopolymers (Plante et al., 2009). Soil carbonate minerals, if any, decompose at 750°C–800°C. The curves of the gas evolution (i.e., the QMS data) were interpreted in order to assess the contribution of individual peaks into the overall trace (Arenillas et al., 1999). The main ion of interest in the QMS analysis was m/z 44 (carbon dioxide). For each sample, the QMS data for the selected ion (m/z 44) were normalised to the total ion intensity to allow comparison of different samples (Arenillas et al., 1999). The corresponding variation in abundance of the m/z 44 with the variation in TG and DSC curves was used to verify the organic origin of the three fractions and differentiate these from the decomposition of carbonate minerals (inorganic carbon). The same intervals considered in the TG-DSC approach (200°C–350°C, 350°C–500°C, 500°C–750°C) were used to seek CO2 peaks and to calculate the area under the peaks, representing the proportion of C released.
2.6 Pyrolysis-gas chromatography-mass spectrometry (Py-GC-MS)
The same sixteen soil samples used for TG-DSC-QMS were subjected to Pyrolysis-Gas Chromatography-Mass Spectrometry (Py-GC-MS) analysis (Supplementary Table S5). Whilst Py-GC-MS has allowed comparison of SOM produced under different environments and land uses (Buurman and Roscoe, 2011; Oliveira et al., 2016), the highly polar pyrolysis products from biopolymers can be either difficult or impossible to detect by Py-GC-MS analysis (Challinor, 1989; Kaal and Janssen, 2008). To address this potential deficiency, on-line thermally assisted hydrolysis and methylation (THM) in the presence of tetramethylammonium hydroxide (TMAH) has been used together with Py-GC-MS. Accordingly, phenolic compounds formed from the TMAH-induced cleavage of ether and ester bonds, which are present in soils from plant-derived macromolecular organic C, can be also characterised (Mason et al., 2012). In this sense, all Py-GC-MS analyses in this study were conducted by THM in the presence of TMAH following adapted analytical procedures described by Abbott et al. (2013).
Analytical procedures involved the use of a Frontier Laboratories Single-shot Pyrolyser Model PY-3030S. The pyrolyser was connected to an HP 6890 gas chromatograph (GC) and interfaced to an HP 5973 MSD. The pyrolysis temperature and time were 610°C and 1 min, respectively. The GC inlet was heated at 320°C and the sample was injected in split mode with a split ratio of 30:1. Gas chromatographic separation of compounds was performed using a Phenomenex ZB-5MS (Torrance, CA, United States of America) fused silica capillary column (60 m × 0.25 mm i.D. x 0.25 μm film thickness). The GC oven temperature program was 50 °C (initial hold time 1 min) then 4°C min−1 to 320°C (final hold time 10 min). Helium was used as carrier gas at a constant flow rate of 1 mL min−1. The GC-MS was operated in full scan mode, scanning the range m/z 50–650. Operating conditions were; electron voltage 70 eV, emission current 35 μA, source temperature 230°C, quadrupole temperature 150°C, multiplier voltage 2200 V and interface temperature 320°C. All the analytical procedures were conducted in triplicate so that analytical reproducibility could be checked.
Data acquisition and processing were performed using Agilent Chemstation software and pyrolysis products were identified using the Chemstation NIST05 library of mass spectra. All prominent, identifiable, products of each sample were quantified relative to the internal standard and reported as a proportion of the total peak area of the identified characteristic ions (i.e., m/z values). The identified products were grouped into n-alkanes, n-alkenes, aromatics, benzofurans, carbohydrates, fatty acids, lignin phenols, N containing compounds, phenols and polycyclic aromatic hydrocarbons (polyaromatics). These groups were defined based on the origin and chemical similarity of the identifiable products.
2.7 Statistical analyses
Linear mixed-effects models were fitted to test the main effects of crop rotation (RT); i) conventional (CONV-RT) vs. organic (ORG-RT); fertility sources (FS); mineral (MINE-FS) vs. compost (COMP-FS), year of sampling (YR) (2011 and 2018) and their interactions (RT*FS*YR) on soil organic C stocks (SOC stock) and C in the SOM fractions (POM >53 μm, HF > 53 μm and SC < 53 μm). Results from the Thermogravimetry-Differential Scanning Calorimetry-Quadrupole Mass Spectrometry (TG-DSC-QMS) and the Pyrolysis-Gas Chromatography-Mass Spectrometry (Py-GC-MS) analyses were only used to elucidate processes, and therefore were not statistically assessed.
For all models, fixed effects were crop rotation, fertility sources, year of sampling and their three-way interaction. The random effect was defined as block, with plot nested to it to account for repeated measures (i.e., years of sampling). The analyses were conducted separately for each depth interval (i.e. 0–0.30 and 0.30–0.60 m). Assumptions were checked for normality and equal variances by examining the QQ plots of residuals (for both fixed and random effects compartments of the model) and scatterplots of standardised against fitted values. The data were Tukey’s Ladder of Powers transformed when visual breakdowns in the model assumptions were revealed by residual plots. To test the significance of the fixed effects on the dependent variables, models were compared with and without the factor of interest using the likelihood ratio tests (LRT) approach. When the interaction term in the model was significant, Tukey’s HSD post hoc test was carried out and a significant effect was determined at p < 0.05. All statistical analysis was carried out in the R programming language 3.4.3 (Team, 2019) using the additional packages, ape (Paradis et al., 2004), nlme (Pinheiro et al., 2018), plyr (Wickham, 2011), ggplot2 (Wickham, 2009), and multcomp (Hothorn et al., 2008) and following statistical approaches described by Zuur et al. (2009).
3 Results
3.1 SOC stocks
For the 0–0.30 m depth, there was an interactive effect between FS and YR affecting SOC stocks (LRT = 5.19; p = 0.02, respectively; Table 2). This has shown that COMP-FS significantly increased SOC stocks over time (i.e., from 2011 to 2018) from 54.81 ± 1.98 to 60.86 ± 1.11 Mg ha−1, suggesting an accumulation mean of 11% at a rate of 0.76 Mg ha yr−1. MINE-FS, on the other hand, increased SOC stocks over the years from 57.74 ± 2.03 to 60.42 ± 1.48 Mg ha−1, i.e., SOC stock accumulation mean of approximately 5% and C accumulation rate of 0.33 Mg ha yr−1, which were not statistically verified (Figure 2). SOC stocks were also higher in the ORG-RT compared with CONV-RT (approximately 5%), regardless of the FS (MINE or COMP) or YR (2011 or 2018) (LRT = 4.45; p = 0.03) (Table 2). In an 8-year rotation, this translates into SOC accumulation rate of 0.31 Mg ha yr−1.
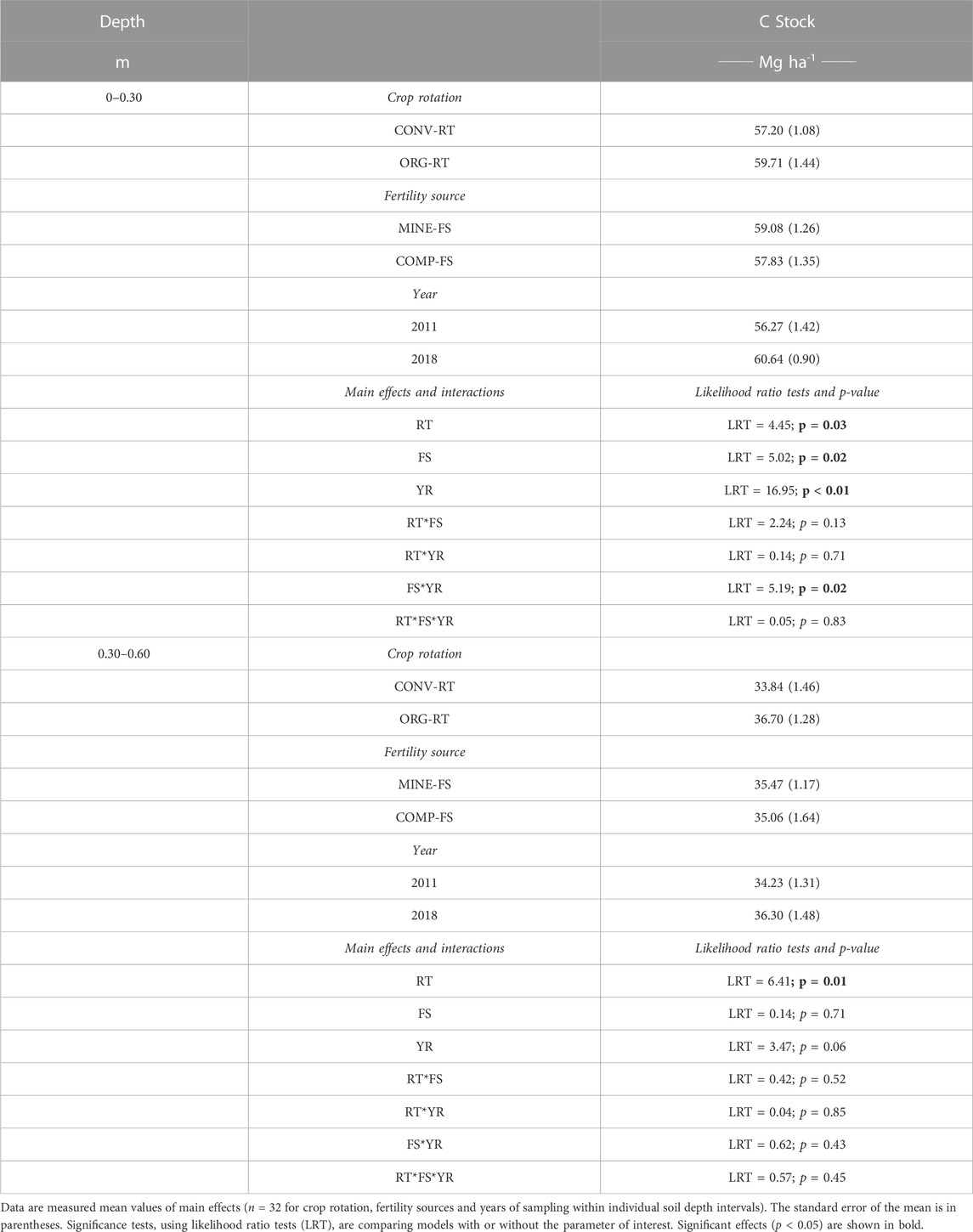
TABLE 2. Main effect means for crop rotation (RT) (conventional-CONV-RT vs. organic-ORG-RT), fertility sources (FS) (mineral-MINE-FS vs. compost-COMP-FS), and years of sampling (YR) (2011 and 2018). Significant of the main effects and their interactions on soil organic C stocks (SOC stock) at 0–0.30 and 0.30–0.60 m soil depth intervals.
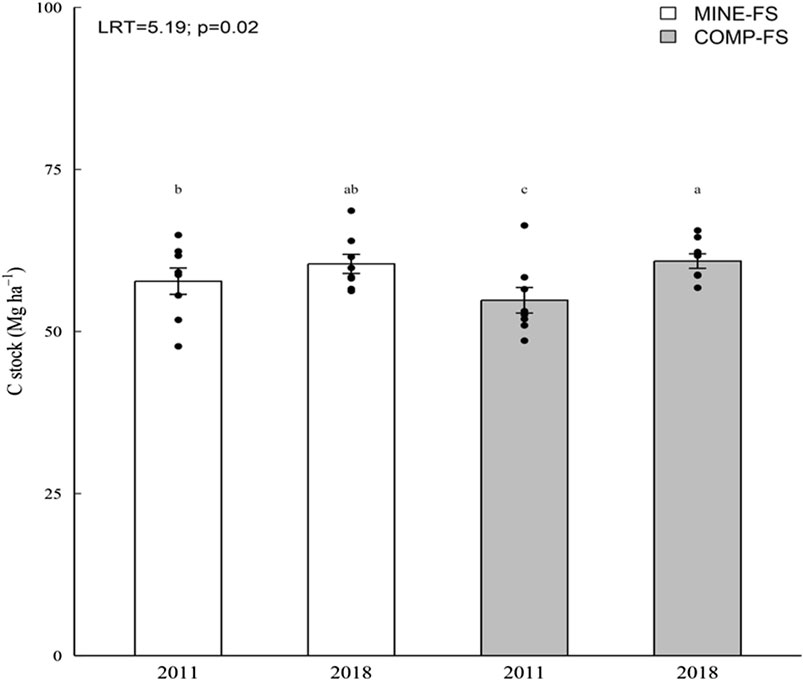
FIGURE 2. Interactive effects between fertility sources (mineral-MINE-FS and compost-COMP-FS) and years of sampling (2011 and 2018) on soil organic C stocks (C stock Mg ha−1) in the 0–0.30 m. Data are measured mean values ±SE (n = 8 for crop rotation schemes, fertility sources and years of sampling). Significance tests, using likelihood ratio test (LRT), are comparing models with or without the parameter of interest.
For deeper soil layers (0.30–0.60 m), SOC stocks were only affected by RT (LRT = 6.41; p = 0.01), where ORG-RT showed higher SOC stocks than CONV-RT regardless of the FS or YR (Table 2).
3.2 SOC distribution in SOM fractions
The average mass balance recovery of physical fractionation ranged between 97% and 98% (Supplementary Table S3), which indicates technique reliability, particularly in terms of mass recovery, for assessing SOM fractions. Whilst most of the soil mass was found in the HF fraction, higher SOC concentration was found in the SC (<53 μm) than either of the other >53 μm fractions (POM or HF), regardless of soil depth interval (0–0.30 or 0.30–0.60 m), RT, FS or YR (Table 3; Supplementary Figure S4).
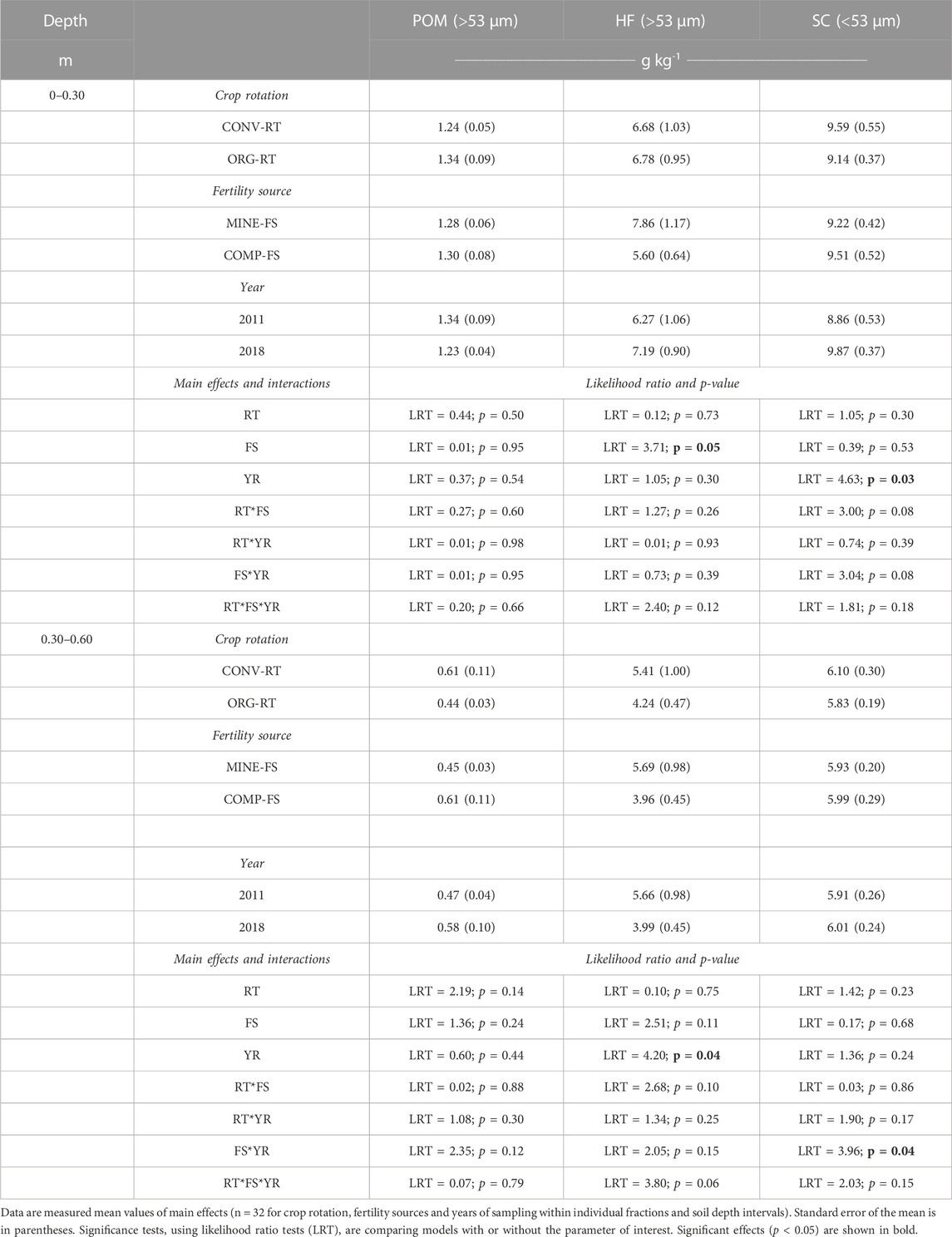
TABLE 3. Main effect means for crop rotation (RT) (conventional-CONV-RT vs. organic-ORG-RT), fertility sources (FS) (mineral-MINE-FS vs. compost-COMP-FS), years of sampling (YR) (2011 and 2018). Significance of the main effects and their interactions on soil organic C concentrations (g per kg-1 soil) in the organic fraction (particulate organic matter-POM >53 μm), heavy fraction (HF > 53 μm) and mineral-associated fraction (silt and clay fraction-SC < 53 μm) at 0–0.30 and 0.30–0.60 m soil depth intervals.
For the 0–0.30 m depth, although SOC concentration in the POM was numerically higher in 2011 than in 2018 samples, the difference was not statistically significant (LRT = 0.37, p = 0.54). The MINE-FS had higher SOC concentration in the HF (>53 μm) fraction compared to the COMP-FS (LRT = 3.71; p = 0.05). In 2018, SOC concentration was higher in the SC fraction compared to 2011 (LRT = 4.63; p = 0.03) (Table 3).
For the 0.30–0.60 m depth, SOC concentration in the POM was not affected by RT, FS or YR (p > 0.05). SOC concentration in the HF (>53 μm) fraction was affected by YR, showing a significant increase from 2011 to 2018 (LRT = 4.20; p = 0.04) irrespective of the RT and FS (Table 3). In the same depth interval (i.e. 0.30–0.60 m), FS and YR interacted resulting in an increased SOC concentration in the SC over time (i.e., from 2011 to 2018) from 5.60 ± 0.48 to 6.26 ± 0.27 g C kg−1 under MINE-FS, whilst under COMP-FS decreased SOC concentration in the SC fraction from 6.22 ± 0.53 to 5.77 ± 0.65 g C kg−1 (LRT = 3.96; p = 0.04) (Figure 3).
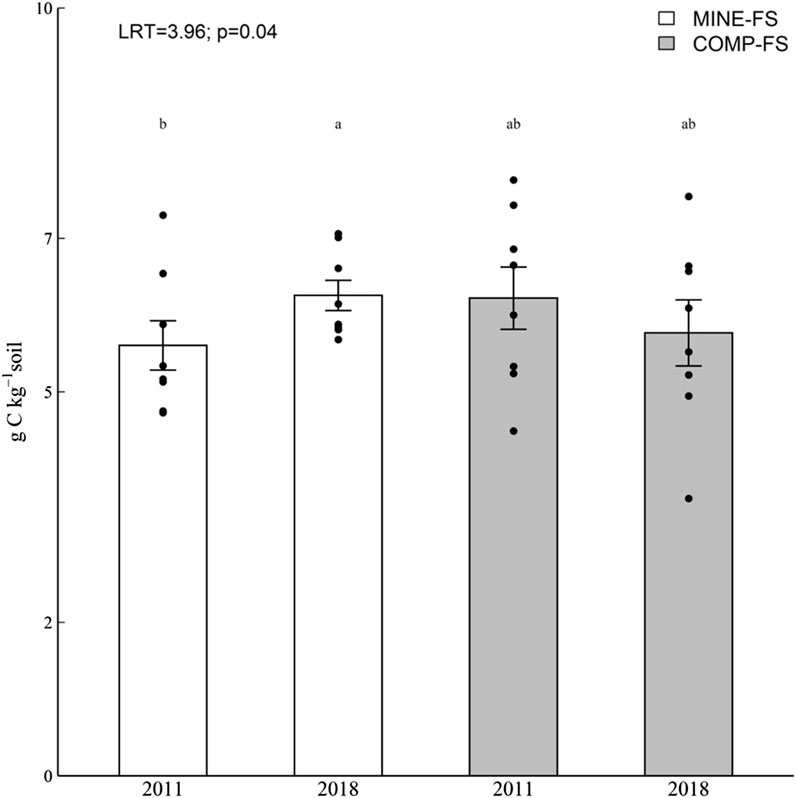
FIGURE 3. Interactive effects between fertility sources (mineral-MINE-FS and compost-COMP-FS) and years of sampling (2011 and 2018) on silt and clay fraction (SC < 53 μm) at 0.30–0.60 m soil depth interval. Data are measured mean values ±SE (n = 8 for fertility sources and years of sampling). Significance tests, using likelihood ratio test (LRT), are comparing models with or without the parameter of interest.
3.3 Thermogravimetry-differential scanning calorimetry-quadrupole mass spectrometry (TG-DSC-QMS)
Total weight loss and relative weight loss values from different temperature intervals (Exo 1 – 200°C–350°C; Exo 2 – 350°C–500°C; and Exo 3 – 500°C–750°C), which represent material loss during heating (e.g., labile, recalcitrant and refractory), are given in Table 4.
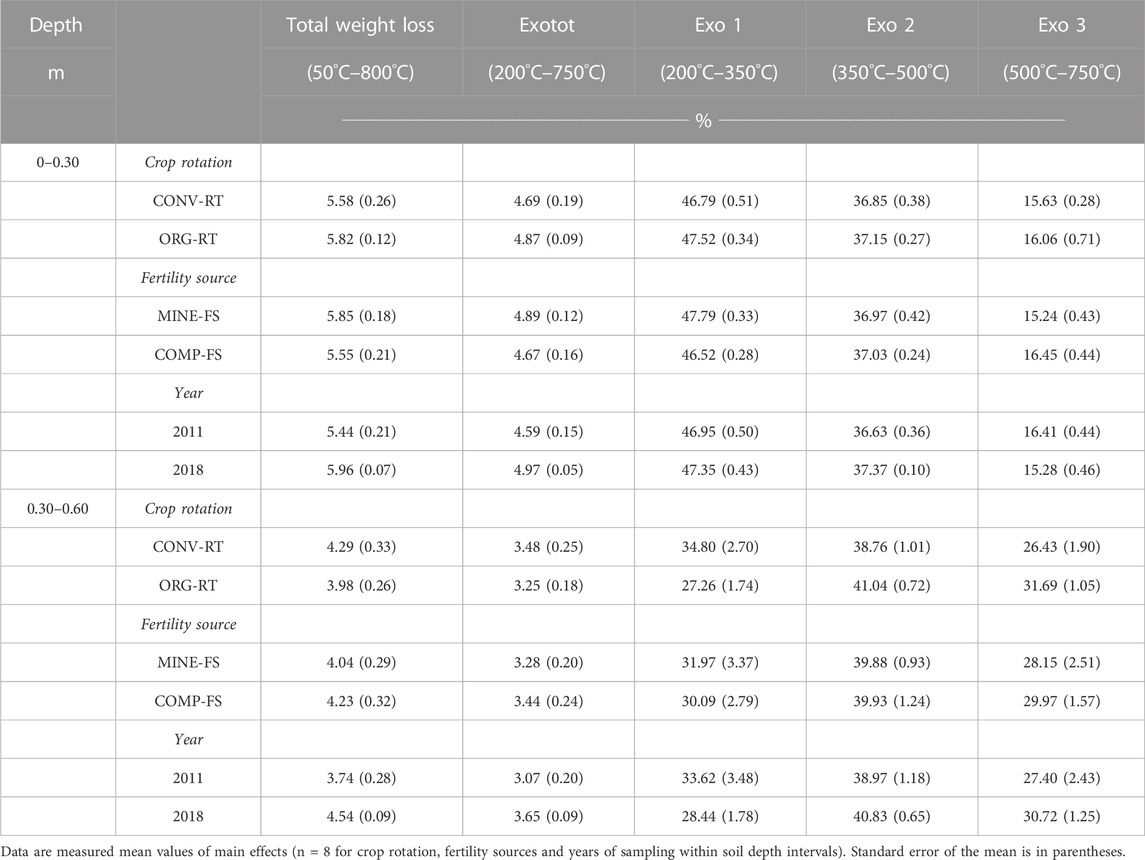
TABLE 4. Changes in total weight loss (50°C–800°C), weight loss for the temperature interval 200°C–750°C (Exotot) and relative weight losses of temperature intervals 200°C–350°C (Exo 1), 350°C–500°C (Exo 2) and 500°C–750°C (Exo 3) as a result of different crop rotation (conventional-CONV-RT or organic-ORG-RT), fertility sources (mineral-MINE-FS or compost-COMP-FS) and years of sampling (YR) (2011 and 2018).
For the 0–0.30 m depth, soil samples showed discrete weight loss variability between the treatments, with labile (Exo 1) and recalcitrant plus refractory (i.e., the sum of Exo 2 + Exo 3) fractions being evenly distributed within the samples (approximately 50/50). In general, ORG-RT, MINE-FS and samples collected in 2018 showed a slightly more weight loss in the labile fraction compared to their counterparts CONV-RT, COMP-FS and samples collected in 2011 (Exo 1). Likewise, ORG-RT and samples collected in 2018 showed a slightly more weight loss in the refractory fraction compared to their counterparts CONV-RT and samples collected in 2011 (i.e., Exo 2 + Exo 3), while COMP-FS outperformed MINE-FS at the same temperature intervals (i.e., Exo 2 + Exo 3) (Table 4).
For deeper soil layers (0.30–0.60 m), more disparity in weight loss was observed between the treatments, with recalcitrant plus refractory fractions (Exo 2 + Exo 3) dominating over the labile fractions (Exo 1) (Table 4). CONV-RT, MINE-FS and samples collected in 2011 showed more weight loss in the labile fraction compared to their counterparts ORG-RT, COMP-FS and samples collected in 2018 (Exo 1). The opposite was observed for the refractory organic matter (Exo 2 + Exo 3), i.e., ORG-RT, COMP-FS and samples collected in 2018 showing more weight loss in the refractory fraction than CONV-RT, MINE-FS and samples collected in 2011 (Table 4).
These differences are highlighted by the differential scanning calorimetry analysis (DSC traces), which showed three exothermic peaks between 200°C and 600 °C in the topsoil (0–0.30 m), characterised by a distinct peak at 300°C–350°C and two other broad peaks, one at 400°C–450°C and another at 500°C–550°C (Supplementary Figure S6A, B). Subsoil (0.30–0.60 m) samples also showed three exothermic peaks characterised by a distinct peak at 400°C–450°C and two other broad peaks, one at 300°C–350°C and another at 500°C–550°C (Supplementary Figure S6C, D). Regardless of the RT, FS or YR, all samples showed an endothermic peak at approximately 570°C–580°C for both depth intervals assessed (Supplementary Figure S6).
Changes in the relative ion intensity for CO2 (m/z 44) clearly show the difference in SOM character when comparing different depths (Figure 4). The shallow samples show a simple major peak around 300°C–350°C, whereas the deep samples show a more complex set of peaks with the strongest around 450°C.
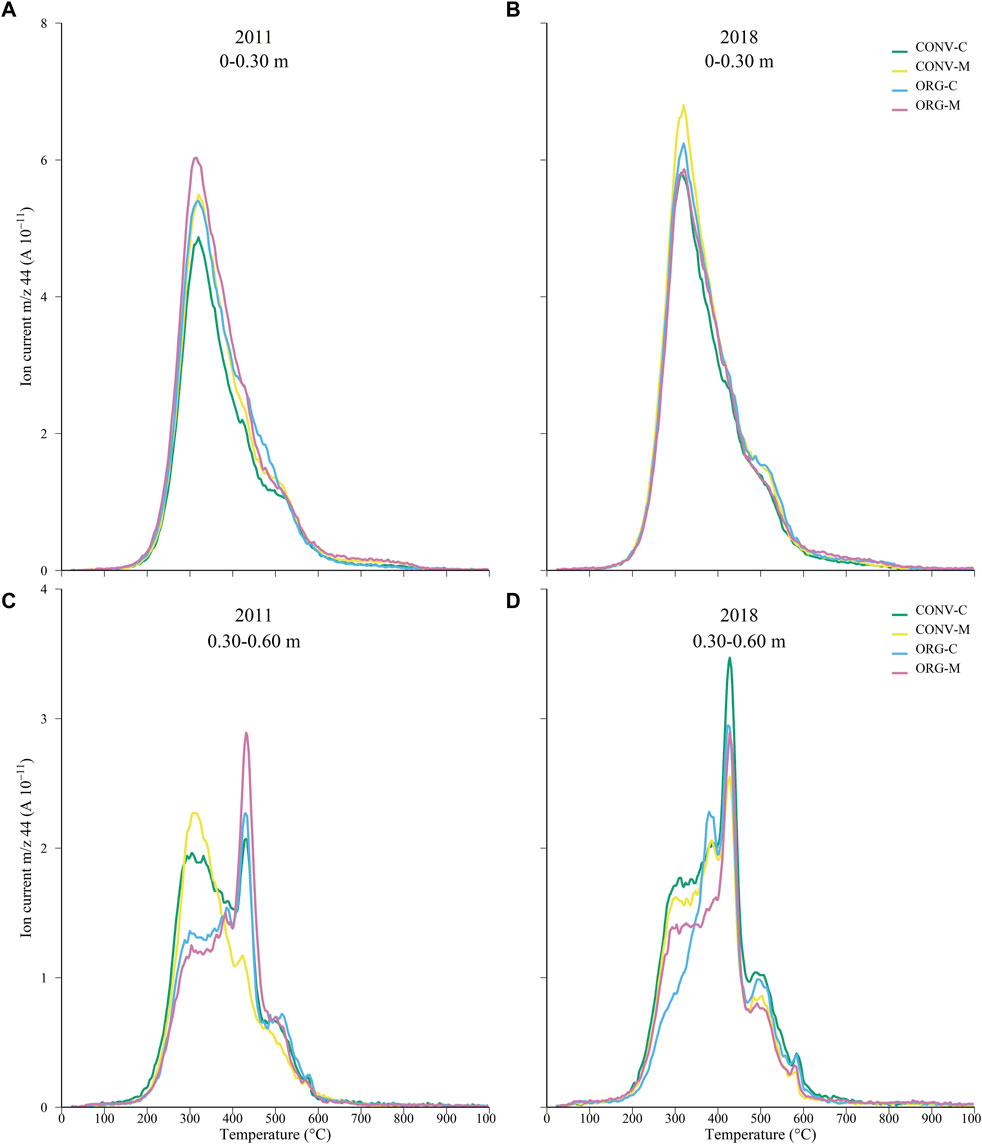
FIGURE 4. Ion current intensity for CO2 (m/z 44) from the soil samples of combined treatment factors: conventional rotation with mineral fertilisation (CONV-M), conventional rotation with compost fertilisation (CONV-C), organic rotation with mineral fertilisation (ORG-M) and organic rotation with compost fertilisation (ORG-C) at 0–0.30 (A, B) and 0.30–0.60 m (C, D) soil depth intervals and different years of sampling 2011 (A, C) and 2018 (B, D).
For the 0–0.30 m depth, regardless of the YR, all samples showed a similar pattern with m/z 44 reaching a maximum at around 300°C–350°C and with two minor shoulders at 400°C–450°C and 500°C–550°C (Figures 4A,B). Except for the ORG-M treatment (i.e., organic rotation with mineral fertilisation), all the other treatments showed a slight increase in the C released, particularly in the first temperature interval (Exo 1 – 200°C–350°C), in 2018 compared to 2011. The other two temperature intervals (Exo 2 + Exo 3 – 350°C–500°C and 500°C–750°C), which represent recalcitrant and refractory fractions, showed a similar release of C with the ORG-RT, COMP-FS and samples collected in 2018 being slightly predominant than their counterparts (CONV-RT, MINE-FS and 2011 samples) (Figures 4A,B). These results are especially highlighted when the amount of C released within each temperature interval was calculated using the m/z 44 peak areas (Table 5). In general, there was a little variability between the treatments in the topsoil (0–0.30 m), with labile (Exo 1 – 200°C–350°C) and recalcitrant and refractory (Exo 2 + Exo 3 – 350°C–500°C and 500°C–750°C, respectively) fractions showing similar C amounts (approximately 50/50). The only major difference observed was regarding the YR, where 2018 samples had higher soil C amounts than 2011 samples (Table 5).
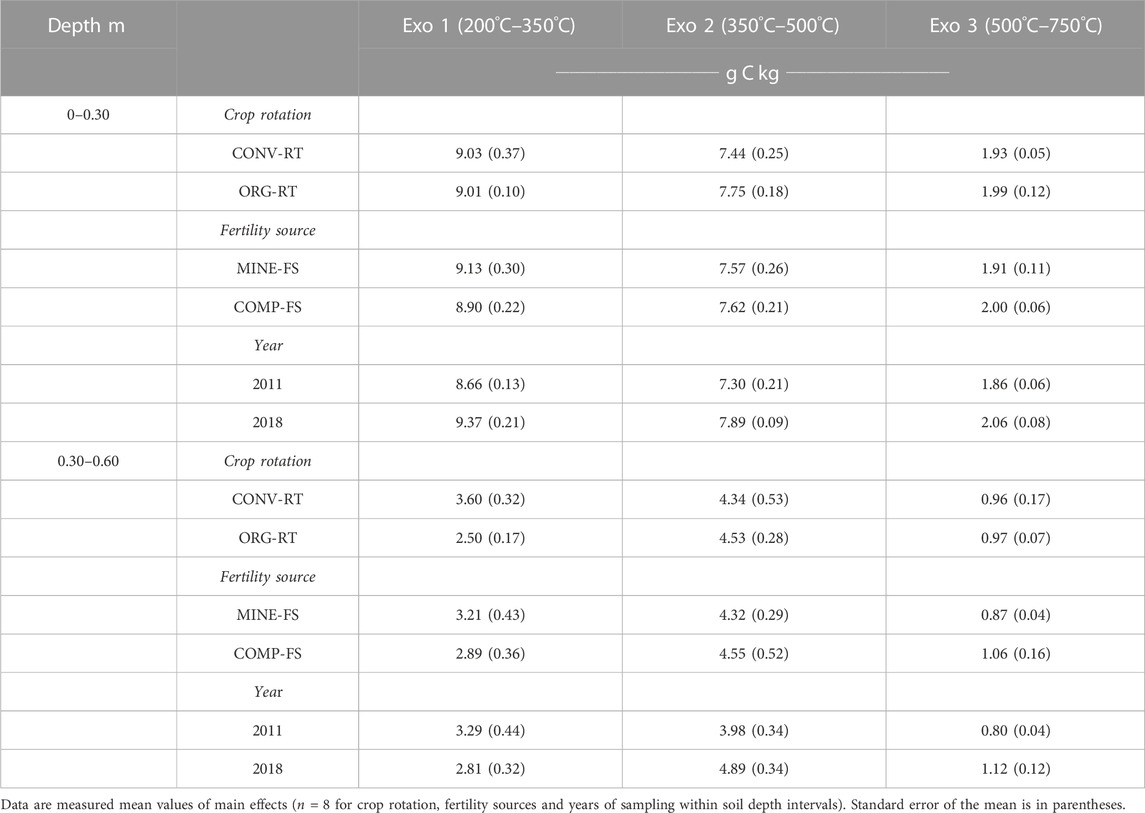
TABLE 5. Changes in carbon (C) released calculated from the m/z 44 (CO2) peak areas in the temperature intervals 200°C–350°C (Exo 1), 350°C–500°C (Exo 2) and 500°C–750°C (Exo 3) as a result of different crop rotation (conventional-CONV-RT or organic-ORG-RT), fertility sources (mineral-MINE-FS or compost-COMP-FS) and years of sampling (YR) (2011 and 2018).
For deeper soil layers (0.30–0.60 m), in both years (2011 and 2018), the m/z 44 reached a maximum at around 400°C–450°C, with two other shoulders observed at 300°C–350°C and 500°C–550°C (Figures 4C,D). Under CONV-RT, there was a shift from 2011 to 2018 in C released to higher temperatures, particularly with the combination of CONV-RT and COMP-FS (CONV-C treatment), which resulted in the highest peak observed under the subsoil layer (Figures 4C,D). Under the ORG-RT, similar peaks were observed between the 2 years of sampling (2011 and 2018). However, it appears that the combination of ORG-RT and COMP-FS (ORG-C treatment) slightly shifted the release of C to higher temperatures resulting in a higher peak at 400°C–450°C whereas the peaks remained unchanged in the combination of ORG-RT and MINE-FS (Figures 4C,D). These results were confirmed by the amount of C released within each temperature interval using the m/z 44 peak areas (Table 5). The CONV-RT, MINE-FS and samples collected in 2011 showed a higher release of C at the first interval (Exo 1 – 200°C–350°C) compared to ORG-RT, COMP-FS and samples collected in 2018. For the recalcitrant and refractory fractions (Exo 2 + Exo 3), 2018 samples showed higher soil C than 2011 samples (Table 5).
For both top- (0–0.30 m) and subsoil layers (0.30–0.60 m), there were no peaks between the 750ºC–900°C temperature range, indicating that there were no detectable soil carbonate minerals present in the samples, therefore, total soil C concentration can be assumed to be equal to total SOC (Figure 4).
3.4 Pyrolysis-gas chromatography-mass spectrometry (Py-GC-MS) in the presence of tetramethylammonium hydroxide (TMAH)
More than 300 pyrolysis product compounds were released of which 184 dominant product compounds were selected and quantified (Supplementary Table S6). All the quantified product compounds are listed in Supplementary Table S6, with their position in the chromatogram indicated by retention time (ReT). Supplementary Table S6 also provides information about the chemical group of the quantified product compounds and in which soil depth interval (0–0.30 and 0.30–0.60 m) they were found. Table 6 provides the relative abundance of the quantified pyrolysis product compounds by chemical groups.
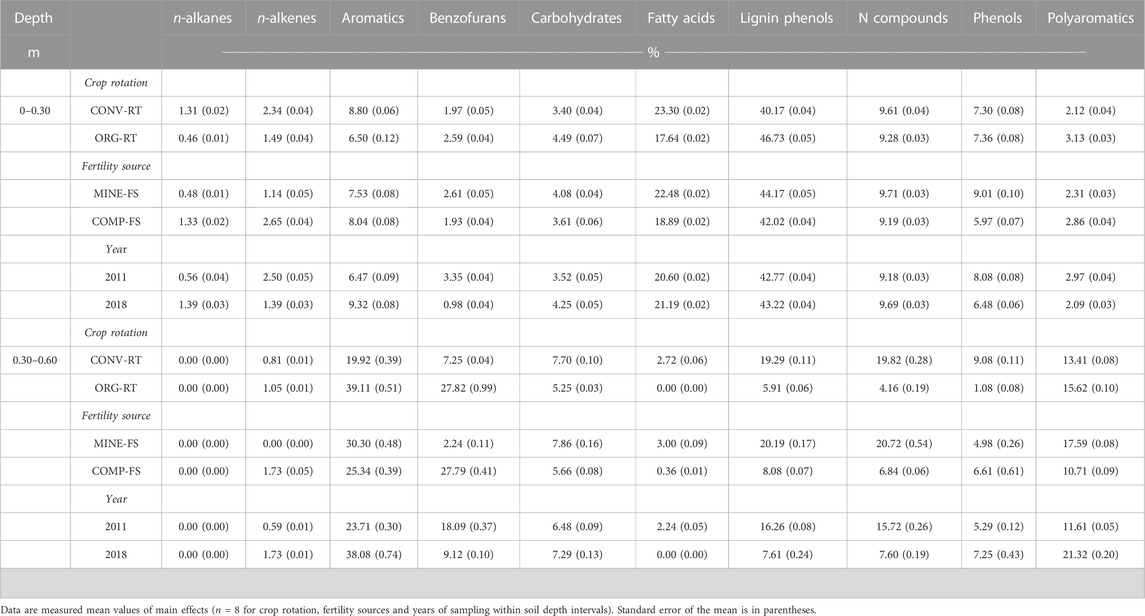
TABLE 6. Relative abundance of groups of pyrolysed product compounds released after Py-GC-MS-TMAH analytical procedures as a result of different crop rotation (conventional-CONV-RT or organic-ORG-RT), fertility sources (mineral-MINE-FS or compost-COMP-FS) and years of sampling (YR) (2011 and 2018).
For the 0–0.30 m soil depth, 161 quantified compounds were observed of the total 184 detected (Supplementary Table S6). More specifically, ORG-RT showed a higher relative abundance of benzofurans, carbohydrates, lignin phenols, phenols, and polyaromatics compared to the CONV-RT. The CONV-RT, on the other hand, showed a higher relative abundance of n-alkanes, n-alkenes, aromatics, fatty acids, and N compounds compared to ORG-RT (Table 6). In terms of fertilisation, COMP-FS showed a higher relative abundance of n-alkanes, n-alkenes, aromatics and polyaromatics compared to MINE-FS. The MINE-FS, on the other hand, showed a higher relative abundance of benzofurans, carbohydrates, fatty acids, lignin phenols, N compounds, and phenols compared to the COMP-FS (Table 6). In relation of years of sampling, samples collected in 2018 showed a higher relative abundance of almost all groups expected for the benzofurans, phenols and polyaromatics than the samples collected in 2011 (Table 6).
For deeper soil layers (0.30–0.60 m), 72 quantified compounds were observed of the total 184 detected (Supplementary Table S6). Comparison between the treatments indicated that ORG-RT had a higher relative abundance of n-alkenes, aromatics, benzofurans, and polyaromatics compared to the CONV-RT. Consequently, the CONV-RT showed a higher relative abundance of carbohydrates, fatty acids, lignin phenols, N compounds and phenols. Concerning fertilisation, the COMP-FS showed a higher relative abundance of n-alkenes, benzofurans and phenols while the MINE-FS showed a higher relative abundance of aromatics, carbohydrates, fatty acids, lignin phenols, N compounds, and polyaromatics (Table 6). In relation of years of sampling, samples collected in 2018 showed a higher relative abundance of n-alkenes, aromatics, carbohydrates, phenols and polyaromatics while samples collected in 2011 showed a higher relative abundance of benzofurans, fatty acids, lignin phenols and N compound (Table 6).
Comparisons between the two depth intervals indicated an increased contribution from aromatics, benzofurans, carbohydrates, and polyaromatics at deeper soil layers (0.30–0.60 m), whereas n-alkenes, fatty acids, and lignin phenols decreased at topsoil layer (0–0.30 m), regardless of the RS, FS or YR (Table 6). It was also observed an increased contribution from N compounds at deeper soil layers (0.30–0.60 m) under CONV-RT, MINE-FS and in the samples collected in 2011 compared to the topsoil layer (0–0.30 m).
4 Discussion
4.1 Changes in soil C stocks due to differences in crop rotation, length of ley periods, and fertilisation sources
Organic rotation with the use of 3 years of temporary grass-clover leys (rather than 2 years, represented by the conventional rotation), and compost fertilisation led to SOC accumulation confirming our hypothesis. This also reflects findings from previous studies, which particularly compared conventional vs. organic systems as a whole (Gattinger et al., 2012; Triberti et al., 2016; Jian et al., 2020). However, while organic rotation showed higher SOC stocks than the conventional rotation under both sampled years (i.e. 2011 and 2018) and soil depth intervals (i.e. 0-0.30 m and 0.30–0.60 m) irrespective of the fertility sources (i.e., mineral or compost), compost fertilisation led to topsoil SOC accumulation (0–0.30 m) over the years (i.e., from 2011 to 2018) under both crop rotations. These results suggested that these two management practices are playing a strategic role in SOC accumulation.
The positive effect on SOC stocks by organic rotation may be partially ascribed to both the incorporation of legumes and the longer length of ley periods (3 years vs. 2 years under organic and conventional rotation, respectively). Previous research has indicated that the mixture of grasses and legumes (e.g., grass-clover) on ley periods can provide additional yield benefits and thus increase SOC stocks via higher crop residue deposition to the soil surface (Persson et al., 2008; O’Dea et al., 2013). This was also the case in our experiment (data not shown, but published in Bilsborrow et al. (2013)). Greater above-ground biomass can also lead to greater below-ground biomass along with more rhizo-deposition, and soil microbial activities (Araujo et al., 2012; Balakrishna et al., 2017), all of which can further benefit SOC accumulation even at deeper soil layers. According to a recent meta-analysis conducted by Jian et al. (2020), a greater mass and activity of root biomass, rhizo-deposits, and soil microbes could enhance the availability of essential nutrients to plant growth (e.g., N, phosphorus, and potassium), which can be a mechanism explaining the positive effect in SOC stocks at both soil depth intervals. The positive effect of both the incorporation of legumes and the longer length of ley periods on SOC stocks is also in line with previous research that suggested a minimum period of three-years ley after five-years arable rotation to promote a significant increase in SOC concentration in topsoil layers (Johnston et al., 2017).
In turn, the topsoil SOC accumulation (0–0.30 m) in both crop rotations over the years (2011–2018) under compost fertilisation can be attributed to the highest and direct supply of SOM to the soil (Aguilera et al., 2013). Previous research also reported significant SOC stock increase under fields receiving organic amendments such as composted dairy manure due to the direct supply of organic C (Christensen, 1988; Gerzabek et al., 2001; Gattinger et al., 2012). Another important factor that may have favoured SOC accumulation under compost fertilisation is its potential to enhance soil aggregate stability (Haynes and Naidu, 1998; Whalen and Chang, 2002). Organic amendments were previous shown to have positive effects on soil biological activity (Maeder et al., 2002; Lori et al., 2017), which can foster the physical protection of C against decomposition through chemical-physical bindings processes (Six et al., 2002).
Whilst the mixture of grasses and legumes and the use of organic amendments often result in an increased SOC stock (Sainju et al., 2006; Jian et al., 2020), mixed results have been reported due to the use of grass or legume during the ley period phases as well as due to the application of different organic amendments source (Mazzoncini et al., 2011; Aguilera et al., 2013; O’Dea et al., 2013). This might be due to differences in biomass production, C:N ratios and lignin content of the crops in the rotation as well as persistence of the organic amendments source to degradability in soils (Tokarski et al., 2019; Zhou et al., 2019). In the organic rotation, along with the grass-clover ley periods, other legumes (e.g., peas and beans) and vegetables (e.g., cabbage, lettuces, onions, and carrots) were cultivated in an 8-year period (2011–2018), which might have provided the finest balance between biomass production and optimal C:N ratio inputs for SOC accumulation benefits. While legumes (usually low C:N ratios) provide soil N to plants by fixing atmospheric N, the grass provides high biomass with high C:N ratios (Jian et al., 2020). In turn, organic amendments such as farmyard manure can increase SOC stocks as it is a C source that offers strong resistance to microbial decomposition (Nardi et al., 2004; Li et al., 2018). In this sense, the combination of grass-clover ley periods, other legumes and vegetables, and compost fertilisation is presumed to be the optimum for long-lasting SOC stock benefits. However, it is important to highlight that the amount of biomass and the characteristics of residues (i.e., C:N ratios, lignin content, as well as other molecular compounds) play a key role in SOM mineralisation (Tian et al., 1992; Triberti et al., 2016). Accordingly, crop choice in the rotation and organic amendment sources can either increase or decrease SOC stocks through effects not only related to residue deposition but also due to potential changes in soil properties, including chemical (nutrient availability), physical (soil structure), and biological (microbial biomass) properties (Campbell et al., 1991; Bandick and Dick, 1999; Sainju et al., 2006). Since this study evaluated SOC stocks in a relatively short period (only 8 years apart between sampling years), the results should be considered with caution as changes in SOC are expected to occur slowly and variations over the years are common regardless of the management practices deployed (Smith et al., 2020).
4.2 Effects of differences in crop rotation, length of ley periods, and fertilisation sources on SOM composition and soil C stabilisation
A unique combination of physical SOM fractionation, TG-DSC-QMS and Py-GC/MS-TMAH analyses allowed us to better understand potential changes in SOM composition and soil C stability brought about by differences in crop rotation, length of ley periods, and fertilisation sources strategies. The results of this study particularly suggested that the increased topsoil (0–0.30 m) SOC stocks under organic rotation with 3 years grass-clover ley period might be susceptible to losses since it occurs through a high contribution from fresh organic materials in the soil surface. On the other hand, the increased subsoil (0.30–0.60 m) SOC stocks under the same rotation have occurred through a higher contribution of more stable compounds, probably related to the set of crops grown, and thus different rooting patterns, implying a potential SOC stabilisation. Likewise, the increased topsoil SOC stocks over years under compost fertilisation showed a larger contribution from more stable compounds.
In this study, crop straw and debris have been always removed from the field under both crop rotations while organic amendments were applied mainly using composted dairy manure. In terms of organic rotation, this indicates that the increased SOC stock in both top- and deeper soil layers was a function of a more diverse rotation system, which is accompanied by a longer period under grass-clover leys (3 years vs 2 years in the conventional rotation). Previous studies have suggested that a more diverse rotation could supply higher C inputs from root biomass and crop stubble; materials acknowledged for their relevant amount of stable SOM (Triberti et al., 2016). In addition to the high C:N ratios of grasses (Jian et al., 2020), studies from Martens, (2000) and Lorenz et al. (2005) indicated that cereal roots and stubbles are slowly decomposed materials as they have high C:N ratios, lignin, and phenols contents. This could be a second mechanism to explain the enhanced SOC accumulation under the organic rotation, as legumes, grasses and cereal were all inserted over the rotation. On the other hand, as compost fertilisation such as farmyard manure per se offers a resistance option to biodegradability in soils (Nardi et al., 2004; Li et al., 2018), it might benefit SOC accumulation, irrespective of the crop rotation, due to the presence of more stabilised C forms. This was confirmed by a meta-analysis study conducted by Aguilera et al. (2013), where the authors found that raw organic amendment materials have a lower capability to increase soil C sequestration than organic composted materials. We also speculate that both organic rotation and compost fertilisation resulted in enhanced faunal activity, particularly worms, which promotes stability of organomineral aggregates and consequently SOC stabilisation (Coq et al., 2007).
Such assumptions were partially validated by our physical fractionation of the SOM, TG-DSC-QMS and Py-GC-MS analyses. Regarding crop rotation, thermal analysis (i.e., TG-DSC-QMS) showed that organic rotation has a slightly higher relative weight loss and ion intensity for CO2 (m/z 44) in the temperature intervals between 350°C–500°C and 500°C–750°C (Exo 2 and Exo 3) for both soil layers. Likewise, compost fertilisation also resulted in a slightly higher relative weight loss and ion intensity for CO2 (m/z 44) in the same temperature intervals at both soil layers. The results of the present study are also in agreement with a recent study conducted by Tokarski et al. (2019), who observed that farmyard manure results in thermal mass losses mainly around 450°C. Previous studies using thermogravimetry (TG) and differential scanning calorimetry (DSC) analysis indicate that exothermic peaks up to 350°C are related to decomposition of organic matter rich in labile aliphatic and carboxyl groups, whereas identified peaks up to approximately 500°C represent dominance of stable aromatic component classes. However, although these findings may indicate high amounts of recalcitrant and refractory C fractions and therefore a potential SOC stabilisation under both organic rotation and compost fertilisation (Lopez-Capel et al., 2005b; 2006; Manning et al., 2005; Plante et al., 2009), some considerations should be carefully taken into account.
Under organic rotation and for both soil depth intervals, there was a trend (non-significant) towards a decreased SOC in the mineral-associated fractions (silt and clay fraction from physical fractionation technique; SC < 53 μm), i.e., less accessible to decomposers and thus more stable and long-lived SOM (von Lützow et al., 2007). Although not statistically proven, this potential disparity between the physical fractionation of the SOM and the thermal analysis (TG-DSC-QMS) results might be due to either the similarity between the two rotations in terms of SOC associated with this fraction (also observed in the TG-DSC-QMS analysis) as well as potential discrepancies between the temperature intervals and soil fractions (Schiedung et al., 2017). At a molecular level, the organic rotation has shown a slightly higher relative abundance of benzofurans, carbohydrates, lignin-phenols, phenols, and polyaromatics in the top 0–0.30 m depth, in comparison to the conventional rotation. Conversely, in deeper soil layers (0.30–0.60 m), organic rotation showed a higher relative abundance of n-alkenes, aromatics, benzofurans, and polyaromatics as well as a much lower relative abundance of carbohydrates, fatty acids, lignin-phenols, N compounds, and phenols. Benzofurans, carbohydrates, lignin-phenols and phenols are products from relatively fresh plant materials while aromatics and polyaromatic compounds are products that originate from different sources, including lignin, carbohydrates proteins and charred plant material (González-Pérez et al., 2004; Kaal et al., 2008; Mazzetto et al., 2019). Pyrolysis products from cutan and suberin result in n-alkanes and n-alkenes compounds, which are more resistant against degradation than lignin (Tegelaar et al., 1995; Klotzbücher et al., 2011). These results indicate that the use of one of these techniques alone can lead to a misleading conclusion about soil C stability. In this study, in particular, the unique combination of techniques allows us to confidently say that while the organic rotation has increased SOC stocks in the topsoil layers, it might be susceptible to losses, as there is a high contribution from fresh organic materials. This is most likely related to the potential higher yields under such crop rotation and hence a higher amount of fresh crop residue deposition to the soil surface (Persson et al., 2008; O’Dea et al., 2013). On the other hand, increased SOC stocks in deeper soil layers under organic rotation may be attributed to other factors rather than crop residue deposition. In particular, it can be attributed to the fact that organic rotation is more diversified with a completely different set of crops and rooting patterns, including deep-rooting crops, compared to the conventional rotation (Blanco-Canqui et al., 2017). Kutsch et al. (2010), highlighted the importance of root biomass, rhizo-deposits, and microbes as sources of below-ground C. The high relative abundance of n-alkenes, aromatics, and polyaromatics in deeper soil layers (i.e., > 0.30 m) under organic rotation is an important finding as it implies that SOC stabilisation may be occurring (Mazzetto et al., 2019).
Concerning the fertility sources, a significant higher SOC in the heavy fraction (HF > 53 μm), i.e., a more labile fraction than the mineral-associated fraction due to its weaker association with clay mineral matrix (Hassink, 1997), was observed in the topsoil layers under the mineral fertilisation in comparison to compost fertilisation treatment. Also, at the same soil depth interval, a trend (also non-significant) towards increased SOC in the mineral-associated fractions (silt and clay fraction; SC < 53 μm) was observed under the compost fertilisation treatment in comparison to mineral fertilisation. In subsoil layers (0.30–0.60 m), mineral fertilisation significantly increased SOC in the mineral-associated fractions (silt and clay fraction; SC < 53 μm) over time, while compost fertilisation decreased it. These results suggest that the observed increased topsoil SOC stocks (0–0.30 m) over 8 years (2011–2018) under compost fertilisation can potentially lead to a SOC stabilisation, but this effect might be limited to depths less than 0.30 m. In contrast, mineral fertilisation might have a positive effect on SOC stabilisation in subsoil layers. The mechanisms for this could be the same as those discussed under organic rotation, i.e., higher yields followed by a higher amount of fresh crop residue deposits, which are potentially incorporated to the soil through tillage events (Bilsborrow et al., 2013; Schellekens et al., 2013) and greater below-ground biomass followed by greater rhizo-deposition, and soil microbial activities (Araujo et al., 2012; Balakrishna et al., 2017). The Py-GC-MS results reflected such assumptions. For the depth range 0–0.30 m, mineral fertilisation showed a higher relative abundance of products originated from fresh plant materials, including lignin-phenols and phenols (i.e., relatively easy to decompose), while compost fertilisation showed a higher relative abundance of compounds that are relatively difficult to decompose including aliphatic compounds (n-alkanes and n-alkenes), aromatics, and polyaromatics. For the 0.30–0.60 m depth interval, although the mineral fertilisation continued to show higher relative abundance of products originated from fresh plant materials, it also showed higher relative abundance of recalcitrant products in comparison to the compost fertilisation treatment (e.g., aromatics and polyaromatics).
Lastly, it is also worth noting a few further points: 1) there was a significant increase in the mineral-associated SOC fractions (SC < 53 μm) after a full rotation cycle, at topsoil layer (0–0.30 m) and irrespective of the crop rotation or fertility source. This is an important outcome as it indicates a potential stabilisation by the interaction of clay minerals and SOC. Previous studies have observed that the thermal behaviour of SOC stocks was affected by clay minerals interactions (Leinweber and Schulten, 1992; Plante et al., 2005). In particular, high clay content soils have a greater potential to stabilise SOC compared to sandy soils (Lützow et al., 2006; Schrumpf et al., 2013; Brandani et al., 2016). It is very unlikely, however, that clay content and soil mineralogy have changed over an 8-year crop rotation period, which ultimately suggests that both crop rotations and fertility sources are somehow stabilising SOC over-time at the 0–0.30 m depth; 2) although some disparities have been observed between physical fractionation of SOM and TG-DSC-QMS analysis, the results of both agreed with each other in relation to years of sampling (e.g., higher mineral-associated C fractions and higher mass losses and soil C released in 2018 in the Exo 2); and 3) at a molecular level, it was observed a substantial decrease in subsoil layers (0.30–0.60 m) was observed for n-alkenes, n-alkanes, fatty-acids, and lignin-phenols, whilst aromatics, benzofurans, carbohydrates, and polyaromatics increased for all treatments in topsoil layers (0–0.30 m). In addition, an increase contribution from N compounds was observed under conventional rotation, mineral fertilisation and samples collected in 2011. The decreases in n-alkenes, n-alkanes, fatty-acids, and lignin-phenols at depth as well as the high contribution from polyaromatics are acceptable findings as they are pyrolysis products from plant biopolymers/biological origin and black carbon, respectively (Ralph and Hatfield, 1991; Nierop et al., 2001; González-Pérez et al., 2014). However, the higher relative abundance of carbohydrates at this depth interval regardless of the treatment as well as the high contribution from N containing compounds under the conventional system practices (i.e., conventional rotation and mineral fertilisation) deserves particular attention. Upon pyrolysis, these are the main products of microbial activities (Derenne and Quéné, 2015) and thus it may suggest an enhanced SOM decomposition (Rumpel and Kögel-Knabner, 2010). Further research is still required to fully understand the impacts of management practices on SOM decomposition in subsoil layers (i.e., > 0.30 m depth).
5 Conclusion
This study has shown that SOC stocks, as well as soil organic matter (SOM) composition, differ between crop rotations that include 2- or 3-years grass-clover ley periods (i.e., conventional and organic crop rotations) and mineral and compost fertilisation sources with potential implications to C stabilisation. More specifically, a more diversified and legume-rich crop rotation system with 3 years grass-clover ley period (organic rotation) has shown higher SOC stocks than a more simplified rotation characterised by cereal intensive cropping and 2 years grass-clover ley period (conventional rotation). This result was observed in both the topsoil and subsoil (i.e. 0–0.30 m and 0.30–0.60 m) regardless of the sampled year (i.e. 2011 and 2018) and applied fertility sources (mineral or compost). In turn, compost fertilisation increased topsoil SOC stocks over years (i.e., from 2011 to 2018) under both crop rotations. The innovative combination of SOM physical fractionation, TG-DSC-QMS, and Py-GC/MS-TMAH analyses helped to better understand the potential shifts in the composition of SOM and consequently, draw more confident conclusions about its C stability. In particular, the findings of this study suggested that the increased topsoil SOC stocks under organic rotation and thus longer grass-clover ley period might be susceptible to losses since it occurs through a high contribution from fresh organic materials in the soil surface, easily lost in thermal analysis and not attached to more stabilised soil fractions (SC < 53 μm). On the other hand, the increased subsoil SOC stocks under the same rotation have occurred through a higher contribution of more stable compounds, only lost at high temperatures in thermal analysis and associated with more stable soil fractions (SC < 53 μm). Likewise, the increased topsoil SOC stocks over years under compost fertilisation showed a larger contribution from more stable compounds (aliphatics, aromatics and polyaromatics), only lost at high temperatures in thermal analysis and soil C associated with more stable soil fractions (SC < 53 μm). These findings ultimately suggest that combining these two management practices (i.e., organic rotation with longer ley periods and compost) could be one of the ways to assist agricultural systems in delivering potential stable soil C sequestration. Along with the positive effect to soil C accumulation, it is important to underscore that the use of the legumes and longer period of grass-clover leys in the rotation are widely acknowledged for their benefits on weed control, disease break crop as well as production. However, despite its potential critical role in agroecosystem functioning, crop rotations have been broadly simplified in modern agricultural systems, which may jeopardise the provision of ecosystem services. It is, therefore, encouraged to use of such practices regardless of the potential benefits to soil C. Further data collection from this, as well other, long-term trials will help to confirm these effects of crop rotation, ley periods, and fertility sources on SOC stocks and stabilisation and further elucidate their relationship with other factors for instance changes in environmental variables. The results, therefore, should be considered carefully under different climate, specific managements, soil texture and type than those tested here, as all these factors can either assist or hinder towards physical protection of SOM and thus affect decomposition and stabilisation of SOC stocks.
Data availability statement
The original contributions presented in the study are included in the article/Supplementary Material, raw data can also be provided on request from the corresponding author.
Author contributions
The data collection in this study, including soil analyses, consists of original work undertaken by the first author, CFZ. The 2018 soil sampling was carried out by the first author, CFZ while the 2011 soil sampling was carried out by farmer technicians as a part of a previous project. DACM and EL-C led the thermal analysis, and GDA led the pyrolysis-GC-MS analysis. Statistical analyses, interpretation of the data, and writing of the manuscript were led by CFZ, with full contributions from all co-authors.
Funding
This work was supported by the Faculty of Science, Agriculture and Engineering, Newcastle University [SAgE Scholarship]. DACM acknowledges support from NERC GGR NE/P019501/1.
Conflict of interest
The authors declare that the research was conducted in the absence of any commercial or financial relationships that could be construed as a potential conflict of interest.
Publisher’s note
All claims expressed in this article are solely those of the authors and do not necessarily represent those of their affiliated organizations, or those of the publisher, the editors and the reviewers. Any product that may be evaluated in this article, or claim that may be made by its manufacturer, is not guaranteed or endorsed by the publisher.
Supplementary material
The Supplementary Material for this article can be found online at: https://www.frontiersin.org/articles/10.3389/fenvs.2023.1113026/full#supplementary-material
References
Abbott, G. D., Swain, E. Y., Muhammad, A. B., Allton, K., Belyea, L. R., Laing, C. G., et al. (2013). Effect of water-table fluctuations on the degradation of Sphagnum phenols in surficial peats. Geochim. Cosmochim. Acta 106, 177–191. doi:10.1016/j.gca.2012.12.013
Aguilera, E., Lassaletta, L., Gattinger, A., and Gimeno, B. S. (2013). Managing soil carbon for climate change mitigation and adaptation in mediterranean cropping systems: A meta-analysis. Agric. Ecosyst. Environ. 168, 25–36. doi:10.1016/j.agee.2013.02.003
Araujo, A. S. F., Leite, L. F. C., De Freitas Iwata, B., De Andrade Lira, M., Xavier, G. R., and Do Vale Barreto Figueiredo, M. (2012). Microbiological process in agroforestry systems. A review. Agron. Sustain. Dev. 32, 215–226. doi:10.1007/s13593-011-0026-0
Arenillas, A., Rubiera, F., and Pis, J. J. (1999). Simultaneous thermogravimetric-mass spectrometric study on the pyrolysis behaviour of different rank coals. J. Anal. Appl. Pyrolysis. 50, 31–46. doi:10.1016/S0165-2370(99)00024-8
Bai, Y., and Cotrufo, M. F. (2022). Grassland soil carbon sequestration: Current understanding, challenges, and solutions. Science 377, 603–608. doi:10.1126/science.abo2380
Balakrishna, A. N., Lakshmipathy, R., Bagyaraj, D. J., and Ashwin, R. (2017). Influence of alley copping system on AM fungi, microbial biomass C and yield of finger millet, peanut and pigeon pea. Agrofor. Syst. 91, 487–493. doi:10.1007/s10457-016-9949-4
Bandick, A. K., and Dick, R. P. (1999). Field management effects on soil enzyme activities. Soil Biol. biochem. 31, 1471–1479. doi:10.1016/S0038-0717(99)00051-6
Basile-Doelsch, I., Balesdent, J., and Pellerin, S. (2020). Reviews and syntheses: The mechanisms underlying carbon storage in soil. Biogeosciences Discuss 17, 5223–5242. doi:10.5194/bg-2020-49
Bilsborrow, P., Cooper, J., Tétard-Jones, C., Średnicka-Tober, D., Barański, M., Eyre, M., et al. (2013). The effect of organic and conventional management on the yield and quality of wheat grown in a long-term field trial. Eur. J. Agron. 51, 71–80. doi:10.1016/j.eja.2013.06.003
Blake, G. H., and Hartge, K. H. (1986). “Bulk density,” in Methods of soil analysis Editor A. Klute 2nd ed. (Wisconsin: The American Society of Agronomy).
Blanco-Canqui, H., Francis, C. A., and Galusha, T. D. (2017). Does organic farming accumulate carbon in deeper soil profiles in the long term? Geoderma 288, 213–221. doi:10.1016/J.GEODERMA.2016.10.031
Brandani, C. B., Abbruzzini, T. F., Conant, R. T., and Cerri, C. E. P. (2016). Soil organic and organomineral fractions as indicators of the effects of land management in conventional and organic sugar cane systems. Soil Res. 55, 145. doi:10.1071/sr15322
Buurman, P., and Roscoe, R. (2011). Different chemical composition of free light, occluded light and extractable SOM fractions in soils of cerrado and tilled and untilled fields, minas gerais, Brazil: A pyrolysis-GC/MS study. Eur. J. Soil Sci. 62, 253–266. doi:10.1111/j.1365-2389.2010.01327.x
Cambardella, C. A., and Elliott, E. T. (1992). Particulate soil organic-matter changes across a grassland cultivation sequence. Soil Sci. Soc. Am. J. 56, 777–783. doi:10.2136/sssaj1992.03615995005600030017x
Campbell, C. A., Biederbeck, V. O., Zentner, R. P., and Lafond, G. P. (1991). Effect of crop rotations and cultural practices on soil organic matter, microbial biomass and respiration in a thin Black Chernozem. Can. J. Soil Sci. 71, 363–376. doi:10.4141/cjss91-035
Challinor, J. M. (1989). A pyrolysis-derivatisation-gas chromatography technique for the structural elucidation of some synthetic polymers. J. Anal. Appl. Pyrolysis. 16, 323–333. doi:10.1016/0165-2370(89)80015-4
Chen, J., Lærke, P. E., and Jørgensen, U. (2022). Land conversion from annual to perennial crops: A win-win strategy for biomass yield and soil organic carbon and total nitrogen sequestration. Agric. Ecosyst. Environ. 330, 107907. doi:10.1016/j.agee.2022.107907
Christensen, B. T. (1985). Carbon and nitrogen in particle size fractions isolated from Danish arable soils by ultrasonic dispersion and gravity-sedimentation. Acta Agric. Scand. 35, 175–187. doi:10.1080/00015128509435773
Christensen, B. T. (1988). Effects of animal manure and mineral fertilizer on the total carbon and nitrogen contents of soil size fractions. Biol. Fertil. Soils. 5 , 304–307. doi:10.1007/BF00262136
Christensen, B. T. (1992). Physical fractionation of soil and organic matter in primary particle size and density separates. Adv. Soil Sci. 20, 1–90. doi:10.1007/978-1-4612-2930-8_1
Christensen, B. T. (2001). Physical fractionation of soil and structural and functional complexity in organic matter turnover. Eur. J. Soil Sci. 52, 345–353. doi:10.1046/j.1365-2389.2001.00417.x
Cooper, J. M., Butler, G., and Leifert, C. (2011a). Life cycle analysis of greenhouse gas emissions from organic and conventional food production systems, with and without bio-energy options. NJAS - Wagening. J. Life Sci. 58, 185–192. doi:10.1016/j.njas.2011.05.002
Cooper, J., Sanderson, R., Cakmak, I., Ozturk, L., Shotton, P., Carmichael, A., et al. (2011b). Effect of organic and conventional crop rotation, fertilization, and crop protection practices on metal contents in wheat (triticum aestivum). J. Agric. Food Chem. 59, 4715–4724. doi:10.1021/jf104389m
Coq, S., Barthès, B. G., Oliver, R., Rabary, B., and Blanchart, E. (2007). Earthworm activity affects soil aggregation and organic matter dynamics according to the quality and localization of crop residues-An experimental study (Madagascar). Soil Biol. biochem. 39, 2119–2128. doi:10.1016/j.soilbio.2007.03.019
Dell’Abate, M. T., Benedetti, A., and Sequi, P. (2000). Thermal methods of organic matter maturation monitoring during a composting process. J. Therm. Anal. Calorim. 61, 389–396. doi:10.1023/A:1010157115211
Dell’Abate, M. T., Benedetti, A., Trinchera, A., and Dazzi, C. (2002). Humic substances along the profile of two Typic Haploxerert. Geoderma 107, 281–296. doi:10.1016/S0016-7061(01)00153-7
Derenne, S., and Quéné, K. (2015). Analytical pyrolysis as a tool to probe soil organic matter. J. Anal. Appl. Pyrolysis. 111, 108–120. doi:10.1016/j.jaap.2014.12.001
Dixon, R. K., Brown, S., Houghton, R. A., Solomon, A. M., Trexler, M. C., and Wisniewski, J. (1994). Carbon pools and flux of global forest ecosystems. Science 263, 185–190. doi:10.1126/science.263.5144.185
Eyre, M. D., Critchley, C. N. R., Leifert, C., and Wilcockson, S. J. (2011). Crop sequence, crop protection and fertility management effects on weed cover in an organic/conventional farm management trial. Eur. J. Agron. 34, 153–162. doi:10.1016/j.eja.2011.01.001
Eyre, M. D., Luff, M. L., Atlihan, R., and Leifert, C. (2012). Ground beetle species (Carabidae, Coleoptera) activity and richness in relation to crop type, fertility management and crop protection in a farm management comparison trial. Ann. Appl. Biol. 161, 169–179. doi:10.1111/j.1744-7348.2012.00562.x
Farewell, T. S., Truckell, I. G., Keay, C. A., and Hallett, S. H. (2011). The derivation and application of soilscapes: Soil and environmental datasets from the national soil resources institute. England: Cranfield University.
Feller, C., and Beare, M. H. (1997). Physical control of soil organic matter dynamics in the tropics. Geoderma 79, 69–116. doi:10.1016/s0016-7061(97)00039-6
Fernández, J. M., Peltre, C., Craine, J. M., and Plante, A. F. (2012). Improved characterization of soil organic matter by thermal analysis using CO2/H2O evolved gas analysis. Environ. Sci. Technol. 46, 8921–8927. doi:10.1021/es301375d
Gattinger, A., Muller, A., Haeni, M., Skinner, C., Fliessbach, A., Buchmann, N., et al. (2012). Enhanced top soil carbon stocks under organic farming. Proc. Natl. Acad. Sci. 109, 18226–18231. doi:10.1073/pnas.1209429109
Gerzabek, M. H., Haberhauer, G., and Kirchmann, H. (2001). Soil organic matter pools and carbon-13 natural abundances in particle-size fractions of a long-term agricultural field experiment receiving organic amendments. Soil Sci. Soc. Am. J. 65, 352–358. doi:10.2136/sssaj2001.652352x
González-Pérez, J. A., Almendros, G., De La Rosa, J. M., and González-Vila, F. J. (2014). Appraisal of polycyclic aromatic hydrocarbons (PAHs) in environmental matrices by analytical pyrolysis (Py-GC/MS). J. Anal. Appl. Pyrolysis 109, 1–8. doi:10.1016/j.jaap.2014.07.005
González-Pérez, J. A., González-Vila, F. J., Almendros, G., and Knicker, H. (2004). The effect of fire on soil organic matter - a review. Environ. Int. 30, 855–870. doi:10.1016/j.envint.2004.02.003
Guest, E. J., Palfreeman, L. J., Holden, J., Chapman, P. J., Firbank, L. G., Lappage, M. G., et al. (2022). Soil macroaggregation drives sequestration of organic carbon and nitrogen with three-year grass-clover leys in arable rotations. Sci. Total Environ. 852, 158358. doi:10.1016/j.scitotenv.2022.158358
Hassink, J. (1997). The capacity of soils to preserve organic C and N by their association with clay and silt particles. Plant Soil 191, 77–87. doi:10.1023/A:1004213929699
Haynes, R. J., and Naidu, R. (1998). Influence of lime, fertilizer and manure applications on soil organic matter content and soil physical conditions: A review. Nutr. Cycl. Agroecosyst. 51, 123–137. doi:10.1023/A:1009738307837
Hothorn, T., Bretz, F., and Westfall, P. (2008). Simultaneous inference in general parametric models. Biom. J. 50, 346–363. doi:10.1002/bimj.200810425
Jian, J., Du, X., Reiter, M. S., and Stewart, R. D. (2020). A meta-analysis of global cropland soil carbon changes due to cover cropping. Soil Biol. biochem. 143, 107735. doi:10.1016/j.soilbio.2020.107735
Johnston, A. E., Poulton, P. R., Coleman, K., Macdonald, A. J., and White, R. P. (2017). Changes in soil organic matter over 70 years in continuous arable and ley-arable rotations on a sandy loam soil in England. Eur. J. Soil Sci. 68, 305–316. doi:10.1111/ejss.12415
Kaal, E., and Janssen, H. G. (2008). Extending the molecular application range of gas chromatography. J. Chromatogr. A 1184, 43–60. doi:10.1016/j.chroma.2007.11.114
Kaal, J., Martínez-Cortizas, A., Nierop, K. G. J., and Buurman, P. (2008). A detailed pyrolysis-GC/MS analysis of a black carbon-rich acidic colluvial soil (Atlantic ranker) from NW Spain. Appl. Geochem. 23, 2395–2405. doi:10.1016/j.apgeochem.2008.02.026
Klotzbücher, T., Kaiser, K., Guggenberger, G., Gatzek, C., and Kalbitz, K. (2011). A new conceptual model for the fate of lignin in decomposing plant litter. Ecology 92, 1052–1062. doi:10.1890/10-1307.1
Kutsch, W. L., Bahn, M., and Heinemeyer, A. (2010). Soil carbon dynamics: An integrated methodology. Cambridge: Cambridge University Press.
Lal, R., Negassa, W., and Lorenz, K. (2015). Carbon sequestration in soil. Curr. Opin. Environ. Sustain. 15, 79–86. doi:10.1016/j.cosust.2015.09.002
Lal, R. (2004). Soil carbon sequestration impacts on global climate change and food security. Science 304, 1623–1627. doi:10.1126/science.1097396
Langier-Kuźniarowa, A. (2002). “Thermal analysis of organo-clay complexes,” in Organo-clay complexes and interactions. Editors S. Yariv, and H. Cross (New York: Marcel Dekker).
Lavallee, J. M., Soong, J. L., and Cotrufo, M. F. (2019). Conceptualizing soil organic matter into particulate and mineral-associated forms to address global change in the 21st century. Glob. Chang. Biol. 26, 261–273. doi:10.1111/gcb.14859
Lehmann, J., and Kleber, M. (2015). The contentious nature of soil organic matter. Nature 528, 60–68. doi:10.1038/nature16069
Leinweber, P., and Schulten, H.-R. (1993). Dynamics of soil organic matter studied by pyrolysis—Field ionization mass spectrometry. J. Anal. Appl. Pyrolysis 25, 123–136. doi:10.1016/0165-2370(93)80036-Y
Leinweber, P., and Schulten, H. R. (1992). Differential thermal analysis, thermogravimetry and in-source pyrolysis-mass spectrometry studies on the formation of soil organic matter. Thermochim. Acta. 200, 151–167. doi:10.1016/0040-6031(92)85112-9
Li, J., Wen, Y., Li, X., Li, Y., Yang, X., Lin, Z., et al. (2018). Soil labile organic carbon fractions and soil organic carbon stocks as affected by long-term organic and mineral fertilization regimes in the North China Plain. Soil Tillage Res. 175, 281–290. doi:10.1016/j.still.2017.08.008
Lopez-Capel, E., Abbott, G. D., Thomas, K. M., and Manning, D. A. C. (2006). Coupling of thermal analysis with quadrupole mass spectrometry and isotope ratio mass spectrometry for simultaneous determination of evolved gases and their carbon isotopic composition. J. Anal. Appl. Pyrolysis 75, 82–89. doi:10.1016/j.jaap.2005.04.004
Lopez-Capel, E., Bol, R., and Manning, D. A. (2005a). Application of simultaneous thermal analysis mass spectrometry and stable carbon isotope analysis in a carbon sequestration study. Rapid Commun. Mass Spectrom. 19, 3192–3198. doi:10.1002/rcm.2145
Lopez-Capel, E., Sohi, S. P., Gaunt, J. L., and Manning, D. A. C. (2005b). Use of thermogravimetry-differential scanning calorimetry to characterize modelable soil organic matter fractions. Soil Sci. Soc. Am. J. 69, 930–140. doi:10.2136/sssaj2005.0930
Lorenz, K., and Lal, R. B. T. (2005). The depth distribution of soil organic carbon in relation to land use and management and the potential of carbon sequestration in subsoil horizons. Cambridge: Academic Press.
Lori, M., Symnaczik, S., Mäder, P., De Deyn, G., and Gattinger, A. (2017). Organic farming enhances soil microbial abundance and activity—a meta-analysis and meta-regression. PLoS One 12, e0180442. doi:10.1371/journal.pone.0180442
Lützow, M. V., Kögel-Knabner, I., Ekschmitt, K., Matzner, E., Guggenberger, G., Marschner, B., et al. (2006). Stabilization of organic matter in temperate soils: Mechanisms and their relevance under different soil conditions - a review. Eur. J. Soil Sci. 57, 426–445. doi:10.1111/j.1365-2389.2006.00809.x
Maeder, P., Fliessbach, A., Dubois, D., Gunst, L., Fried, P., and Niggli, U. (2002). Soil fertility and biodiversity in organic farming. Science 296, 1694–1697. doi:10.1126/science.1071148
Manning, D. A. C., Lopez-Capel, E., and Barker, S. (2005). Seeing soil carbon: Use of thermal analysis in the characterization of soil C reservoirs of differing stability. Mineral. Mag. 69, 425–435. doi:10.1180/0026461056940260
Martens, D. A. (2000). Plant residue biochemistry regulates soil carbon cycling and carbon sequestration. Soil Biol. biochem. 32, 361–369. doi:10.1016/S0038-0717(99)00162-5
Mason, S. L., Filley, T. R., and Abbott, G. D. (2012). A comparative study of the molecular composition of a grassland soil with adjacent unforested and afforested moorland ecosystems. Org. Geochem. 42, 1519–1528. doi:10.1016/j.orggeochem.2010.11.003
Mazzetto, J. M. L., Melo, V. F., Bonfleur, E. J., Vidal-Torrado, P., and Dieckow, J. (2019). Potential of soil organic matter molecular chemistry determined by pyrolysis-gas chromatography/mass spectrometry for forensic investigations. Sci. Justice 59, 635–642. doi:10.1016/j.scijus.2019.07.003
Mazzoncini, M., Sapkota, T. B., Bàrberi, P., Antichi, D., and Risaliti, R. (2011). Long-term effect of tillage, nitrogen fertilization and cover crops on soil organic carbon and total nitrogen content. Soil Tillage Res. 114, 165–174. doi:10.1016/j.still.2011.05.001
Mc Lean, E. O. (1982). “Soil pH and lime requirement,” in Methods of soil analysis, Part 2: Chemical and microbiological properties (Madison: ACSESS).
McKenzie, A. J., Vickery, J. A., Leifert, C., Shotton, P., and Whittingham, M. J. (2011). Disentangling the effects of fertilisers and pesticides on winter stubble use by farmland birds. Basic Appl. Ecol. 12, 80–88. doi:10.1016/j.baae.2010.10.007
Meier, D., and Faix, O. (1992). “Pyrolysis-gas chromatography-mass spectrometry BT - methods in lignin chemistry,” in, eds. S. Y. Lin, and C. W. Dence (Berlin, Heidelberg: Springer Berlin Heidelberg).
Mückenhausen, E. (1981). Avery, B.W.: Soil classification for England and wales (higher categories). Soil survey, technical monograph No. 14, rothamsted experimental station, harpenden/england 1980. Preis£. Z. für Pflanzenernährung Bodenkd. 144, 228. doi:10.1002/jpln.19811440221
Nardi, S., Morari, F., Berti, A., Tosoni, M., and Giardini, L. (2004). Soil organic matter properties after 40 years of different use of organic and mineral fertilisers. Eur. J. Agron. 21, 357–367. doi:10.1016/j.eja.2003.10.006
Nelson, D. W., and Sommers, L. E. (1996). “Total carbon, organic carbon, and organic matter,” in Methods of soil analysis. Part 2. 2. SSSA Book Series, 5. Editors A. L. Page, R. H. Miller, and D. R. Keeney (Madison: ASA).
Nierop, K. G. J., Pulleman, M. M., and Marinissen, J. C. Y. (2001). Management induced organic matter differentiation in grassland and arable soil: A study using pyrolysis techniques. Soil Biol. biochem. 33, 755–764. doi:10.1016/S0038-0717(00)00223-6
O’Dea, J. K., Miller, P. R., and Jones, C. A. (2013). Greening summer fallow with legume green manures: On-farm assessment in north-central Montana. J. Soil Water Conserv. 68, 270–282. doi:10.2489/jswc.68.4.270
Oliveira, D. M. da S., Schellekens, J., and Cerri, C. E. P. (2016). Molecular characterization of soil organic matter from native vegetation-pasture-sugarcane transitions in Brazil. Sci. Total Environ. 548-549, 450–462. doi:10.1016/j.scitotenv.2016.01.039
Paradis, E., Claude, J., and Strimmer, K. (2004). Ape: Analyses of phylogenetics and evolution in R language. Bioinformatics 20, 289–290. R package version 5.0. doi:10.1093/bioinformatics/btg412
Paustian, K., Lehmann, J., Ogle, S., Reay, D., Robertson, G. P., and Smith, P. (2016). Climate-smart soils. Nature 532, 49–57. doi:10.1038/nature17174
Persson, T., Bergkvist, G., and Kätterer, T. (2008). Long-term effects of crop rotations with and without perennial leys on soil carbon stocks and grain yields of winter wheat. Nutr. Cycl. Agroecosyst. 81, 193–202. doi:10.1007/s10705-007-9144-0
Pinheiro, J., Bates, D., DebRoy, S., Sarkar, D., and Team, R. C. (2018). nlme: Linear and nonlinear mixed effects models. R. package version 3, 1–10. doi:10.1016/j.forsciint.2018.06.019
Plante, A. F., Fernández, J. M., and Leifeld, J. (2009). Application of thermal analysis techniques in soil science. Geoderma 153, 1–10. doi:10.1016/j.geoderma.2009.08.016
Plante, A. F., Pernes, M., and Chenu, C. (2005). Changes in clay-associated organic matter quality in a C depletion sequence as measured by differential thermal analyses. Geoderma 129, 186–199. doi:10.1016/j.geoderma.2004.12.043
Poeplau, C., Don, A., Six, J., Kaiser, M., Benbi, D., Chenu, C., et al. (2018). Isolating organic carbon fractions with varying turnover rates in temperate agricultural soils – a comprehensive method comparison. Soil Biol. biochem. 125, 10–26. doi:10.1016/J.SOILBIO.2018.06.025
Powlson, D. S., Glendining, M. J., Coleman, K., and Whitmore, A. P. (2011). Implications for soil properties of removing cereal straw: Results from long-term studies. Agron. J. 103, 279–287. doi:10.2134/agronj2010.0146s
Ralph, J., and Hatfield, R. D. (1991). Pyrolysis-GC-MS characterization of forage materials. J. Agric. Food Chem. 39, 1426–1437. doi:10.1021/jf00008a014
Rumpel, C., and Kögel-Knabner, I. (2010). Deep soil organic matter—A key but poorly understood component of terrestrial C cycle. Plant Soil 338, 143–158. doi:10.1007/s11104-010-0391-5
Sainju, U. M., Singh, B. P., Whitehead, W. F., and Wang, S. (2006). Carbon supply and storage in tilled and nontilled soils as influenced by cover crops and nitrogen fertilization. J. Environ. Qual. 35, 1507–1517. doi:10.2134/jeq2005.0189
Schellekens, J., Barberá, G. G., Buurman, P., Pérez-Jordà, G., and Martínez-Cortizas, A. (2013). Soil organic matter dynamics in Mediterranean A-horizons - the use of analytical pyrolysis to ascertain land-use history. J. Anal. Appl. Pyrolysis. 104, 287–298. doi:10.1016/j.jaap.2013.07.004
Schiedung, M., Don, A., Wordell-Dietrich, P., Alcántara, V., Kuner, P., and Guggenberger, G. (2017). Thermal oxidation does not fractionate soil organic carbon with differing biological stabilities. J. Plant Nutr. Soil Sci. 180, 18–26. doi:10.1002/jpln.201600172
Schmidt, M. W., Torn, M. S., Abiven, S., Dittmar, T., Guggenberger, G., Janssens, I. A., et al. (2011). Persistence of soil organic matter as an ecosystem property. Nature 478, 49–56. doi:10.1038/nature10386
Schrumpf, M., Kaiser, K., Guggenberger, G., Persson, T., Kögel-Knabner, I., and Schulze, E. D. (2013). Storage and stability of organic carbon in soils as related to depth, occlusion within aggregates, and attachment to minerals. Biogeosciences 10, 1675–1691. doi:10.5194/bg-10-1675-2013
Six, J., Feller, C., Denef, K., Ogle, S. M., de Moraes, J. C., and Albrecht, A. (2002). Soil organic matter, biota and aggregation in temperate and tropical soils - effects of no-tillage. Agronomie 22, 755–775. doi:10.1051/agro:2002043
Smith, P., Soussana, J- F., and Angers, D. (2020). How to measure, report and verify soil carbon change to realize the potential of soil carbon sequestration for atmospheric greenhouse gas removal. Glob Change Biol. 26, 219–241. doi:10.1111/gcb.14815
Soil Association (2019). Soil association standards farming and growing. United Kingdom: Soil Association.
Team, R. D. C. (2019). R: A language and environment to statistical computing. Austria: R Foundation for Statistical Computing.
Tegelaar, E. W., Hollman, G., Van Der Vegt, P., De Leeuw, J. W., and Holloway, P. J. (1995). Chemical characterization of the periderm tissue of some angiosperm species: Recognition of an insoluble, non-hydrolyzable, aliphatic biomacromolecule (suberan). Org. Geochem. 23, 239–251. doi:10.1016/0146-6380(94)00123-I
Tian, G., Kang, B. T., and Brussaard, L. (1992). Biological effects of plant residues with contrasting chemical compositions under humid tropical conditions-Decomposition and nutrient release. Soil Biol. biochem. 24, 1051–1060. doi:10.1016/0038-0717(92)90035-V
Tokarski, D., Šimečková, J., Kučerík, J., Kalbitz, K., Demyan, M. S., Merbach, I., et al. (2019). Detectability of degradable organic matter in agricultural soils by thermogravimetry. J. Plant Nutr. Soil Sci. 182, 729–740. doi:10.1002/jpln.201800516
Triberti, L., Nastri, A., and Baldoni, G. (2016). Long-term effects of crop rotation, manure and mineral fertilisation on carbon sequestration and soil fertility. Eur. J. Agron. 74, 47–55. doi:10.1016/j.eja.2015.11.024
Trumbore, S. E. (1997). Potential responses of soil organic carbon to global environmental change. Proc. Natl. Acad. Sci. U. S. A. 94, 8284–8291. doi:10.1073/pnas.94.16.8284
Tuomisto, H. L., Hodge, I. D., Riordan, P., and Macdonald, D. W. (2012). Does organic farming reduce environmental impacts?-a meta-analysis of European research. J. Env. Manag. 112, 309–320. doi:10.1016/j.jenvman.2012.08.018
von Lützow, M., Kögel-Knabner, I., Ekschmitt, K., Flessa, H., Guggenberger, G., Matzner, E., et al. (2007). SOM fractionation methods: Relevance to functional pools and to stabilization mechanisms. Soil Biol. biochem. 39, 2183–2207. doi:10.1016/j.soilbio.2007.03.007
Wang, J., Werner, D., and Manning, D. A. C. (2022). A framework for integrating the terrestrial carbon stock of estates in institutional carbon management plans. Soil Use Manag. 38, 1172–1188. doi:10.1111/sum.12776
Wendt, J. W., and Hauser, S. (2013). An equivalent soil mass procedure for monitoring soil organic carbon in multiple soil layers. Eur. J. Soil Sci. 64, 58–65. doi:10.1111/ejss.12002
Whalen, J. K., and Chang, C. (2002). Macroaggregate characteristics in cultivated soils after 25 annual manure applications. Soil Sci. Soc. Am. J. 66, 1637–1647. doi:10.2136/sssaj2002.1637
Wickham, H. (2011). The split-apply-combine strategy for data analysis. J. Stat. Softw. 40 (1), 1–29. doi:10.18637/jss.v040.i01
WRB (2015). “World Reference Base for Soil Resources 2014, update 2015 International soil classification system for naming soils and creating legends for soil maps,” in World soil resources reports No. 106 (Rome: FAO).
Zani, C. F., Gowing, J., Abbott, G. D., Taylor, J. A., Lopez-Capel, E., and Cooper, J. (2021). Grazed temporary grass-clover leys in crop rotations can have a positive impact on soil quality under both conventional and organic agricultural systems. Eur. J. Soil Sci. 72, 1513–1529. doi:10.1111/ejss.13002
Zani, C. F., Lopez-Capel, E., Abbott, G. D., Taylor, J. A., and Cooper, J. M. (2022). Effects of integrating grass-clover leys with livestock into arable crop rotations on soil carbon stocks and particulate and mineral-associated soil organic matter fractions in conventional and organic systems. Soil Use Manag. 38, 448–465. doi:10.1111/sum.12754
Zhao, J., Chen, J., Beillouin, D., Lambers, H., Yang, Y., Smith, P., et al. (2022). Global systematic review with meta-analysis reveals yield advantage of legume-based rotations and its drivers. Nat. Commun. 13, 4926. doi:10.1038/s41467-022-32464-0
Zhou, G., Xu, S., Ciais, P., Manzoni, S., Fang, J., Yu, G., et al. (2019). Climate and litter C/N ratio constrain soil organic carbon accumulation. Natl. Sci. Rev. 6, 746–757. doi:10.1093/nsr/nwz045
Zhu, X., Chen, J., Huang, S., Li, W., Penuelas, J., Chen, J., et al. (2022). Manure amendment can reduce rice yield loss under extreme temperatures. Commun. Earth Environ. 3, 147. doi:10.1038/s43247-022-00481-y
Keywords: carbon persistence, carbon stocks, crop rotation, fertilisation sources, interactions, management practices
Citation: Zani CF, Manning DAC, Abbott GD, Taylor JA, Cooper J and Lopez-Capel E (2023) Diversified crop rotations and organic amendments as strategies for increasing soil carbon storage and stabilisation in UK arable systems. Front. Environ. Sci. 11:1113026. doi: 10.3389/fenvs.2023.1113026
Received: 30 November 2022; Accepted: 04 April 2023;
Published: 17 April 2023.
Edited by:
Daniela Businelli, University of Perugia, ItalyReviewed by:
Ji Chen, Aarhus University, DenmarkJoao Paulo Gonsiorkiewicz Rigon, São Paulo State University, Brazil
Copyright © 2023 Zani, Manning, Abbott, Taylor, Cooper and Lopez-Capel. This is an open-access article distributed under the terms of the Creative Commons Attribution License (CC BY). The use, distribution or reproduction in other forums is permitted, provided the original author(s) and the copyright owner(s) are credited and that the original publication in this journal is cited, in accordance with accepted academic practice. No use, distribution or reproduction is permitted which does not comply with these terms.
*Correspondence: Caio F. Zani, caiofzani@gmail.com